DOI:
10.1039/D3RA00866E
(Review Article)
RSC Adv., 2023,
13, 16488-16511
Stimuli-responsive nanocarrier delivery systems for Pt-based antitumor complexes: a review
Received
9th February 2023
, Accepted 30th March 2023
First published on 1st June 2023
Abstract
Platinum-based anticancer drugs play a crucial role in the clinical treatment of various cancers. However, the application of platinum-based drugs is heavily restricted by their severe toxicity and drug resistance/cross resistance. Various drug delivery systems have been developed to overcome these limitations of platinum-based chemotherapy. Stimuli-responsive nanocarrier drug delivery systems as one of the most promising strategies attract more attention. And huge progress in stimuli-responsive nanocarrier delivery systems of platinum-based drugs has been made. In these systems, a variety of triggers including endogenous and extracorporeal stimuli have been employed. Endogenous stimuli mainly include pH-, thermo-, enzyme- and redox-responsive nanocarriers. Extracorporeal stimuli include light-, magnetic field- and ultrasound responsive nanocarriers. In this review, we present the recent advances in stimuli-responsive drug delivery systems with different nanocarriers for improving the efficacy and reducing the side effects of platinum-based anticancer drugs.
1 Introduction
Since cisplatin, cis-diamminedichloroplatinum(II), has been used in cancer treatment with the FDA approval in 1978, it has been widely used to treat a variety of solid tumors, such as ovarian cancer, head and neck squamous cell carcinoma, non-small cell lung cancer, etc. However, the efficacy of cisplatin (CDDP) is heavily limited due to inherent or acquired drug resistance and systemic toxicity. In order to improve the limitations of cisplatin, numerous efforts have been made and thousands of platinum complexes have been synthesized. At present, carboplatin, oxaliplatin, heptaplatin, and other platinum drugs have been put into clinical use.1 Over the past fifty years, platinum-based (Pt-based) drugs for treating cancer have been used in the chemotherapy of more than 50% malignant tumors as a single agent or in combination with other agents. However, all these Pt-based drugs are modified based on the basic structure of cisplatin. While retaining the efficacy of cisplatin, they also retain some side effects of cisplatin to the same degree.2
Although Pt-based drugs have shown good potential in the treatment of many tumors, their systemic toxicity and drug resistance cannot be ignored. Various strategies have been developed for the design of new platinum drugs, and the drug delivery system based on nanoparticles has been applied in clinical practice as one of the most important options.3 For example, great efforts have been made to successfully develop selective nanocarriers that deliver drugs and genes to diseased sites.4,5 In the past decade, nano drug carriers, including polymer conjugates, liposomes, micelles, and inorganic and organic nanoparticles, have made significant contributions to the treatment of cancer.6 They can inhibit drug resistance, improve drug solubility, extend the half-life of drugs, provide a means to sustain and stimulate the release of reactive drugs, target drugs to tumors, and simultaneously deliver two or more drugs.7–9 Moreover, targeting drug delivery systems with nanocarriers have undergone a rapid development. Several targeting nanocarrier–drug conjugates have been applied in clinics with the approval of the FDA.10 However, targeting drug delivery systems with nanocarriers still remain questionable. Therefore, more efficient strategies need to be explored. The concept of stimulatory reactive drug delivery systems was first put forward in the late 1970s and then attracted extensive attention of researchers, especially on nanocarriers.
Stimulating reactive drug delivery systems is an important constituent of the drug delivery strategy. It can control drug release by generating specific responses to tiny exterior changes or stimuli in the physiological environment. To take advantage of specific micro environmental differences between tumor cells/tissues and normal cells/tissues, endogenous stimuli-responsive drug delivery systems including pH-, enzyme-, and redox-sensitive drug delivery attracted more attention.11 In order to maximize the therapeutic effect of Pt-based anti-cancer drugs and reduce side effects, functionalized nanocarriers have emerged as robust vehicle in the targeted delivery of Pt-based drugs, which showed better controlled-release profile and higher drug concentrations at the tumor tissue. These intriguing drug delivery systems are inert in the normal environment of the body but can be activated under weak acidity values, thermal stimuli, special enzymes and other changes of the microenvironment in vivo.12 Apart from endogenous stimuli, extracorporeal physical stimuli, including light, temperature, magnetic field and ultrasound, can be also applied in the targeted delivery and controlled release of Pt-based agents.13 In this review, we focus on the recent development of stimuli-responsive nanocarrier delivery systems of Pt-based drugs.
2 Endogenous stimuli-responsive drug delivery
Endogenous stimulations refer to stimulation from different environments between normal cells/tissues and tumor cells/tissues in vivo, including low pH, high redox potential and high enzyme level between cells (intracellular).
2.1 pH responsive drug delivery
So as to satisfy the high energy demands to maintain rapid cell divisions, cancer cells in the glycolytic metabolic environment project an increasing trend in glucose uptake and lactate production rather than oxidative phosphorylation in normal cells, which is called Warburg effect. As a result, lactic acid accumulates around tumors in a high concentration, which results in a lower pH value in the extracellular environment than in normal tissues (pH 7.2–7.4). The lower pH in endosomes and lysosomes can trigger the release of agents from acid-sensitive materials in the tumor regions. Taking advantage of the endogenous pH stimuli, different types of nanocarriers have been explored for developing pH-responsive drug delivery systems of Pt-based antitumor agents, such as polymersomes, liposomes, micelles, dendrimers, and inorganic and organic nanoparticles. These pH-responsive nanocarriers can preserve encapsulated Pt-based agents in blood circulation. However, they are responsive to the weak acidic environment of solid tumors and can release the integrated Pt-based agents near the tumor cells.14,15
2.1.1 Liposome Pt-based nanodrug delivery system. Liposomes are an artificial membrane, which is very effective for improving the reactions of some clinical compounds. They can encapsulate amphiphilic drugs, that is, combine hydrophilic drugs in the water chamber, and encapsulate lipophilic drugs in the lipid bilayer.16,17 In fact, several drugs with liposomal delivery systems have been approved and several other liposomal drugs are in clinical trials (Table 1). For Pt-based drugs, the liposomal formulations used in preclinical and clinical trials have shown their ability to maintain efficacy while reducing side effects. Liposomal formulation for Pt-based agents, such as SPI-077 (ref. 18) and lipoplatin,19 provided a novel approach to overcome the shortcomings of Pt-based drugs. SpHL-CDDP, prepared with dioleoylphosphatidylethanolamine (DOPE) and phosphatidylethanolamine (PE), is a pH-sensitive liposome formulation for cisplatin. The formulation also includes cholesteryl hemisuccinate (CHEMS) and distearoylphosphatidylethanolaminepolyethyleneglycol2000 (DSPE-PEG2000). CHEMS and DOPE are the key components to release CDDP from the liposomal formulation. Because CHEMS is negatively charged at neutral pH, it provides an electrostatic repulsion in the bilayer, reducing intermolecular interactions of DOPE. After the formulation is broken down, the entrapped drug is released rapidly when it is under acidic conditions. These properties reveal an advantage of tumor cells, the acidic microenvironment. This kind of behavior can cut down the toxicity of free CDDP on the whole body after administration.20,21 This classical pH-sensitive liposome formulation for Pt-based compounds strongly indicates that liposomes represent the prospect of clinical applied research of Pt-based derivatives. Furthermore, this review only addressed the application of single-function pH-sensitive liposomal systems in the development of platinum drugs. The dual/multi pH-sensitive liposomal systems have been reported and are expected to show promising results in the study of Pt-based antitumor drugs.22
Table 1 Liposomal platinum preparations in clinical trials
Name |
Drug |
Composition |
SPI-077 |
Cisplatin |
HSPC/CH/DSPE-PEG |
Lipoplatin |
Cisplatin |
DPPG/HSPC/CH/DSPE-mMPEG |
SpHL |
Cisplatin |
DOPE/PE/CHEMS/DSPE-PEG |
LTSL-CDDP |
Cisplatin |
DPPC/MSPC/DSPE-PEG |
SPI-077B103 |
Cisplatin |
Uns-lipid/CH/DSPE-PEG |
Lipoxal |
Oxaliplatin |
HSPC/DPPG/CH/DSPE-PEG |
LiPlaCis |
Cisplatin |
DSPC/DSPG/PEG-PE |
MBP-426 |
Oxaliplatin |
PE-transferrin/PEG-PE |
Aroplatin |
cis-Bisneodecanoato-trans-R,R-1,2-diaminocyclohexane platinum |
DMPC/DMPG |
2.1.2 Polymer Pt-based nanodrug delivery system. Polymersomes are supramolecular polymers, which are similar to cell membranes in structure and can be prepared from amphiphilic polymer blocks. The spherical nano structures include an aqueous core and a hydrophobic membrane. By combining with specific ligands, stimulating the release of ligands and modifying the polymer surface, it can promote the uptake and targeted release of anticancer drugs by cells.23,24 Because the stabilization and ductility of the polymersomes surpass those of liposomes, they can effectively protect the drugs in them from being hydrolyzed or degraded by enzymes, so as to retain their biological activity.25,26 Polymers administered via the system can touch off the drug after the drug hits its target. Therefore, based on the low pH environment near the tumor, numerous pH-responsive polymersomes that can pack, convey, and control the targeted release of Pt-based anticancer drugs were designed.27 The representation of polymerizate-bound Pt-based chemotherapeutic agents is poly (HPMA)-GGG-Ama = Pt= (1R,2R)-DACH (AP5346). AP5346 is a macromolecule consisting of biocompatible and water soluble poly hydroxypropylmethacrylamide (poly(HPMA)) bound to an oxaliplatin analog via a pH-sensitive chelating group. In a weak acid environment, platinum releases more rapidly after linking with the polymer through the pH sensitive group, which is common in many tumors. Both preclinical and phase I/II monotherapy study data indicate that AP5346 exhibits superior activity to oxaliplatin, while demonstrating excellent tolerability. Another typical polymerizate-bound Pt-based chemotherapeutant is AP5280, poly(HPMA)–carboplatin conjugate, which has a similar structure to AP5346 (Fig. 1).28
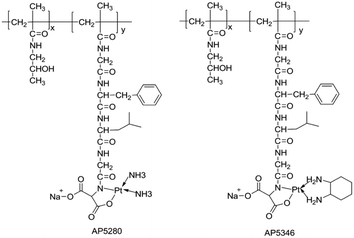 |
| Fig. 1 Polymer-bound Pt complexes AP5280 and AP5346 showing a similar structure. | |
Apart from being stable ligands of Pt-based drugs, polymers can also offer departure groups for their coordination. According to the structure–activity relationship of Pt-based drugs, carboxyl and hydroxyl perssads are the most aspiration leaving perssads. At present, many polymer–Pt conjugates are obtained by combining polymers that can provide –OH and –COOH perssads with platinum drugs (Fig. 2).29–31 Activated platinum drugs can be set free by hydrolysis of these conjugates. These characteristics provide conditions for the stimulatory response release of Pt-based drugs supported by polymer nanocarriers. For example, Lee et al. developed a cisplatin nanogel containing chondroitin sulfate (CS), and then added CS to the pH-responsive bioabsorbable polyethylene glycol (β urethane carbamate). By controlling the pH to release cisplatin, cisplatin was specifically delivered to cancer cells and the purpose of cancer treatment was achieved.32
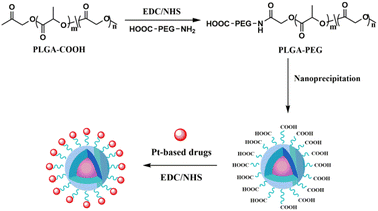 |
| Fig. 2 Polymers coordinated with Pt-based prodrugs by leaving groups. | |
Furthermore, encapsulating and conjugating drugs with nanocarriers to stimulate drug release in vivo provide a novel idea for the research and development of Pt-based drugs for pH-responsive delivery. Single polymer-caged nanobins (PCNs) offer a new means for building synergy into combination chemotherapy regimens. SonBinh T Nguyen constructed a single PCN for synergistic cisplatin–doxorubicin combination chemotherapy successfully. High density of doxorubicin can be encapsulated in the liposome core by PCNs. Around the liposome are pH-responsive polymer cages containing Pt prodrugs modified with various Pt/DOX ratios (Fig. 3). The drug release attributes of these PCNs in sour environments can enhance drug avidity and have an excellent synergistic effect when compared to dissociated drug combinations or single nano packing drugs.33
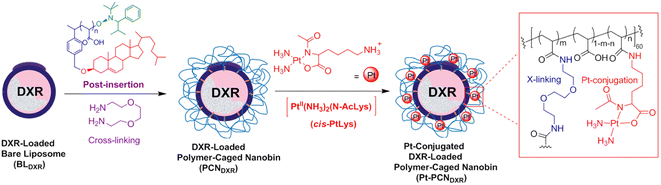 |
| Fig. 3 PCN conjugated platinum and loaded doxorubicin containing Pt prodrugs modified with various Pt/DOX ratios. | |
As a biodegradable polymer, acetylated dextran (Ac-Dex) has proven to be a latent candidate for medicine delivery applications.34 According to the standard lotion method, oil-in-water or water-in-oil-in-water, the acetylated dextran polymer is manufactured into micro and nano particles, which can be used for enclosure of hydrophobic or hydrophilic compounds. Because acetal radical is easy to hydrolyze in a weak acid environment (pH 5.5), and transfer back to its precursor dextran, it is considered that the degradation of the polymer containing acetal radical is pH-responsive.35 Nanoparticles based on the biodegradable Ac-Dex polymers used for governing send Pt(IV) prodrugs to cancer cells. This novel nano machinery possessed high drug loading capacity, high potting productivity, and high stability and pH-responsiveness in normal physical circumstances.36
2.1.3 Peptide Pt-based nanodrug delivery system. Peptides are secure and efficacious nanocarriers that can deliver non-viral genes to cells.37 They can penetrate the cell membrane, fuse endosomes and deliver substances to the nucleus. Furthermore, polypeptides are also a kind of carrier that can efficiently deliver drugs and genes, such as glutamic–acid–alanine–leucine–alanine (GALA), GALA short chain (shGALA) and lysine–alanine–leucine–alanine (KALA).38,39 Greg G. Qiao developed a class of novel smart delivery systems which lodge polypeptide vesicles loaded with cisplatin. These vesicles were copolymers formed after the coupling of the maleimide-poly(ethylene oxide)114-b-poly(L-glutamic acid)12 (Mal-PEG114-b-PLG12) block and cisplatin (Fig. 4). The study on drug release rate capacity found that the drug release rate was high “burst-like” in the weak acid environment of tumor cells (pH = 5.2), while the release rate was low in physiological conditions (pH = 7.4).40
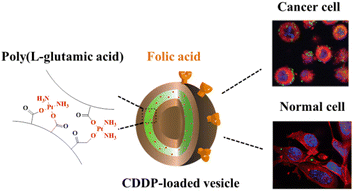 |
| Fig. 4 Peptide-based block copolymers conjugated with cisplatin. | |
2.1.4 Micelle Pt-based nanodrug delivery system. The polymeric micelles born by amphiphilic block copolymerization that can pot the medicine in their hydrophobic core are nanoscale carriers with a core and shell structure. By condensation of Pt2+ with the anions of poly(ethylene oxide)-b-polymethacrylate, a core shell co block ionomer composite micelle is obtained. Then, through chemical crosslinking of multi ion chains in the micellar core, a core crosslinking block ionomer with high cisplatin loading force can be synthesized. The ion module in its structure is pH-sensitive and can control the trigging and release of cisplatin (Fig. 5A). The core crosslinking method can effectively avoid structure dissolution, stabilize micelles and guard against medicine release precautiously.41,42 As a result of CDDP loading, cl-micelles showed pH-sensitive release of Pt(II) species in a sustained manner, which can be controlled by making adjustments to cross-linking levels.43,44 CDDP formulated as NC-6004 in a polymeric micellar form was developed by Dr Kataoka's group (Fig. 5B).45 Based on the PEO-poly(glutamic acid) (PEO-P(Glu)) block copolymer, a polymeric micelle, showing excellent pH-stimulation release, was formed after forming a complex with CDDP and adhering to the CDDP. These micelles were used in the development of a new delivery system with CDDP-loaded micelles by Afsaneh Lavasanifar et al. The pH-responsive polymeric micelles delivering CDDP were developed using methyl poly(ethylene oxide)-block-poly(carboxylate-caprolactone). Poly(f-caprolactone) particles were prepared by complexing CDDP with pendant carboxyl groups. A pH difference of 7.4, 6.0 and 5.0 was evaluated to determine whether CDDP was released in vivo. The nanopolymer-based micelles slowly released CDDP at physiological pH levels. As a result, CDDP is released from polymeric micelles in the presence of acidic conditions mimicking those found within the extracellular microenvironment of tumors or within the cells' internal organelles (Fig. 5C).46 The newly synthesized PEO-b-poly(methacrylic acid) block copolymer was modified with platinum by post-polymerization to form an acid-degradable micelle. In response to acidic stimuli, hydrazone linkages were formed, which were hydrolytically degraded more than 10 times at pH 5. An acid-sensitive response is a factor that triggers the release of platinum at the tumor site. In vitro experiments have shown high cytotoxicity for micelles containing platinum. Nanocarriers based on platinum are hydrolytically degradable and offer promise as a means to release drugs.47
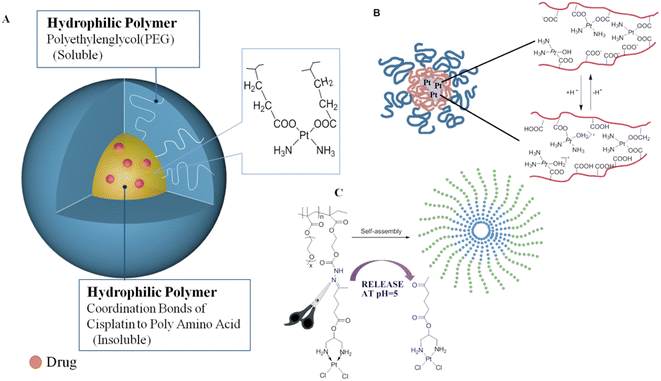 |
| Fig. 5 Micelle-based pH-responsive nanodrug delivery system: (A) nuclear-crosslinked block ionomers with high cisplatin adsorption; (B) NC-6004 prepared in the form of CDDP polymer micelles; (C) release of CDDP from polymer micelles under a simulated tumor microenvironment. | |
2.1.5 Dendrimer Pt-based nanodrug delivery system. Several properties of dendrimers have attracted attention in drug delivery, including their high branching, tunable surfaces, and precise molecular weights, and low intrinsic viscosity which can facilitate blood transport.48–50 Dendrimers can covalently link to or encapsulate anticancer drugs, improving their stability and decreasing their toxicity. In addition, dendrimers can also be easily modified with different functional moieties to be used for targeted diagnosis and therapy. The release of payloads by modified dendrimers is stimuli-responsive, which has distinct advantages over passive release. In stimuli-responsive delivery systems, enzymes, hypoxia, and tumor acidity act as triggers. Researchers have extensively studied pH-responsive delivery systems as one of the most promising solutions. In a study, Zhongwei Gu and colleagues presented dendrimer–DACHPt conjugates as pH-responsive drug delivery vehicles containing mPEGylated peptide dendrimers linked to Pt(II) (dendrimer-DACHPt) (Fig. 6A). DACHPt, which has a similar structure to oxaliplatin, was chelated with dendrimers via N,O-chelates. Dendrimer–DACHPt conjugates were released significantly faster at pH 5.0 than at pH 7.4 (alkaline environment). A study of the conjugate's in vitro efficacy and side effects demonstrated that it suppressed tumor growth better than clinical oxaliplatin and showed less toxic side effects on normal tissues.51 Xinyun Zhu et al. reported a dendrimer–platinum(II) complex responsive to tumor pH (Suc-HPMHO-CDDP) containing carboxyl-modified hyper-branched polyether (Suc-HPMHO) and cisplatin (Fig. 6B). Suc-HPMHO benefits from the existence of hydrophobic nucleus and the ionization of the surface carboxylic acid on the surface, showing a reversible pH reaction in the aqueous solution. Suc-HPMHO-CDDP is mainly deposited around the cell/around the cells. Therefore, the pH responsive release of the branches of the tumor tissues can be achieved.52
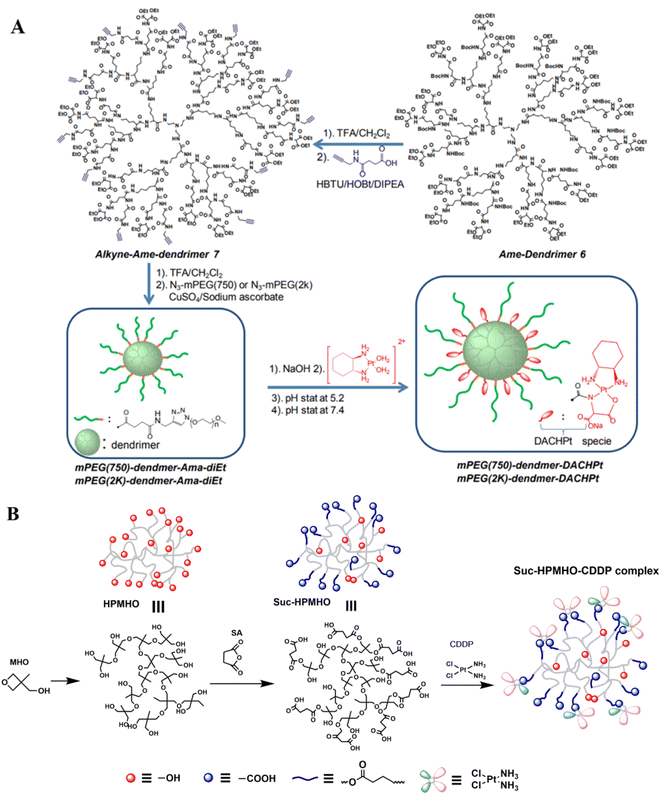 |
| Fig. 6 Synthesis of dendrimer-based pH-responsive nanodrugs.51 (A) Dendrimer-DACHPt; (B) dendrimer containing carboxyl-modified hyper-branched polyether (Suc-HPMHO) and cisplatin. | |
A novel stimuli-responsive clustered nanoparticle was constructed through molecular assembly of platinum prodrug-conjugated poly(amidoamine)-graft-polycaprolactone dendrimers (PCL-CDM-PAMAM/Pt) (Fig. 7). The initial size of the nanoparticle of the intelligent cluster is about 100 nm to achieve longer circulation and selective exudation. However, once the tumor is stopped, the inherent tumor extracellular acidity will trigger the PCL-CDM-PAMAM/Pt exclusion and promote tumor penetration. Finally, it can quickly reduce PAMAM to release active and effective cisplatin in the restored cytoplasm to kill cancer cells and lead to a strong antitumor effect.53
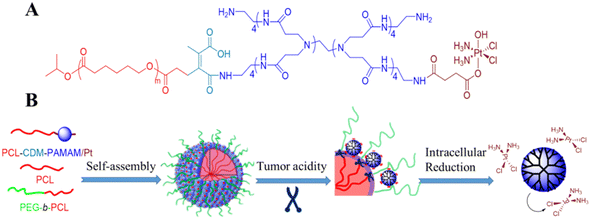 |
| Fig. 7 Dendrimer-based pH-responsive nanodrug delivery system. (A) PCL-CDM-PAMAM/Pt; (B) construction and pH-responsive release of PCL-CDM-PAMAM/Pt-based nanodrug delivery system. | |
2.1.6 Mesoporous silica nanoparticle Pt-based prodrug delivery system. As one of the most promising inorganic drug delivery systems, mesoporous silica nanoparticles (MSNPs) have high loading capacity, biocompatibility, easy production capacity and high degree of tunability regarding size, morphology and pore diameter.54,55 In recent years, MSNPs have been widely studied as targeted drug delivery systems for Pt-based drugs, and have made much major progress. For instance, the MSN nanocarriers with high-density carboxyl prepared by Gu et al.56 can combine with the platinum atoms in cisplatin. However, when targeted MSNPs accompany drug delivery systems into tumor tissues/cells, some problems need to be overcome to improve the release of Pt-based drugs loaded in MSNPs. In the MSNPs-Pt systems, the intrinsic tumor extracellular acidic environment is one of the best stimulating factors to trigger the release of platinum drugs. Inspired by the above-mentioned works, an MSN-oxaliplatin conjugate (MSN-Pt) stimuli-responsive delivery system was designed and synthesized (Fig. 8A). This MSN-Pt showed good pH-responsive release performance, with nearly 70% of the 24-h-loaded platinum release at pH 5.0 and only 20% at pH 7.4. In vitro MTT assay of MSN-Pt demonstrated an improved cytotoxicity against HepG-2 cells compared to free oxaliplatin.57 Through the hydrazone bond, Chiahung Lee et al. developed a novel cisplatin delivery system using modified MSNP surfaces. MSN surfaces modified with carboxylates and CDDP substituted with hydroxo can be used to further immobilize CDDP (Fig. 8B). Depending on the pH in the endosomes or lysosomes, the formulation can be extremely effective at increasing cellular uptake and drug release efficiency. CDDP prodrugs may also be encapsulated within MSNs in order to minimize non-specific release during enzyme hydrolysis.58
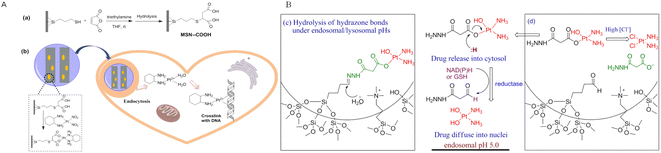 |
| Fig. 8 (A) MSNP-based pH-responsive nanodrug delivery system; (B) MSN surfaces modified with carboxylates and CDDP substituted with hydroxo. | |
2.1.7 Metal–organic framework Pt-based nanodrug delivery system. Metal–organic frameworks (MOFs) are a new family of inorganic–organic materials that are self-assembled by metals, including metal ions, clusters and chains with organic linkers. Due to their high drug-loading capacities, easy functionalization, high loading capacity, good biodegradability, and good biocompatibility, MOFs have gained considerable interest in nanotechnology, particularly in the field of Pt-based drug delivery systems.59 Because of the acidic tumor microenvironment and the sensitivity of the coordination bonds in MOFs to external pH, pH-responsive MOF nanocarriers are the most widely studied of all MOF nanocarriers.60 Various MOFs used as platinum drug delivery carriers have been reported.61–63 Sun Baiwang reported a novel biocompatible 2D MOF loading cisplatin through forming favorable carboxyl–drug interactions (Fig. 9A). The biocompatible MOFs possess good stability in physiological conditions but quick release behavior in response to the weak acid tumor microenvironment.64 Nanoscale coordination polymers (NCPs) are a class of “soft” materials constructed from metal ion connectors and polydentate bridging ligands. NCPs can alternatively be designed for controlled release of biologically functional species by exploiting their inherent solubility in an aqueous environment.65,66 NCPs were studied as a novel nanocarrier for stimuli-responsive drug release of Pt-based agents. Wenbin Lin et al.67 reported a novel and general strategy for the delivery of Pt-based drugs to cancer cells via their inclusion into NCPs (Fig. 9B). NCPs constructed from Tb3+ ions and c,c,t-(diamminedichlorodisuccinato) Pt(IV) bridging ligands were precipitated from an aqueous solution of the components via the addition of a poor solvent. The Pt-based NCPs are stabilized with shells of amorphous silica to prevent rapid dissolution and to effectively control the release of the Pt species.
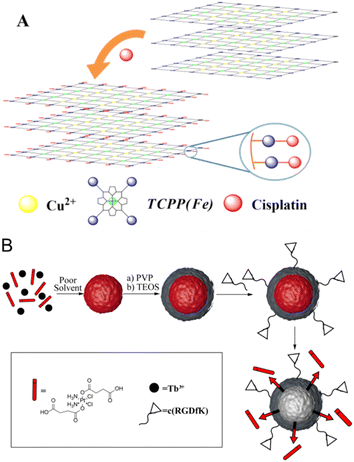 |
| Fig. 9 (A) Novel biocompatible 2D MOFs loading cisplatin through forming favorable carboxyl–drug interactions; (B) NCPs for Pt(IV) prodrug delivery. | |
2.1.8 Magnetic nanoparticle Pt-based prodrug delivery system. Iron oxide nanomaterials have attracted much attention as platinum-targeted drug carriers recently, due to their magnetic field-mediated targeting and magnetic resonance in diagnostic and therapeutic applications, especially as biocompatible magnetite (ferric oxide) nanoparticles (MNPs).68 As magnetite is very sensitive to acidic and oxidative conditions, the presence of an outer protective layer is of great importance to ensure that this component remains stable. Polyacrylic acid (PAA)-coated MNPs were synthesized and used as a pH-responsive delivery system of cisplatin. The results indicated that this delivery system is stable in systemic conditions and delivers cargos in the acidic tumor environment. Simultaneously, the magnetic resonance (MR) and optical imaging modalities assisted in monitoring drug loading and cancer treatment.69The use of superparamagnetic iron oxide nanoparticles (SPIONs) in cancer therapy may be beneficial. In addition to their magnetic properties, surface-modified SPIONs are also biocompatible, biodegradable, and dispersible in water.70 Generally speaking, there are at least two methods to construct drug-SPION conjugates: (a) SPION coatings are embedded in hydrophobic media; (b) SiO2 matrices may incorporate both the drug and SPIONs. Zijian Guo et al. constructed a pH-responsive Pt-SPION drug delivery system using the first approach (Fig. 10). Dechlorinated cisplatin (CMDP) is tethered to maghemite nanoparticles modified with 4-oxo-4-(3-(triethoxysilyl)propylamino)-butanoic acid (OTPBA-SPION) through the surface carboxylate groups. The CMDP-OTPBA-SPION revealed high Pt loading capacity and showed higher DNA binding capacity in acidic media than in physiological conditions, suggesting that the acidic cancerous environment favors the release of Pt-based agents.71 Incorporating both the drug and SPIONs in a SiO2 matrix to construct the formulation of Pt-based-SPION conjugates has not been reported.71
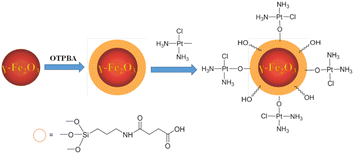 |
| Fig. 10 MNP-based pH-responsive nanodrug delivery system. | |
Because graphene-based magnetic nanoparticles have high chemical and thermal stability, high charge carrier mobility, and large surfaces, they are ideal for functionalization. The microenvironments of tumor endosomes and lysosomes can be used to trigger pH-responsive controlled drug release systems. However, the superparamagnetic core of these nanocomposites generates heat in the tumor, thereby killing cancer cells. As a result of magnetic hyperthermia, drugs are released on abnormal tissues more efficiently.72 Multifunctional graphene-based magnetic nanomaterials have, therefore, combined magnetic mild hyperthermia with stimulation-responsive drug delivery for thermo-chemotherapy, which has been found to have a dramatic impact on the treatment of cancer and medicine.73,74 Carboplatin and oxaliplatin loaded on GO-Fe3O4-PANI NPs were used as drug models to study the drug release in vitro. The results indicated that the release of carboplatin was more sensitive to pH, and the amounts released in neutral and acidic environments (pH 6.0 and 7.4, respectively) were higher than in alkaline environments (pH 8.0). Meanwhile, the release of oxaliplatin has no significant change at different pHs.75 The results suggest that GO-Fe3O4-PANI NPs are biocompatible and these particular NP drug delivery systems can lead to advances in cancer treatment.
2.2 Enzyme responsive drug delivery
Bio-nanotechnology relies heavily on enzymes because of their outstanding capabilities in bio-recognition and catalysis. In nanomedicine, they are extremely beneficial due to their high selectivity and high efficiency. Upregulation and overexpression of different secreted or membrane-bound enzymes, including proteases, MMPs, and glycosidases, are characteristic of most invasive diseases, including cancer. Drug delivery systems for cancer treatment can be designed by triggering selective drug release with enzymes as biological stimuli.76–78 Numerous efforts have been made to exploit the properties of sustained enzyme overexpression in cancer tissues to activate prodrugs or to transport them to tumor cells for releasing and then activating them by cellular enzymes. Furthermore, nanoparticle carriers are able to control and target drug delivery to diseased tissues, thus avoiding systemic side effects. Spatiotemporal control of drug release can be achieved using certain nanocarriers capable of responding to elevated levels of disease-specific enzymes.79,80 Therefore, enzyme responsive Pt-based drug delivery systems with nanocarriers are a promising strategy for cancer therapy. Bronich et al. reported functional biodegradable and biocompatible polypeptide-based polymeric micelles that can realize the goal of co-delivery of CDDP and paclitaxel at a controlled ratio to the cancer cells through enzymatic hydrolysis of the drug loaded polypeptide-based polymeric micelles.81 Shin Jung et al. prepared cisplatin-incorporated nanoparticles based on ion complex formation between hyaluronic acid (HA) and cisplatin for antitumor drug delivery.82
Based on the previous study, Zijian Guo et al. constructed a novel enzyme-responsive drug delivery system with PEGylated SPIONs and c,t,c-[PtCl2(OH)(O2CCH2CH2CO2H)(NH3)2] (HSPt) (Fig. 11). An HSPt-PEG-SPION is formed by loading HSPt onto PEGylated SPIONs. Cancer cells respond to HSPt-PEG-SPIONs as well as cisplatin, but normal cells are relatively unaffected. Glutathione (GSH) is able to dissociate and reduce HSPt-PEG-SPION species into Pt(II) species. A positive correlation exists between HSPt-PEG-SPION cytotoxicity and tumor cell GSH levels.83
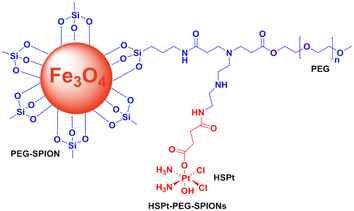 |
| Fig. 11 HSPt-PEG-SPION nanodrug delivery system. | |
Biomedical applications like drug repositories have been studied with carbon nanotubes (CNTs) hollow interior spaces. For further development of CNTs as smart materials, Chengkuo Lee et al. developed an innovative “gold–carbon nanobottle” drug delivery system (Fig. 12). Various cleavable linkages were used to connect the nanobottle-capped AuNPs to the open tips of MWCNTs, such as hydrazine, ester, and disulfide-containing linkages. CDDP was encapsulated in CNTs. The in vitro release studies showed that only disulfide linkage linked AuNP-capped CNTs demonstrated controlled release of CDDP selectively in reducing conditions, which mimic the intracellular environment. Therefore, by tuning capping strategies or introducing targeting groups, the development of a MWCNT-based enzyme-responsive delivery system with selective bond cleavage and localized hyperthermia could enhance current CDDP treatment methods.84
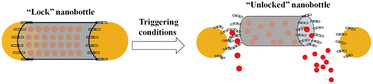 |
| Fig. 12 CNT-based enzyme responsive nanodrug delivery system. | |
In a paper by Seongbong Jo et al., a self-assembling peptide amphiphile (PA) containing a matrix metalloproteinase-2 (MMP-2)-sensitive GTAGLIGQRGDS and a fatty acid was developed for delivery of CDDP. By incubating CDDP and PAs together, PAs self-assemble into nanofibers, which are physically cross-linked to form a CDDP-PA nanofiber gel. By cleaving the MMP-2-sensitive sequence in the PA, the self-assembled CDDP-PA nanofiber gels released CDDP, directly proportional to the concentration of the enzyme. A biomimetic PA containing MMP-2-sensitive and integrin-binding peptides has great potential for enzyme-responsive Pt-based drug delivery.85
It is well known that multifunctional mesoporous silica nanoparticles (MSNs) are attractive carriers for delivering drugs. There is the possibility of selectively functionalizing these carriers at specific locations within nanoparticles. The outer-shell functionalization, for example, allows external functions to only be attached to the particle's outer surface, which does not interfere with the pore environment. A controlled drug release system can be created by exploiting this mechanism.86 Avidin-capped MSNs functionalized with linkers that are specifically cleaved by matrix metalloproteinase 9 (MMP9) were developed by Thomas Bein's research group.87 Through the use of ex vivo 3D lung tissue technology, they demonstrate that MMP triggers drug release from MSNs in human tumor cells.
By controlling drug release based on MMP activity, Gemeinhart et al. developed hydrogel-based drug delivery systems. In this study, CDDP was complexed with peptide-linkers (peptide-substrate) and incorporated into poly(ethylene glycol) diacrylate hydrogel wafers with different lengths of poly(ethylene glycol) chains. The results suggested that CDDP can be retained in PEGDA hydrogels by complexation with aspartate-containing peptides and released at an accelerated rate when MMPs are present.88
Jayakannan et al. discovered novel GSH resistant polymer-cisplatin core shell nanoparticles which were designed based on biodegradable carboxylic functional polycaprolactone (PCL)-block-polyethylene glycol diblock copolymers (PEG-b-CPCL). In the central core, CDDP was chemically conjugated with Pt-OORPCL, which was shielded by the PEG shells at the periphery. The study of the in vitro release of cisplatin showed that the PEG shell protected the drug from the attacks of cytoplasmic thiol residues, GSH, allowing it to remain free from detoxification.89 Cheng et al. reported a novel enzyme-responsive delivery system with dendrimer-encapsulated gold nanoparticles (DEGNPs) (Fig. 13). These DEGNPs showed glutathione-triggered “off–on” release behavior of anticancer drugs. A low-dose release behavior was observed for drugs loaded within DEGNPs containing thiol compounds when thiol-reducing agents such as glutathione and dithiothreitol were present. In comparison with the free anticancer compounds, the thiolated doxorubicin and cisplatin loaded within nanoparticles were much less cytotoxic.90 Based on a novel polymer micelle containing tellurium, Xu et al. reported a competitive coordination system (Fig. 14). Water-soluble polymers were used as the first vehicle for delivering tellurium. Using the method of chemical coordination of platinum and tellurium, Pt-based drugs could be loaded. Biomolecules compete with each other to release the drugs, which is controlled by tellurium-containing polymers.91,92
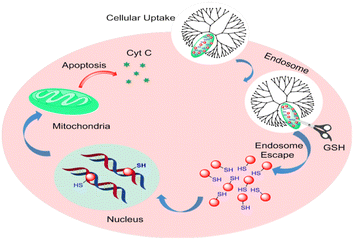 |
| Fig. 13 DEGNP-based enzyme responsive nanodrug delivery system. | |
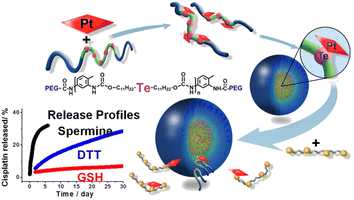 |
| Fig. 14 Te-based micelle enzyme responsive nanodrug delivery system.91 | |
2.3 Redox responsive drug delivery
Redox-sensitive PNPs may be used in controlled drug delivery as a switchable responsive nanomaterial. These systems are interesting because of their differing redox potentials between the extracellular and intracellular spaces. Typical intracellular concentrations of reduced GSH are 10 mM in the cytosol, while extracellular concentrations are only 0.002 mM. The cytosol has a low redox potential due to the abundance of reduced GSH.93 Additionally, tumor cells possess significantly higher levels of GSH than normal cells. This redox potential can be exploited to develop redox-sensitive intracellular delivery therapeutic strategies. Disulfide bonds, which are stable in oxidizing extracellular environments, are cleaved in reducing environments due to the reduction of the disulfide to thiol groups in oxidizing enzymes.94 Rezaei et al. have developed redox-responsive Pt(II) prodrug micelles (Fig. 15A). Physiological conditions appear to be relatively stable for the release of Pt(II) prodrugs in vitro. When disulfide links are rapidly cleaved, conjugated platinum drugs are released.95 Cisplatin–polyethyleneimine conjugate BPEI-SS-Pt coated with HA was constructed for redox-sensitive delivery of cisplatin (Fig. 15B).96 Lin et al. prepared redox-sensitive prodrugs of Pt(IV) using metal–organic framework nanoparticles coated with silica.65,97
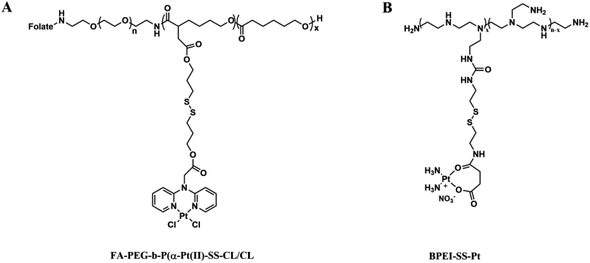 |
| Fig. 15 (A) Structure of folate-decorated polymeric Pt(II) prodrug micelles; (B) structure of micelles constructed by branched polyethyleneimine. | |
Novel cross-linked redox-responsive micelles that were able to deliver anticancer drugs and near-infrared (NIR) fluorescent agents for combined photothermal and chemotherapy were reported (Fig. 16). In this work, P(MEO2MA-co-MASI)-b-PIPMA), poly(N-(2-hydroxypropyl)methacrylamide) cross-linked with poly(N-(2-hydroxypropyl)methacrylamide, was synthesized by poly[(2-(2-methoxyethoxy)ethyl methacrylate)-co-(N-methacryloxy succinimide)]-block. Finally, the alkynyl-functionalized cypate was conjugated with a Pt(IV) complex via a click reaction. Cisplatin was released when hydrogen peroxide was added to CCL micelles. Photothermal and chemotherapy synergistically increase CCL micelle cytotoxicity at low concentrations.98
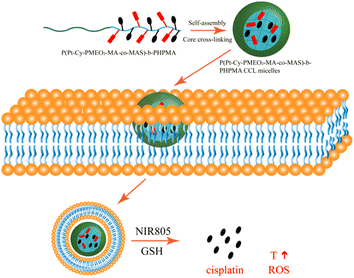 |
| Fig. 16 Micelle-based redox-responsive nanodrug delivery system. | |
In the future, nanoparticles could deliver Pt(IV) prodrugs to cells that would resist thiol-mediated detoxification by exhausting GSH. In order to maximize the therapeutic benefits of Pt-based drugs in cancer patients, Omid C. Farokhzad et al. have developed glutathione-responsive Pt(IV) prodrugs (Fig. 17). The delivery of lethal cargos by macropinocytosis is one of the most efficient methods for transporting nanoparticles across the cytomembrane. As a result, sufficient active Pt(II) metabolites were released, which covalently bound to target DNA and induced apoptosis after being reduced by GSH.99
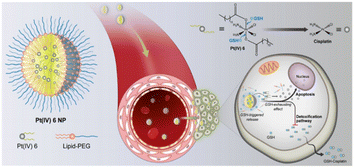 |
| Fig. 17 Glutathione redox-responsive nanoparticles for Pt(IV) prodrug delivery.99 | |
2.4 Thermo responsive drug delivery
Temperature has gained a great deal of attention for its potential to control the release of drugs from stimulation-responsive DDSs with spatiotemporal control. It is possible for most inflamed pathological sites and tumors to stimulate the body internally by their hyperthermic nature. Hyperthermia has been used clinically for decades as an adjuvant therapy in the treatment of solid tumors.100–102 Hyperthermia increases the permeability of the tumor tissue vasculature and thus enhances anticancer drug delivery to the tumor site.103 The idea of thermosensitive systems came from the temperature difference between diseased and healthy tissues. Thermo-responsive PNPs have drawn attention as smart materials for biomedical applications due to their phase-transition behavior in response to changes in temperature. As temperature changes are applied externally, thermo-responsive MNPs can also be activated, making them an attractive alternative for stimuli-responsive DDSs.104
Polymers that undergo a phase transition at a certain temperature can be characterized as thermally sensitive polymers. The thermal response of polymers is typically explained as a result of their balance between hydrophilic and hydrophobic moieties.105,106 Some polymers are temperature-responsive, which can be used to design stimuli-responsive delivery systems. Covalently cross-linked hydrogels are one class of thermally sensitive materials that have been extensively studied as a thermally responsive drug delivery system. Thermosensitive hydrogels of poly(N-isopropylacrylamide) (PNIPAAm) with chitosan (CPN) were prepared and evaluated for delivery of cisplatin and carboplatin. The effects of polymers containing different ratios of chitosan on the physicochemical and drug release characteristics were examined.107 As reported by Moura, two types of chitosan hydrogels loaded with cisplatin exist, those crosslinked by glycerol-phosphate disodium salt, and those crosslinked ionically/covalently. Cisplatin released from chitosan/glycosan (C/GP) hydrogels is significantly less than that from covalent/ionic cross-linked hydrogels 10 (C/GP/GE10) or C/GP/GE20 and the amount of drugs released from these two types of hydrogels were also rather different.108
According to the study by Chen, PLGA/Gypsum/PLGA hydrogels were used as a localized co-delivery system for doxorubicin (DOX), cisplatin and methotrexate (MTX) in the treatment of osteosarcoma (Fig. 18). The release profiles of the drugs from the hydrogels were investigated in vitro. Hydrogels containing multidrug coloads showed synergistic cytotoxicity against osteosarcoma Saos-2 cells and MG-63 cells.109
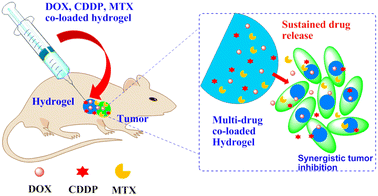 |
| Fig. 18 Co-delivery of DOX, CDDP, and MTX by thermosensitive hydrogels. | |
Temperature-sensitive nanocarriers depend on a strategy that raises bulk tumor temperature above a critical transition temperature to accomplish a phase change.110 However, raising bulk tumor temperature selectively and safely remains a significant challenge. There is a particular interest in carbon nanotubes, including single-walled carbon nanotubes (SWCNTs), which are derivatized, functionalized, and loaded with chemotherapeutic agents.111 It is also important to note that SWCNTs are unique and they support local electric field absorption and dissipate RF energy as heat and align parallel to the incident E-field.112–114 Curley et al. developed carbon nanocapsules comprised of an ultra-short carbon nanotube shell (US-tubes) loaded with cisplatin (CDDP@US-tubes) and covered with a Pluronic surfactant wrapping to minimize passive release (Fig. 19). Non-invasive RF fields activate CDDP@US-tubes, causing heat that disrupts Pluronic and triggers cisplatin release when activated with RF fields.115
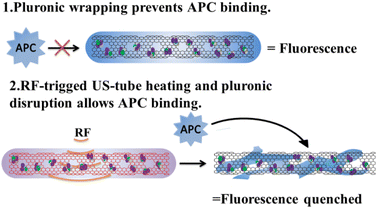 |
| Fig. 19 SWCNT-based thermo-responsive nanodrug delivery system. | |
3 Extracorporeal stimuli-responsive drug delivery
3.1 Ultrasound responsive drug delivery
To ensure that drugs are released at the desired location, ultrasounds provide spatiotemporal control, thereby preventing side effects to healthy organs. The use of ultrasounds is also attractive because they are not invasive, without ionizing radiation, and can easily regulate the depth of tissue penetration by adjusting the frequency, duty cycle, and exposure time.116,117
A variety of nanocarriers may release drugs after being subjected to ultrasound waves due to thermal and/or mechanical effects caused by cavitation phenomena or radiation forces (Fig. 20).118–121 Indeed, using cavitation mechanisms, it has been demonstrated that nanocarriers can be destabilized, drugs are released, and vessel permeability is increased, which promotes cellular uptake of therapeutic molecules.122,123
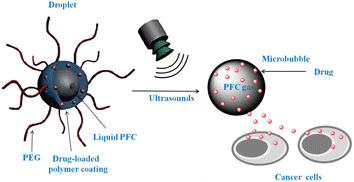 |
| Fig. 20 Ultrasound-responsive nanodrug delivery system. | |
In particular, low frequency ultrasound (LFUS) is used to improve membrane permeability.124–128 Also, LFUS increases liposome permeability, since liposomes have phospholipid bilayers similar to biological membranes.129 The group of Yechezkel Barenholz treated with liposomal cisplatin and LFUS scored the highest therapeutically when compared to all others. Utilizing LFUS, we demonstrate in vivo drug release from liposomes. Liposome-based formulations of cisplatin, such as SPI-77, failed in clinical trials due to their excellent stability. Schroeder et al. demonstrated that in mouse tumors treated with 20 kHz ultrasound the amount of cisplatin released from liposomes increased by nearly three times, from less than 3% to almost 70%.122,130
LFUS is able to reach the cavitation threshold, but the enhancement of ultrasound mediated vascular permeability may result in drawbacks such as metastatic diffusion. Therefore, in diagnostic frequencies, it has been found effective to use microbubbles or other agents that interact effectively with ultrasonic waves in order to reduce the cavitation threshold.131,132 Microbubbles for tissue targeting, however, might be limited by their short lifespan and lack of extravasation. A new method for overcoming this problem has been developed using perfluorocarbon nanoemulsions. These nanoemulsions transform into microbubbles when they are injected with ultrasound therapy. Acoustic droplets are vaporized and cavitated, resulting in the formation of bubbles that enhance Pt-based drug uptake and release from tumors.133–135 Consequently, metastatic dissemination has been suppressed, while therapeutic efficacy has increased.
High frequency ultrasound (HFUS) is capable of producing significant temperature rises. As mentioned above, during pressure wave propagation, acoustic energy is absorbed by tissue. Besides kinetic motion, energy is lost as the tissue heats up. When acoustic waves are focused by curved arrays or multiple elements, HFUS can lead to significant hyperthermia in a discrete region. HFUS has been used in a lot of research studies and trials as a targeting and drug release technique. Liposomes, polymer micelles, mesoporous silica nanoparticles and other nanomaterials as HFUS responsive drug carriers were reported.136–138
3.2 Light responsive drug delivery
In addition to stimulating or triggering drug release, light irradiation can also be used as an antidote. Molecular orbitals with higher band gaps will absorb more photon energy from light. Of the several stimuli used in smart DDSs, light irradiation has attracted a great deal of attention due to the ease with which it can precisely modulate its intensity, the ability to control exposure duration and tissue location, and the perceived noninvasive nature of light-modulated activation. The release of drugs from nanocarriers can be triggered using ultraviolet (UV), visible, and NIR irradiation.139–141
Another novel approach to triggering drug release in reducing environments, such as those associated with cancer, is the photo-reduction of Pt-based prodrugs. In this experiment, Pt(IV) prodrugs through photoirradiation are converted to the active cytotoxic drug Pt(II). As a result of photo-triggers such as UV, visible light and NIR irradiation, anticancer drugs are released in a photo-reductive way, which enhances the specific cytotoxicity of an anticancer drug, stimulates the apoptosis of cancerous cells, and inhibits the growth of cancerous cells; high bioavailability, high light penetration, significant inhibition of tumor growth, and simultaneous imaging capabilities may be achieved after the treatment is given in vivo.142,143
3.2.1 UV light. Photoactive Pt(IV)-azide prodrugs have been developed and converted to the biologically active Pt(II) form in vitro through mild UVA irradiation.144,145 Based on this work, Bilgicer et al. prepared a novel series of photo-sensitive Pt(IV)-azide prodrug-loaded micellar nanoparticles (Fig. 21). In vitro and in vivo experiments demonstrated that NC1-NC4 micellar nanoparticles had highly controlled and selective release of active Pt(II) at the tumor site, which enhanced efficacy and decreased systemic toxicity upon low energy UVA irradiation.146 Won Jong Kim et al. reported a novel photo-responsive nanoparticle comprising nitric oxide that releases platinum prodrugs and polymeric micelles. Photo-responsive nitrobenzene and photo-labile 4,5-dimethoxy-2-nitrobenzyl polymeric micelles were used to synthesize Pt(IV) prodrug nanoparticles for photo-triggered NO-release. As a result of ultraviolet light irradiation, nitric oxide was released. Photounstable hydrophobic groups within the micelle separate, while the nitric oxide-releasing donor changes to a more hydrophilic state, resulting in rapid release of the Pt(IV) precursor drugs.147
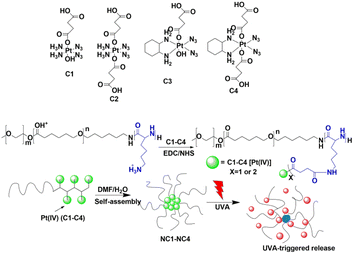 |
| Fig. 21 Micellar nanoparticle based light responsive delivery system. Concluding the structures and synthesis methods of photo-sensitive Pt(IV)-azide prodrugs. | |
3.2.2 NIR. Due to the low absorption and scattering of NIR in tissues, while hemoglobin, water, and lipids hardly absorb it, the transmission of NIR in tissues is better. In comparison with visible light, NIR has a lower energy per photon, which makes it less damaging to cells. The problem of NIR photons having low energy was addressed by developing organic chromophores able to simultaneously absorb two low-energy photons. Upconversion nanoparticles (UCNPs) are innovative materials that can overcome such fundamental defect of transition metal complexes. They have unique optical features and can be efficiently converted from NIR photons into visible and UV light via multi-photon energy transfer processes.148 Such novel and amazing photo conversion UCNPs as a powerful NIR-induced mediator in vitro and in vivo are widely used in the biomedical field, including remote control photodynamic inactivation of tumor,149 photothermal therapy,150 biological sensing and imaging,151 regulation of biological activity,152 and specific delivery of drug molecules and genes into target cells or living donor sites.153Salassa et al. developed novel photoactivated UCNPs capable of releasing Pt(II) species upon excitation with NIR light. Pt(IV) complex cis, cis, trans-[Pt(NH3)2Cl2(O2CCH2CH2CO2H)2] is photoactivated by NIR light using core–shell UCNPs. As nanoparticle surfaces are modified by combining this cisplatin prodrug with a biocompatible PEGylated phospholipid DSPE-PEG (2000)-NH2, this method is useful. Photoreaction experiments indicated that Pt(IV) is fully converted to Pt(II) at the end of the photoreaction.154 As part of this research, Guangyu Zhu et al. developed a multimodal nanoplatform using 808 nm-excited and biocompatible core–shell–shell UCNs covalently loaded with photosensitizers (PSs), rose bengal (RB) and Pt(IV) prodrugs c,c,t-[Pt(NH3)2Cl2(OCOCH2CH2NH2)2], respectively. Through the use of UCNPs, NIR light can be converted into visible light, which is used by RB as a source of singlet oxygen. Nanoplatforms deliver prodrugs into cancer cells at the same time. Thus, this upconversion nanoplatform enables the combination and synchronization of photodynamic therapy (PDT) and platinum chemotherapy.155 Incorporating upconversion luminescence, magnetic resonance (MR) imaging, and computer tomography trimodality imaging, as well as NIR-activated pre-platinum delivery, Lin Jun et al. developed a multifunctional drug delivery system (Fig. 22). As well as using UCNPs as drugs carriers, DPP (Pt(N3)2(NH3)(py)(CCH2CH2COOH)2) has been conjugated to UCNP surfaces. In addition to using UCNPs as carriers of drugs, DPP has been conjugated to UCNP surfaces using Pt(N3)2(NH3)(py)(CCH2CH2COOH)2. Hence, UCNPs-DPP-PEG can effectively kill cancer cells under 980 nm laser irradiation. Importantly, UCNPs-DPP-PEG inhibited tumor growth in mice under 980 nm laser irradiation compared to that under 365 nm UV irradiation. These results suggest that in practical cancer treatment, using the NIR to UV approach is more effective than using UV directly due to the higher tissue penetration depth of NIR light.156 On this basis, Lin Jun et al. reported a novel NIR light responsive dual-drug system with upconversion mesoporous silica nanostructures. Mesoporous silica coated UCNPs with a core–shell structure were prepared and loaded with the antitumor drug DOX. Both DOX and Pt(II) complexes kill cancer cells. This dual-drug delivery system may open a new avenue for the application of UCNPs in photoactivated cancer therapy.157
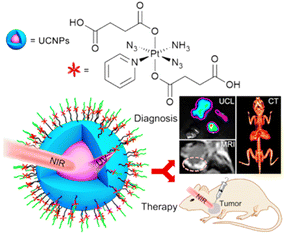 |
| Fig. 22 UCNP-based light responsive nanodrug delivery system combining photodynamic therapy (PDT) and platinum chemotherapy.156 | |
UCNPs modified with lanthanide elements have unique luminescence characteristics, and are an ideal carrier for remote control of light radiation. Xing et al. discovered a new personalized NIR-mediated antitumor drug activation system, which neutralizes a specific photoactive prodrug and an apoptosis-sensing peptide bound to the surface of silica coated UCNPs (Fig. 23). This NIR photoresponsive nano-conjugate can selectively trigger the local activation of the effective Pt(II) complex at the targeted tumor, maximize the light penetration and avoid non-specific toxicity to normal tissues, realize remote control of drug release, and real-time imaging, and evaluate the corresponding antitumor activity in the targeted tumor cell line.158
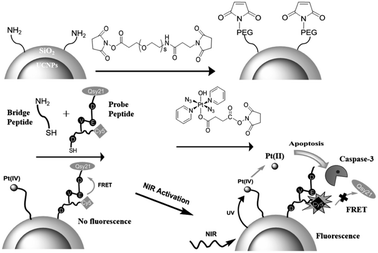 |
| Fig. 23 Lanthanide-doped UCNP-based light responsive nanodrug delivery system. | |
In addition to UCNPs, NIR is also used to trigger the release of Pt-based drugs. A nanoparticle based on a block polymer encapsulated with cisplatin and photosensitive indocyanine green (ICG) dye has been discovered by Li et al. (Fig. 24). The block polymer is modified with tellurium to combine with cisplatin platinum, but tellurium is easily oxidized by reactive oxygen species (ROS). Under the stimulation of 808 nm NIR, ICG dye produces singlet oxygen, which induces the release of cisplatin.159 The researchers also developed a new method α-cyclodextrin and polyethylene glycol modified resinous polymer encapsulated platinum nanoparticles, which are a NIR photo-responsive supramolecular hydrogel. After NIR irradiation, they are subject to photothermal degradation and can release therapeutic agents in a dose adjustable manner.150,160
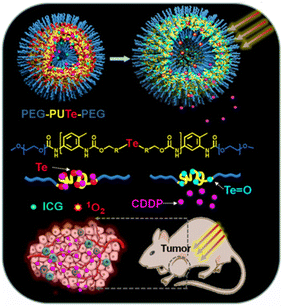 |
| Fig. 24 The block polymer based light responsive nanodrug delivery system.159 The nanoparticle encapsulated with cisplatin and photosensitive indocyanine green (ICG) dye. | |
Erythrocyte membrane coated nanocarriers are a promising drug delivery nano-treatment platform that retains the complete cell membrane structure and membrane protein, and has excellent cell-specific functions, such as long-term blood circulation and immune escape. By embedding 1,2-diaminocyclohexane Pt(II) (DACH-Pt) and indocyanine green in the red blood cell membrane (R-RBC@BPtI) modified by targeted peptide, it can be used to enhance tumor immunity and synergetic chemotherapy (Fig. 25). This nano red blood cell membrane has multiple cores, and has excellent stability and high encapsulation efficiency. Due to the prominent stealth and targeted modification function of the erythrocyte membrane, R-RBC@BPtI has significant targeted delivery and cell uptake function for DACHPt. After NIR irradiation, R-RBC@BPtI shows significant instability through singlet oxygen and thermo-mediated lysis, which can trigger drug release effectively and realize deep penetration and accumulation of DACHPt and ROS in tumor sites. Therefore, R-RBC@BPtI has targeted specificity for tumor. It can achieve tumor ablation and inhibit lung metastasis in vivo through the combination of phototherapy and chemotherapy.161
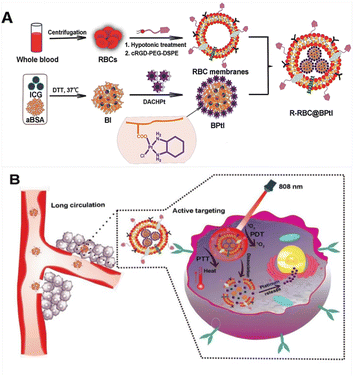 |
| Fig. 25 RBC membrane-cloaked nanoparticles as a light responsive nano drug delivery system. (A) R-RBC@BPtI; (B) R-RBC@BPtI shows significant instability under NIR irradiation.161 | |
3.2.3 Visible light. Visible light is rarely used in supramolecular assemblies. As a result of photoinduced electron transfer, Mareque-Rivas et al. reported the first application of quantum dots (QDs) to reduce Pt(IV) complexes and form Pt(II) complexes (Fig. 26). Based on the results, PET could be used to photoregulatedly generate a Pt(II) complex between the CdSe-ZnS QDs and the Pt(IV) complex. After 1 h, using low power visible laser light, about 2000–2200 molecules of Pt(II) were generated per QD despite the absence of covalent bonds. Photodynamic and Pt-based anticancer therapy could be revolutionized through the use of QDs and Pt(IV) prodrugs in this study.162 The mechanism of photoreduction of the Pt(IV) complex cis, cis, trans-[Pt-(NH3)2Cl2(O2CCH2CH2CO2H)2] into Pt(II) species by QDs, a process which holds potential for photodynamic therapy, was elucidated by Ivan Infante and his colleagues.163
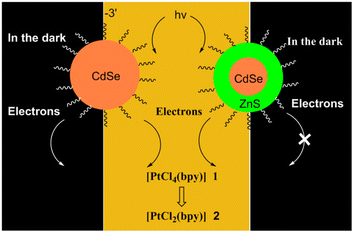 |
| Fig. 26 Around the QDs-based light responsive nanodrug delivery system, PET could be used to photoregulatedly generate a Pt(II) complex between the CdSe-ZnS QDs and the Pt(IV) complex. | |
3.2.4 Laser. As mentioned above, UV light, visible light and NIR irradiation were used to stimulate or trigger Pt-based drug release from nanocarriers. However, there are few studies on laser-triggered Pt-based drug release. Chiyi Xiong et al. reported a novel strategy of laser-triggered release of cisplatin from cisplatin-loaded hollow gold nanoparticles (HGNPs). The HGNP containing Pt(II) is obtained by complexing cisplatin with tripeptide (acetyl-GluGlu-Cys-NH2), and the Pt(III) ion forms a coordination bond with the carboxylic acid group of the side chain of glutamic acid residue. Under a NIR nanosecond pulse laser, cisplatin can accelerate the release by breaking the Au–S bond between the tripeptide connector and the Au surface. These research results show that the photothermal properties of HGNPs can be used for bimodal photothermal therapy and platinum drug chemotherapy triggered by NIR laser.164
3.3 Magnetic field responsive drug delivery
Magnetic-sensitive nanocarrier drug delivery systems of anticancer agents are one of the ideal candidates for drug targeting to the desirable site of action.165,166 Biocompatible magnetite nanoparticles have been widely used in clinical diagnosis and treatment for platinum drug delivery, especially iron oxide nanoparticles, due to their unique magnetic field-mediated targeting and magnetic resonance characteristics.167 For example, a magnetic-responsive nanocarrier drug delivery system based on carboplatin prodrug loaded Fe3O4 nanoparticles (MNPs@carboplatin) was developed (Fig. 27). MNPs@carboplatin has excellent delivery ability, can promote drug integration, lead to intracellular drug accumulation, and enhance cytotoxicity. Furthermore, MNPs@carboplatin can be widely distributed into major organs, and in the presence of an external magnetic field, the Fe3O4 nanocarrier is beneficial to visualize the tumor site location and promote the subsequent antitumor efficacy.168
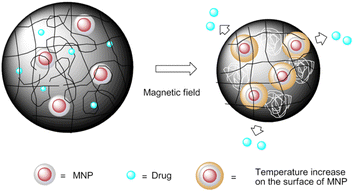 |
| Fig. 27 MNP-based magnetic responsive nanodrug delivery system. | |
In a study by Lin et al.169 a novel magnetic hybrid nanosystem for overcoming multidrug resistance of ovarian cancer through successfully delivering cisplatin and Fe3O4 NPs into the region of cancer cells was developed. This magnetic hybrid nanosystem can preferentially increase the Pt and Fe accumulation in the tumor site via magnetic-field mediated-localization and monitoring by MRI-guided delivery and also activated oxidases, which triggered a cascade reaction to form H2O2. The MNPs degraded and were metabolized in the cancer cells, releasing excess labile iron ions that catalyze H2O2 decomposition into highly toxic ROS within cancer cells. As magnetite is very sensitive to acidic and oxidative conditions, the presence of an outer protective layer is of great importance to ensure that this component remains stable. A novel strategy used to obtain protective layers is coating of the magnetite with a layer to obtain high stability under extreme conditions, a natural porous structure, and a platform for chelating groups.170
Administrating an encapsulation of Fe3O4 NPs into drug delivery systems such as liposomes,171–175 nanomicelles,176,177 or inorganic mesoporous silica nanoparticles178–181 can adequately exploit their favourable features in drug delivery stimulated by pH/T/light/ultrasound. These combination nanocarriers have also received considerable attention to trigger cargo release under the effect of a high-frequency alternating magnetic field (AMF). Liposomes loaded and decorated with MNPs are the type of nanoparticles most commonly used for magnetically induced drug release. These novel magnetoliposomes with high drug loading and better synergistic effect for cisplatin were reported by Baiwang Sun. Magnetoliposomes have been observed to internalize and release loading charges when exposed to AMF through hysteresis loss and/or Néel relaxation.182
Although magnetosensitive nanocarriers have been widely used in the drug delivery systems of antitumor agents, they are seldom used in the research of platinum antitumor drugs.166,170,183–186 In the future, the use of magnetite nanoparticles to deliver platinum drugs can enable the magnetic targeted delivery of drugs and potential MRI imaging capabilities.
4 Multistimuli-responsive drug delivery
Nowadays, increasing attention is being paid to engineering stimuli-responsive nanocarrier delivery systems for further optimizing antitumor drug delivery. However, most single-responsive nanosystems are limited by their slow and insufficient drug release at tumor target sites with reduced therapeutic efficacy since tumor cells exhibit remarkable heterogeneity within tumor tissues or even within single solid tumors. For these reasons, multiple stimuli-responsive nanoparticles are highly pursued for responding to heterogeneous and complicated biological microenvironments, allowing for sufficient site-specific delivery within tumor cells.187
4.1 pH/redox dual-responsive
In certain pathological situations, pH and redox responsiveness may be used together because of the coexistence of a pH gradient and an oxidative environment. The special inert Pt(IV) complexes have been widely used to minimize the adverse side effects that existed in the Pt(II)-based chemotherapies. Pt(IV) complexes regain their cytotoxicity when present at higher concentrations in the tumor cells than in blood and normal tissues, which are believed to be reduced to Pt(II) complexes by reductants like ascorbic acid and glutathione inside the cancer cells. As mentioned above, the tumor micro environment has an altered state compared with normal tissues. Therefore, the most important strategy for improving drug efficacy and specificity is to improve the capacity of reduction at pH < 7.1, where Pt(IV) prodrugs have both pH and redox dual-responsive properties in the cellular environment. Stimuli-triggered drug delivery and release can be achieved through nanocarriers.
The liposomes,188 polymers,189 micelles,190,191 inorganic nanomaterials192–195 and metal–organic frameworks10,196,197 were widely used to synthesize the Pt(IV) prodrugs with pH/redox responsive properties. Besides chemical nanomaterials, biomolecule nanomaterials were developed for responsive Pt-based drug release. Human serum albumin conjugated nanoparticles (HSA@NPs) for pH/redox dual-responsive Pt-based drug delivery were prepared by using hybrid nanoparticles of human serum albumin (HSA), calcium phosphate and a Pt(IV) prodrug of cisplatin (Fig. 28). The conjugate protected Pt(IV) prodrug in HSA from reduction under extracellular conditions. But low pH value and high glutathione concentration could trigger the conjugated nanoparticles to release their active species of cisplatin rapidly. As a result, this delivery system demonstrated more efficiently than cisplatin to various cancer cells but not to normal cells.198
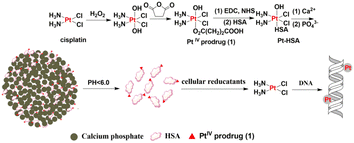 |
| Fig. 28 HSA@NP-based pH/redox dual-responsive nanodrug delivery system. Low pH value can trigger the conjugated nanoparticles to release their active species of cisplatin rapidly. | |
In addition to Pt(IV) complexes, Pt(II) complexes are also available for pH/reduction dual-responsive metallodrugs.
A pH/redox dual-responsive theranostic nanoplatform (TN) for efficient Pt-based drug delivery and NIR tracking was constructed with supramolecular PEGylated dendritic and Pt(II) derivatives. Supramolecular dendritic template was stabilized by bioreducible disulfide bonds and endowed with NIR fluorescence probes. PEGylated platinum derivatives were coordinated onto the abundant peripheral groups of supramolecular dendritic templates. Upon reaching the acidic and hypoxic environment, the TN was disassembled quickly due to acid-labile coordination bonds and redox-cleavable disulfide linkages, and then Pt-based drugs were delivered into the nuclei to exert antitumor activity. In vivo antitumor treatments indicated that TN not only provided high antitumor efficiency which was comparable to that of clinical cisplatin, but also reduced renal toxicity of Pt-based drugs. Moreover, NIR fluorescence of the TN successfully visualized in vitro and in vivo fate of nanoplatforms and disclosed the intracellular platinum delivery and pharmacokinetics.199
Hierarchical stimuli-responsive nanocarriers, which can change their properties by responding to either internal and intrinsic environments of tumor or external and artificial stimuli, attracted much attention gradually.200–204 A transactivator of transcription (TAT)-presenting hierarchical stimuli-responsive nanomedicine (DATAT-NP/Pt) was constructed (Fig. 29). The TAT peptide bond with import receptors can promote cellular internalization and actively transport cargos into cellular nuclei. Simultaneously, it was modified with a tumor acidity-activated mask that can escape recognition by the RES and consequently causes rapid removal from blood circulation. The masked TAT peptide can be reactivated by the extracellular pH of tumor tissue to markedly enhance the binding of TAT with the extracellular matrix and tumor cells and facilitate cellular translocation to the perinuclear region. The encapsulated Pt(IV) prodrug was released in the perinuclear region and cell nucleus and reduced to generate the cytotoxic Pt(II) species, which resulted in the formation of Pt and DNA adducts in cell nuclei, thereby sensitizing the drug-resistant cells to chemotherapy. The mechanism has been comprehensively evaluated in vivo.205
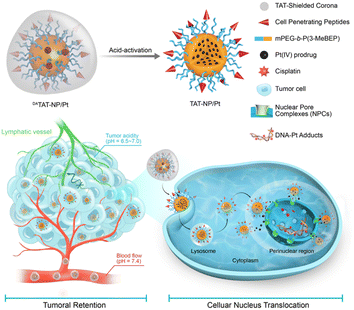 |
| Fig. 29 DATAT-NP/Pt hierarchical stimuli-responsive nanodrug delivery system.205 The TAT peptide bond with import receptors can promote cellular internalization and actively transport cargos into cellular nuclei. | |
Hierarchically stimuli-responsive nanocarriers based on hollow mesoporous silica nanoparticles (HMSNs) were constructed by electrostatic complexation between the functional HMSNs loaded with gemcitabine (GEM) and small-sized poly (amidoamine) dendrimer conjugated with platinum prodrug (PAMAM-Pt). At pH 7.4, the nanocarriers had a stable structure with a dimension of 130 nm, which is beneficial for the circulation of blood and extravasation of tumor cells. In response to the acidic tumor microenvironment, the nanocarriers can be dissociated into HMSN@GEM-CS and PAMAM-Pt dendrimer nanocarriers. Then the HMSN@GEM exhibited antitumor properties in surface tumor tissue. Due to its small size and positive charge, the dissociated PAMAM-Pt is highly effective in tumor penetration, cellular uptake and intracellular trafficking. Finally, the active cisplatin released from PAMAM-Pt dendrimer kills deep tumor cells.206
The hierarchically stimuli-responsive approach provides a simple and highly applicable approach for overcoming drug delivery barriers and has promising future clinical applications.
4.2 Redox/light dual-responsive drug delivery system
The release kinetics of platinum-loaded drug nanocarriers controlled by light and reduction environment has also been widely concerned. For example, supramolecular self-assembled nanoparticles (SMSANPs) loading Pt(IV) complexes were constructed with photosensitizers. Photosensitizers can be used to produce ROS upon irradiation by light. Host–guest interactions of porphyrin and Pt(IV) prodrug bridged β-CD dimer structured a redox/light dual stimuli-responsive nanoparticle (Fig. 30). Porphyrin is a photo sensitizer to produce ROS. Pt(IV) prodrug was reduced to produce the active Pt(II) species in the presence of natural reductants. The nanoparticles showed higher cytotoxicity than cisplatin to A549R cells and the cytotoxicity substantially increased under light irradiation.207
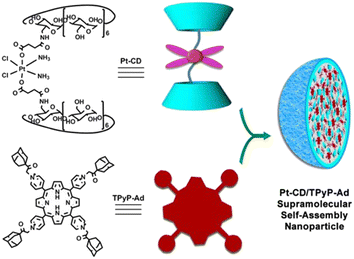 |
| Fig. 30 SMSANP-based redox/light dual-responsive drug delivery system.207 Host–guest interactions of porphyrin and Pt(IV) prodrug bridged β-CD dimer. | |
Pt(IV) prodrug and RB as photosensitizers were used to construct redox/light dual stimuli-responsive nanoparticles via covalent loading on UCNPs (Fig. 31). The maximum absorption wavelength of RB matches perfectly with the emission from UCNPs and then RB can generate ROS through using the visible light emitted by UCNPs. As a result, the nanoparticles showed a decreased IC50 upon irradiation at 808 nm against both cisplatin-sensitive and -resistant cancer cells as compared with free cisplatin or RB loaded UCNPs.208
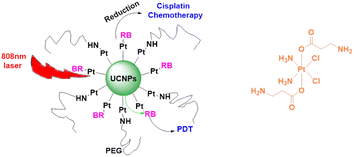 |
| Fig. 31 UCNP-based redox/light dual-responsive drug delivery system. | |
A reduction/light dual-responsive nanoconjugate (PEG-NGO-Pt) was constructed by integrating a Pt(IV) prodrug with PEGylated graphene oxide nanoparticles (Fig. 32). Graphene oxide nanoparticles with high NIR-optical absorption and specific surface area have been widely used in drug delivery and photothermal therapy. The nanoparticles could release active Pt(II) species through reducing Pt(IV) prodrug in the presence of GSH and the release rate could be easily accelerated upon NIR irradiation. As a result, the nanoconjugate showed significant cytotoxicity under irradiation against 4T1 cells as compared to the Pt(IV) parent compound.209
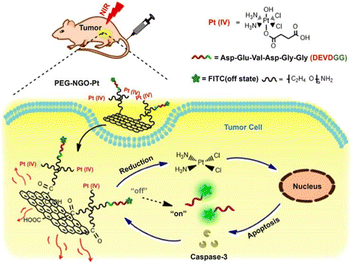 |
| Fig. 32 NGO-based redox/light dual-responsive drug delivery system.209 | |
Folate and cRGD dual modified nanoparticles based on a NIR light and glutathione dual stimuli-responsive release system was reported. The prepared nanoparticles showed a stable uniform spherical morphology and the encapsulated cisplatin was rapidly released in an acidic environment especially under added GSH and NIR irradiation. Moreover, the prepared nanoparticles can be efficiently internalized by tumor cells through the enhanced dual targeted ligands (folate and cRGD) for ICG imaging.210
The multifunctional nanoplatform UCNPs-Pt(IV)-ZnFe2O4 was designed for collaborative cancer treatment. In the system, the UCNPs-Pt(IV)-ZnFe2O4 triggered by near-infrared light can generate high energy photons, which act as the UV-vis source to simultaneously mediate the Fenton's reaction and PDT effect of ZnFe2O4 nanoparticles. Additionally, glutathione in the cancer cells can reduce Pt(IV) prodrugs into highly virulent Pt(II), which can bond to DNA and inhibit the copy of DNA. In vitro and in vivo results confirm the synergistic therapeutic effect.211
4.3 pH/thermal dual-responsive drug delivery system
Improved Pt-based drug-release activation by dual responsiveness to pH and temperature has been shown for multiple nanomaterials. For example, a pH-thermal dual responsive nanoplatform was applied for cisplatin delivery. The nanoplatform was constructed by cisplatin loading into the nanoplatform via conjugation with the carboxyl groups in the nanoplatform and introducing a thermal-responsive unit N-isopropyl acrylamide (NIPAM) into the nanogel structure. The results indicated that H+ attack and temperature rise accelerated cisplatin release from the NIPAM containing nanogels. The cisplatin-loaded nanogels exhibited better anticancer performance and longer circulation time than free cisplatin. Furthermore, these nanogels have also reduced some side effects of cisplatin, such as renal toxicity, phlebitis, bone marrow suppression, etc.212
In a study by Kurd et al., a dual pH/thermal-responsive composite nanoparticle, poly(n-isopropyl acrylamide-methacrylic acid-hydroxy ethyl methacrylate) loading Fe3O4 MNPs and cisplatin, was synthesized. High level of drug release under the conditions of low pH and high temperature was observed. This result suggests that the dual pH/temperature-responsive P(NIPAAM-MAA-HEM) magnetic nanocomposite can be very effective in hyperthermia and controlled cisplatin delivery.213
pH and thermo dual stimuli-responsive nanoparticles for targeted delivery of Pt-based antitumor agents were also built by platinum-acridine agents and cyclic (Arg-Gly-Asp-D-Phe-Lys) (cRGD) peptide modified porphyrin liposomes. Platinum-acridine agents, a dual binding mode involving intercalation and monofunctional platination of nucleobase nitrogen, were encapsulated into liposomes. The cRGD peptide was a targeted ligand. It was found that platinum drug release was steerable and fast under low pH and high temperature, and displayed significant inhibitory effects in cancer cells.214
4.4 Light/magnetic dual-responsive drug delivery system
High-intensity chemo-radiotherapies are the main methods to treat tumors at present. Simultaneously, followed with undesirable drug specific and radiation therapy-incurred side effects, combinatorial therapeutic approaches consisting of various nanostructures and advanced instrumentation are becoming one of the most exciting forefront fields to solve the problem. Induced hyperthermia or photothermal destruction of cancer cells by oscillating magnetic fields is another method that is of concern. A combinatorial therapeutic strategy involving simultaneous light and oscillating magnetic field stimulation of a multifunctional nanocarrier system has successfully been implemented to achieve complete ablation of neuroblastoma cells in culture (Fig. 33). This novel photo-magnetic dual-responsive nanocarrier was constructed with cisplatin loading on a double-layered shell thermo-activated polymer network. The combination of released cisplatin from nanocarriers and photo-magnetic coupled hyperthermia mediated cytotoxicity led to the complete apoptosis of neuroblastoma cells in culture. Although this photo-magnetic dual-responsive drug delivery system is a viable approach to increase the efficacy of Pt-based drugs to neuroblastoma cells, few studies have been conducted in this regard to date.215
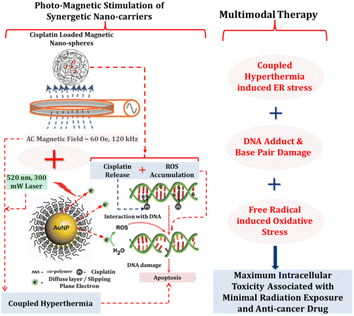 |
| Fig. 33 Photo-magnetic dual-responsive drug delivery system. The photo-magnetic dual-responsive nanocarrier was constructed with cisplatin loading on a double-layered shell thermo-activated polymer network.215 | |
Improved Pt-based drug-release activation by dual responsiveness to light and pH has been shown for polymeric nanoparticles.172,216 Other systems have shown response to ultrasound and magnetic field for Pt-based drug delivery.217
4.5 Triple-responsive drug delivery system
Development of triple stimuli-responsive drug delivery systems is promising for tumor therapy under tumor microenvironments. A variety of nanocarriers have been reported for triple stimuli-responsive release of antitumor drugs, for example, pH/thermal/redox triple-responsive nanogels,218 periodic mesoporous organosilica nanoparticles,219 dendrimeric nanocage system,187,220 Ca2+/pH/thermal triple-responsive Zr-based MOF system,221 light/ultrasound/enzyme triple-responsive inorganic–organic hybrid microcapsules,222 ionic strength/pH/enzyme triple-responsive zwitterionic hydrogel,223 magnetic/redox/thermal triple-responsive nanocarriers based on modified core–shell magnetic mesoporous silica nanoparticles,224 photo/pH/redox triple-responsive nanogels,225 thermal/pH/enzyme triple-responsive nanogels,226 thermo/pH/magnetic triple-responsive nanogels,227,228 pH/enzyme/light triple-responsive magnetic hollow porous carbon-based nanodrug delivery system,229 and pH/ROS/protein triple-responsive block copolymer,230 but few studies have been conducted on the application of Pt-based antitumor drugs. Liu Zhuang et al. reported a multistage responsive cancer theranostic nanoplatform BM@NCP (DSP)-PEG (Fig. 34). This multistage responsive system shows efficient tumor homing as well as rapid renal excretion, as illustrated by magnetic resonance imaging and confirmed by biodistribution measurement. Notably, an excellent in vivo tumor growth inhibition effect is observed with BM@NCP (DSP)-PEG nanoparticles after the combined chemoradiotherapy treatment.231
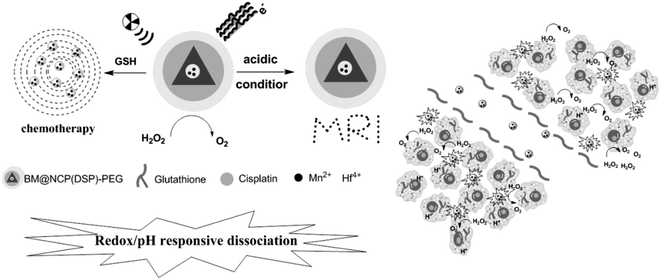 |
| Fig. 34 Multistage redox/pH/H2O2-responsive drug delivery system. | |
5 Conclusions and future outlook
Pt-based anticancer drugs play a crucial role in the clinical treatment of various cancers. However, the application of Pt-based drugs is heavily restricted by their severe toxicity and drug resistance/cross resistance. Development of drug-delivery systems that selectively target neoplastic cells has been a major goal of the future development of platinum antitumor drugs. Based on the complex tumor microenvironment and advances in decoration techniques for nanocarriers, a large quantity of nanocarriers have been shown to have the capacity for being modified with ligands on their surface or encapsulating drugs in their core, allowing for controlled release by a variety of triggers including endogenous and extracorporeal stimuli. These features have made stimuli-responsive nanocarrier delivery strategies one of the most promising strategies for platinum drugs. In this review, the diversiform responsive nanomaterials that can be assembled in different architectures and the multitudinous exogenous and endogenous stimuli to trigger the Pt-based drug release were summarized. This smart drug delivery system of Pt-based antitumor drugs is regarded as an excellent device to maximize the anticancer efficacy and minimize the limitations since anticancer agents can be concentrated in tumor tissue rather than in normal tissues or organs. Although a lot of research has been made in stimuli-responsive nanocarrier delivery systems of Pt-based antitumor drugs, only a few have been tested in in vivo preclinical test, and very few have reached the clinical stage. Therefore, improvements in smart nanocarrier technology and new advances in triggering Pt-based drugs should now be given more attention to obtain clinically acceptable systems.
The ideal tumor therapy should not only accurately destroy the primary tumor, but also improve the immunogenicity of the tumor microenvironment. Metal immunotherapy is one of the important unsolved scientific problems in the field of metal chemical biology. Recent advances in metal immunotherapy for cancer have received widespread attention. Looking into the clinical application of platinum drugs in chemotherapy and metal immunotherapy, companion immunosuppressive mechanism of platinum antitumor drugs will contribute to the development of stimuli-sensitive nanocarrier drug delivery systems towards the direction of targeted immunity. On the other hand, the strategy of targeted stimulus-responsive drug delivery system and diagnostic strategies of the biomarker expression in the tumor microenvironment can help this system develop integrated precision therapy. A broad range of tumor therapies may benefit from the strategy of assembling biomarkers of cancer biology and antineoplastic drugs into stimulus-responsive nanocarriers.
List of abbreviations
CDDP | Cisplatin |
DOPE | Dioleoylphosphatidylethanolamine |
CH | Cholesterol |
DMPC | Dimyristoylphosphatidylcholine |
DMPG | Dimyristoylphosphatidylglycerol |
DPPG | Dipalmitoylphosphatidylglycerol |
PE | Phosphatidylethanolamine |
CHEMS | Cholesteryl hemisuccinate |
DSPE-PEG2000 | Distearoylphosphatidylethanolaminepolyethyleneglycol2000 |
HSPC | Hydrogenated soy phosphatidylcholine |
MSPC | Monostearoyl-phosphatidylcholine |
Uns-lipid | Unsaturated lipid similar to HSPC |
HPMA | Hydroxypropylmethacrylamide |
CS | Chondroitin sulfate |
PCN | Polymer-caged nanobin |
Ac-Dex | Acetalated dextran |
GALA | Glutamic acid–alanine–leucine–alanine |
KALA | Lysine–alanine–leucine–alanine |
Mal-PEG114-b-PLG12 | Maleimide-poly(ethyleneoxide)114-b-poly(L-glutamic acid)12 |
DACHPt | Diaminocyclohexyl platinum |
PAMAM | Poly(amidoamine) |
MSNPs | Mesoporous silica nanoparticles |
MOFs | Metal–organic frameworks |
NCPs | Nanoscale coordination polymers |
PAA | Polyacrylic acid |
MR | Magnetic resonance |
SPION | Superparamagnetic iron oxide nanoparticles |
CMDP | Dechlorinated cisplatin |
HA | Hyaluronic acid |
GSH | Glutathione |
BPEI | Branched polyethyleneimine |
CNTs | Carbon nanotubes |
MSNs | Mesoporous silica nanoparticles |
MMP9 | Matrix metalloproteinase 9 |
PCL | Polycaprolactone |
DEGNPs | Dendrimer-encapsulated gold nanoparticles |
NP | Nanoparticle |
CPN | Chitosan |
DOX | Doxorubicin |
MTX | Methotrexate |
SWCNTs | Single-walled carbon nanotubes |
RF | Radio frequency |
LFUS | Low frequency ultrasound |
HFUS | High frequency ultrasound |
UV | Ultraviolet |
NIR | Near infrared |
UCNPs | Upconversion nanoparticles |
PSs | Photosensitizers |
RB | Rose bengal |
PDT | Photodynamic therapy |
UCL | Upconversion luminescence |
MR | Magnetic resonance |
CT | Computer tomography |
ICG | Indocyanine green |
RBC | Red blood cell |
BSA | Bovine serum albumin |
QDs | Quantum dots |
HGNPs | Hollow gold nanoparticles |
AMF | Alternating magnetic field |
HSA | Human serum albumin |
TN | Theranostic nanoplatforms |
TAT | Transactivator of transcription |
HMSNs | Hollow mesoporous silica nanoparticles |
GEM | Gemcitabine |
SMSANPs | Supramolecular self-assembled nanoparticles |
ROS | Reactive oxygen species |
NIPAM | N-Isopropyl acrylamide |
PEG | Polyethylene glycol |
HF | Hafnium |
Author contributions
Tianshuai Wang and Yan Zhang were involved in conceptualization and design of the work; Tianshuai Wang, Yan Zhang and Chen Wu were involved in the acquisition, analysis, or interpretation of data; Yan Zhang were involved in the creation of new software used in the work; Tianshuai Wang and Chen Wu were involved in writing-original draft preparation; Junkai Ma and Yanggen Hu were involved in writing-review and editing.
Conflicts of interest
There are no conflicts to declare.
Acknowledgements
This work is supported by the Natural Science Foundation of Hubei Province (2019CFC838) and the Key projects of Hubei Provincial Department of Education (D20192102) and the Hubei Provincial Education Department Research Fund (B202116), Key Project of Hubei Science and Technology Department (2022BGE260), which are gratefully acknowledged.
References
- S. Ghosh, Bioorg. Chem., 2019, 88, 102925 CrossRef CAS PubMed.
- L. K. Bai, C. Z. Gao and Q. H. Liu, et al., Eur. J. Med. Chem., 2017, 140, 349–382 CrossRef CAS PubMed.
- Y. Zhong, C. Jia and X. Zhang, et al., Eur. J. Med. Chem., 2020, 194, 112229 CrossRef CAS PubMed.
- X. Y. Wang and Z. J. Guo, Chem. Soc. Rev., 2013, 42, 202–224 RSC.
- R. J. Browning, P. J. T. Reardon and M. Parhizkar, et al., ACS Nano, 2017, 11, 8560–8578 CrossRef CAS PubMed.
- P. A. Ma, H. H. Xiao and C. X. Li, et al., Mater. Today, 2015, 18, 554–564 CrossRef CAS.
- M. A. Farooq, M. Aquib and A. Farooq, et al., Artif. Cells, Nanomed., Biotechnol., 2019, 47, 1674–1692 CrossRef CAS PubMed.
- A. M. Pisoschi, A. Pop and C. Cimpeanu, et al., Eur. J. Med. Chem., 2018, 157, 1326–1345 CrossRef CAS PubMed.
- C. Z. Gao, Y. Zhang and J. Chen, et al., Mini-Rev. Med. Chem., 2016, 16, 872–891 CrossRef CAS PubMed.
- H. S. Oberoi, N. V. Nukolova and A. V. Kabanov, et al., Adv. Drug Delivery Rev., 2013, 65, 1667–1685 CrossRef CAS PubMed.
- S. Hossen, M. K. Hossain and M. K. Basher, et al., J. Adv. Res., 2019, 15, 1–18 CrossRef CAS PubMed.
- J. Ma, X. Yang and W. Hao, et al., Eur. J. Med. Chem., 2017, 128, 45–55 CrossRef CAS PubMed.
- X. H. Wang, X. Y. Wang and S. X. Jin, et al., Chem. Rev., 2019, 119, 1138–1192 CrossRef CAS PubMed.
- M. Y. Li, C. Y. Du and N. Guo, et al., Eur. J. Med. Chem., 2019, 164, 640–653 CrossRef CAS PubMed.
- A. A. Gabizon, J. Drug Targeting, 2002, 10, 535–538 CrossRef CAS PubMed.
- M. Li, C. Du and N. Guo, et al., Eur. J. Med. Chem., 2019, 164, 640–653 CrossRef CAS PubMed.
- W. D. Wu, X. L. Yi and L. X. Jiang, et al., Curr. Drug Metab., 2015, 16, 894–910 CrossRef CAS PubMed.
- E. S. Kim, C. Lu and F. R. Khuri, et al., Lung Cancer, 2001, 34, 427–432 CrossRef CAS PubMed.
- G. P. Stathopoulos and T. Boulikas, J. Drug Delivery, 2012, 2012, 581363 CAS.
- S. Zalba and M. J. Garrido, Expert Opin. Drug Delivery, 2013, 10, 829–844 CrossRef CAS PubMed.
- M. C. De Oliveira, E. Fattal and P. Couvreur, et al., Biochim. Biophys. Acta, 1998, 1372, 301–310 CrossRef CAS PubMed.
- M. Kanamala, B. D. Palmer and S. M. F. Jamieson, et al., Nanomedicine, 2019, 14, 1972–1990 CrossRef PubMed.
- T. Thambi, J. H. Park and D. S. Lee, Biomater. Sci., 2016, 4, 55–69 RSC.
- H. H. Xiao, L. S. Yan and E. M. Dempsey, et al., Prog. Polym. Sci., 2018, 87, 70–106 CrossRef CAS.
- X. L. Hu, Y. G. Zhang and Z. G. Xie, et al., Biomacromolecules, 2017, 18, 649–673 CrossRef CAS PubMed.
- Q. Tang, B. Yu and L. L. Gao, et al., Curr. Med. Chem., 2018, 25, 1837–1866 CrossRef CAS PubMed.
- X. Pang, Y. Jiang and Q. C. Xiao, et al., J. Controlled Release, 2016, 222, 116–129 CrossRef CAS PubMed.
- D. P. Nowotnik and E. Cvitkovic, Adv. Drug Delivery Rev., 2009, 61, 1214–1219 CrossRef CAS PubMed.
- A. Paraskar, S. Soni and S. Basu, et al., Nanotechnology, 2011, 22, 265101 CrossRef PubMed.
- N. Alam, M. Koul and M. J. Mintoo, et al., Biomed. Pharmacother., 2017, 95, 856–864 CrossRef CAS PubMed.
- J. Y. Wang, Y. Wang and X. Meng, Nanoscale Res. Let., 2016, 11, 524 CrossRef PubMed.
- M. S. Gil, T. Thambi and V. H. G. Phan, et al., J. Mater. Chem. B, 2017, 5, 7140–7152 RSC.
- S. M. Lee, T. V. O'Halloran and S. T. Nguyen, J. Am. Chem. Soc., 2010, 132, 17130–17138 CrossRef CAS PubMed.
- E. M. Bachelder, E. N. Pino and K. M. Ainslie, Chem. Rev., 2017, 117, 1915–1926 CrossRef CAS PubMed.
- M. A. Collier, R. D. Junkins and M. D. Gallovic, et al., Mol. Pharm., 2018, 15, 4933–4946 CrossRef CAS PubMed.
- C. B. Braga, G. Perli and T. B. Becher, et al., Mol. Pharmacol., 2019, 16, 2083–2094 CrossRef CAS PubMed.
- J. Huang and A. Heise, Chem. Soc. Rev., 2013, 42, 7373–7390 RSC.
- J. V. John, R. P. Johnson and M. S. Heo, et al., J. Biomed. Nanotechnol., 2015, 11, 1–39 CrossRef CAS PubMed.
- P. Zhang, M. Li and C. Xiao, et al., Chem. Commun., 2021, 57, 9489–9503 RSC.
- S. J. Shirbin, K. Ladewig and Q. Fu, et al., Biomacromolecules, 2015, 16, 2463–2474 CrossRef CAS PubMed.
- L. S. Yan and X. D. Li, Curr. Pharm. Biotechnol., 2016, 17, 227–236 CAS.
- Y. M. Li, A. H. Yu and L. B. Li, et al., J. Drug Targeting, 2018, 26, 753–765 CrossRef CAS PubMed.
- Y. Dai, X. Chen and X. Zhang, Polym. Chem., 2019, 10, 34–44 RSC.
- M. Shahin and N. Safaei-Nikouei, J. Drug Targeting, 2014, 22, 629–637 CrossRef CAS PubMed.
- H. Uchino, Y. Matsumura and T. Negishi, et al., Br. J. Cancer, 2005, 93, 678–687 CrossRef CAS PubMed.
- S. Binauld, W. Scarano and M. H. Stenzel, Macromolecules, 2012, 45, 6989–6999 CrossRef CAS.
- H. S. Oberoi, F. C. Laquer and L. A. Marky, et al., J. Controlled Release, 2011, 153, 64–72 CrossRef CAS PubMed.
- C. Kojima, Drug Delivery, 2010, 7, 307–319 CAS.
- H. Wang, Q. Huang and H. Chang, et al., Biomater. Sci., 2016, 4, 375–390 RSC.
- Y. H. Cheng, C. L. He and J. E. Riviere, et al., ACS Nano, 2020, 14, 3075–3095 CrossRef CAS PubMed.
- D. Pan, W. She and C. Guo, et al., Biomaterials, 2014, 35, 10080–10092 CrossRef CAS PubMed.
- Y. M. Xia, Y. M. Wang and Y. P. Wang, et al., Colloids Surf., B, 2011, 88, 674–681 CrossRef CAS PubMed.
- H. J. Li, J. Z. Du and X. J. Du, et al., Proc. Natl. Acad. Sci. U. S. A., 2016, 113, 4164–4169 CrossRef CAS PubMed.
- J. L Vivero-Escoto, I. I. Slowing and B. G. Trewyn, et al., Small, 2010, 6, 1952–1967 CrossRef PubMed.
- Y. Alyassin, E. G. Sayed and P. Mehta, et al., Drug Discovery Today, 2020, 25, 1513–1520 CrossRef CAS PubMed.
- J. L. Gu, J. P. Liu and Y. S. Li, et al., Langmuir, 2013, 29, 403–410 CrossRef CAS PubMed.
- H. He, H. Xiao and H. Kuang, et al., Colloids Surf., B, 2014, 117, 75–81 CrossRef CAS PubMed.
- C. H. Lin, S. H. Cheng and W. N. Liao, et al., Int. J. Pharm., 2012, 429, 138–147 CrossRef CAS PubMed.
- W. Cai, J. Q. Wang and C. C. Chu, et al., Adv. Sci., 2019, 6, 1801526 CrossRef PubMed.
- X. Shen, Y. Pan and Z. H. Sun, et al., Mini-Rev. Med. Chem., 2019, 19, 1644–1665 CrossRef CAS PubMed.
- C. He, K. Lu and D. Liu, et al., J. Am. Chem. Soc., 2014, 136, 5181–5184 CrossRef CAS PubMed.
- M. Ibrahim, R. Sabouni and G. A. Husseini, Curr. Med. Chem., 2017, 24, 193–214 CrossRef CAS PubMed.
- S. X. Lin, W. L. Pan and R. J. Niu, et al., Dalton Trans., 2019, 48, 5308–5314 RSC.
- Y. J. Li, Z. G. Gao and F. H. Chen, et al., ACS Appl. Mater. Interfaces, 2018, 10, 30930–30935 CrossRef CAS PubMed.
- Y. Liu, S. Lv and D. Liu, et al., Acta Biomater., 2020, 116, 16–31 CrossRef CAS PubMed.
- K. Han, W. Y. Zhang and J. Zhang, et al., Adv. Healthcare Mater., 2017, 6, 1700470 CrossRef PubMed.
- W. J. Rieter, K. M. Pott and K. M. L. Taylor, et al., J. Am. Chem. Soc., 2008, 130, 11584–11585 CrossRef CAS PubMed.
- T. Vangijzegem, D. Stanicki and S. Laurent, Drug Delivery, 2019, 16, 69–78 CAS.
- J. Kallu, T. Banerjee and S. Sulthana, et al., Nanotheranostics, 2019, 3, 120–134 CrossRef PubMed.
- C. Janko, T. Ratschker and K. Nguyen, et al., Front. Oncol., 2019, 9, 59 CrossRef PubMed.
- R. M. Xing, X. Y. Wang and C. L. Zhang, et al., J. Mater. Chem., 2011, 21, 11142–11149 RSC.
- N. Alegret, A. Criado and M. Prato, Curr. Med. Chem., 2017, 24, 529–536 CrossRef CAS PubMed.
- R. O. Rodrigues, G. Baldi and S. Doumett, et al., Mater. Sci. Eng., C, 2018, 9, 206–217 CrossRef PubMed.
- M. S. Moorthy, G. Hoang and P. Manivasagan, et al., J. Porous Mater., 2019, 26, 217–226 CrossRef CAS.
- Y. F. Yang, F. Y. Meng and X. H. Li, et al., J. Nanosci. Nanotechnol., 2019, 19, 7517–7525 CrossRef CAS PubMed.
- Y. Mi, J. Wolfram and C. F. Mu, et al., Pharmacol. Res., 2016, 113, 92–99 CrossRef CAS PubMed.
- A. C. Hortelao, T. Patino and A. Perez-Jimenez, et al., Adv. Funct. Mater., 2018, 28, 1705086 CrossRef.
- M. Shahriari, M. Zahiri and K. Abnous, et al., J. Controlled Release, 2019, 308, 172–189 CrossRef CAS PubMed.
- C. E. Callmann, C. V. Barback and M. P. Thompson, et al., Adv. Mater., 2015, 27, 4611–4615 CrossRef CAS PubMed.
- T. R. Kuang, Y. R. Liu and T. T. Gong, et al., Curr. Nanosci., 2016, 12, 38–46 CrossRef CAS.
- Q. Zhou, L. Zhang and T. H. Yang, et al., Int. J. Nanomed., 2018, 13, 2921–2942 CrossRef CAS PubMed.
- Y. I. Jeong, S. T. Kim and S. G. Jin, et al., J. Pharm. Sci., 2008, 97, 1268–1276 CrossRef CAS PubMed.
- Z. Z. Zhu, Z. H. Wang and Y. G. Hao, et al., Chem. Sci., 2016, 7, 2864–2869 RSC.
- J. Li, S. L. Yoong and W. J. Goh, et al., Int. J. Nanomed., 2015, 10, 7425–7441 CAS.
- J. K. Kim, J. Anderson and H. W. Jun, et al., Mol. Pharm., 2009, 6, 978–985 CrossRef CAS PubMed.
- J. M. Rosenholm, A. Duchanoy and M. Linden, Chem. Mater., 2008, 20, 1126–1133 CrossRef CAS.
- S. H. van Rijt, D. A. Bolukbas and C. Argyo, et al., ACS Nano, 2015, 9, 2377–2389 CrossRef CAS PubMed.
- J. R. Tauro and R. A. Gemeinhart, Bioconjugate Chem., 2005, 16, 1133–1139 CrossRef CAS PubMed.
- B. Surnar, K. Sharma and M. Jayakannan, Nanoscale, 2015, 7, 17964–17979 RSC.
- X. Wang, X. Cai and J. Hu, et al., J. Am. Chem. Soc., 2013, 135, 9805–9810 CrossRef CAS PubMed.
- W. Cao, Y. W. Gu and M. Meineck, et al., J. Am. Chem. Soc., 2014, 136, 5132–5137 CrossRef CAS PubMed.
- W. Cao, Y. Li and Y. Yi, et al., Chem. Sci., 2012, 3, 3403–3408 RSC.
- W. Q. Zhang and C. H. Tung, Chem. Commun., 2018, 54, 8367–8370 RSC.
- J. P. Xia, Y. N. Du and L. P. Huang, et al., Nanomedicine, 2018, 14, 713–723 CrossRef CAS PubMed.
- S. J. T. Rezaei, V. Amani and M. R. Nabid, et al., Polym. Chem., 2015, 6, 2986 RSC.
- Y. Y. Jia, J. J. Zhang and Y. X. Zhang, et al., Int. J. Pharm., 2020, 580, 119190 CrossRef CAS PubMed.
- J. Della Rocca, M. E. Werner and S. A. Kramer, et al., Nanomedicine, 2015, 11, 31–38 CrossRef PubMed.
- Y. Han, J. J. Li and M. H. Zan, et al., Polym. Chem., 2014, 5, 3707–3718 RSC.
- X. Ling, J. S. Tu and J. Q. Wang, et al., ACS Nano, 2019, 13, 357–370 CrossRef CAS PubMed.
- M. Kakehi, K. Ueda and T. Mukojima, et al., Int. J. Hyperthermia, 1990, 6, 719–740 CrossRef CAS PubMed.
- O. L. Cremer and C. J. Kalkman, Prog. Brain Res., 2007, 162, 153–169 Search PubMed.
- K. Ahmed, Y. Tabuchi and T. Kondo, Apoptosis, 2015, 20, 1411–1419 CrossRef CAS PubMed.
- M. F. Gnant, L. A. Noll and R. E. Terrill, et al., Surgery, 1999, 126, 890–899 CrossRef CAS PubMed.
- A. Sohail, Z. Ahmad and O. A. Beg, et al., Bull. Cancer, 2017, 104, 452–461 CrossRef PubMed.
- M. Sponchioni, U. Capasso Palmiero and D. Moscatelli, Mater. Sci. Eng., C, 2019, 102, 589–605 CrossRef CAS PubMed.
- P. Zarrintaj, M. Jouyandeh and M. R. Ganjali, et al., Eur. Polym. J., 2019, 117, 402–423 CrossRef CAS.
- J. Y. Fang, J. P. Chen and Y. L. Leu, et al., Drug Delivery, 2008, 15, 235–243 CrossRef CAS PubMed.
- M. J. Moura, M. H. Gil and M. M. Figueiredo, Eur. Polym. J., 2013, 49, 2504–2510 CrossRef CAS.
- H. C. Ma, C. L. He and Y. L. Cheng, et al., ACS Appl. Mater. Interfaces, 2015, 7, 27040–27048 CrossRef CAS PubMed.
- Z. H. Han, L. W. Lv and Y. Ma, et al., J. Biophotonics, 2017, 10, 1607–1616 CrossRef CAS PubMed.
- S. Alidori, D. L. J. Thorek and B. J. Beattie, et al., PLoS One, 2017, 12, e0183902 CrossRef PubMed.
- T. Murakami, Chem. Pharm. Bull., 2017, 65, 629–636 CrossRef CAS PubMed.
- Q. R. Cao, X. X. Zhang and H. Y. Huang, et al., J. Pharm. Invest., 2019, 49, 57–65 CrossRef.
- M. Anas, Y. Zhao and M. A. Saed, et al., Nanoscale, 2019, 11, 9617–9625 RSC.
- M. Raoof, B. T. Cisneros and A. Guven, et al., Biomaterials, 2013, 34, 1862–1869 CrossRef CAS PubMed.
- Y. Z. Zhao, L. N. Du and C. T. Lu, et al., Int. J. Nanomed., 2013, 8, 1621–1633 Search PubMed.
- X. Zhou, L. Guo and D. Shi, et al., Drug Delivery, 2020, 27, 469–481 CrossRef CAS PubMed.
- J. A. Oshiro-Junior, C. Rodero and G. Hanck-Silva, et al., Curr. Med. Chem., 2020, 27, 2494–2513 CrossRef CAS PubMed.
- W. X. Sun, H. T. Jiang and X. Wu, et al., Nano Res., 2019, 12, 115–119 CrossRef CAS.
- G. B. Yang, J. J. Liu and Y. F. Wu, et al., Coord. Chem. Rev., 2016, 320, 100–117 CrossRef.
- S. G. Zheng, H. X. Xu and H. R. Chen, World Journal of Radiology, 2013, 5, 468–471 CrossRef PubMed.
- A. Schroeder, R. Honen and K. Turjeman, et al., J. Controlled Release, 2009, 137, 63–68 CrossRef CAS PubMed.
- A. Kheirolomoom, L. M. Mahakian and C. Y. Lai, et al., Mol. Pharm., 2010, 7, 1948–1958 CrossRef CAS PubMed.
- W. G. Pitt, G. A. Husseini and B. J. Staples, Expert Opin. Drug Delivery, 2004, 1, 37–56 CrossRef CAS PubMed.
- S. Mitragotri and J. Kost, Adv. Drug Delivery Rev., 2004, 56, 589–601 CrossRef CAS PubMed.
- N. Rapoport, A. I. Smirnov and A. Timoshin, et al., Arch. Biochem. Biophys., 1997, 344, 114–124 CrossRef CAS PubMed.
- M. Duvshani-Eshet, L. Baruch and E. Kesselman, et al., Gene Ther., 2006, 13, 163–172 CrossRef CAS PubMed.
- J. Sundaram, B. R. Mellein and S. Mitragotri, Biophys. J., 2003, 84, 3087–3101 CrossRef CAS PubMed.
- A. Schroeder, Y. Avnir and S. Weisman, et al., Langmuir, 2007, 23, 4019–4025 CrossRef CAS PubMed.
- Y. N. Dou, R. A. Weersink and W. D. Foltz, et al., J. Visualized Exp., 2015, 106, e53055 Search PubMed.
- Y. Watanabe, A. Aoi and S. Horie, et al., Cancer Sci., 2008, 99, 2525–2531 CrossRef CAS PubMed.
- N. Sasaki, N. Kudo and K. Nakamura, et al., J. Med. Ultrason., 2014, 41, 11–21 CrossRef PubMed.
- C. H. Heath, A. Sorace and J. Knowles, et al., Otolaryngol.--Head Neck Surg., 2012, 146, 938–945 CrossRef PubMed.
- N. Sasaki, N. Kudo and K. Nakamura, et al., Ultrasound Med. Biol., 2012, 38, 109–118 CrossRef PubMed.
- N. Y. Rapoport, A. M. Kennedy and J. E. Shea, et al., J. Controlled Release, 2009, 138, 268–276 CrossRef CAS PubMed.
- C. R. Hill and G. R. ter Haar, Br. J. Radiol., 1995, 68, 1296–1303 CrossRef CAS PubMed.
- C. Oerlemans, R. Deckers and G. Storm, et al., J. Controlled Release, 2013, 168, 327–333 CrossRef CAS PubMed.
- R. Deckers, A. Paradissis and C. Oerlemans, et al., Langmuir, 2013, 29, 9483–9490 CrossRef CAS PubMed.
- T. Bian, Z. L. Chu and R. Klajn, Adv. Mater., 2020, 32, e1905866 CrossRef PubMed.
- Y. J. Yu, Q. Xu and S. S. He, et al., Coord. Chem. Rev., 2019, 387, 154–179 CrossRef CAS.
- W. Zhao, Y. Zhao and Q. Wang, et al., Small, 2019, 15, e1903060 CrossRef PubMed.
- J. Xiang, X. Tong and F. Shi, et al., J. Mater. Chem. B, 2018, 6, 3531–3540 RSC.
- C. Yao, P. Wang and X. Li, et al., Adv. Mater., 2016, 28, 9341–9348 CrossRef CAS PubMed.
- Z. Dai and Z. Wang, Molecules, 2020, 25, 5167 CrossRef CAS PubMed.
- P. J. Bednarski, F. S. Mackay and P. J. Sadler, Adv. Anticancer Agents Med. Chem., 2007, 7, 75–93 CrossRef CAS PubMed.
- A. Najjar, N. Rajabi and R. Karaman, Curr. Pharm. Des., 2017, 23, 2366–2376 CrossRef CAS PubMed.
- S. Pramanick, J. Kim and J. Kim, et al., Bioconjugate Chem., 2018, 29, 885–897 CrossRef CAS PubMed.
- J. Zhou, Z. Liu and F. Li, Chem. Soc. Rev., 2012, 41, 1323–1349 RSC.
- N. M. Idris, M. K. Gnanasammandhan and J. Zhang, et al., Nat. Med., 2012, 18, U1580–U1190 CrossRef PubMed.
- X. Wang, C. Wang and Q. Zhang, et al., Chem. Commun., 2016, 52, 978–981 RSC.
- H. Y. Wang, H. Zhang and S. Chen, et al., Curr. Med. Chem., 2019, 26, 4029–4041 CrossRef CAS PubMed.
- Y. Zhang, F. Zheng and T. Yang, et al. T, Nat. Mater., 2012, 11, 817–826 CrossRef CAS PubMed.
- J. A. Liu, W. B. Bu and L. M. Pan, et al., Angew. Chem., Int. Ed. Engl., 2013, 52, 4375–4379 CrossRef CAS PubMed.
- E. Ruggiero, J. Hernández-Gil and J. C. Mareque-Rivas, et al., Chem. Commun., 2016, 52, 9299 RSC.
- F. Ai, T. Sun and Z. Xu, et al., Dalton Trans., 2016, 45, 13052–13060 RSC.
- Y. L. Dai, H. H. Xiao and J. H. Liu, et al., J. Am. Chem. Soc., 2013, 135, 18920–18929 CrossRef CAS PubMed.
- Y. L. Dai, H. T. Bi and X. R. Deng, et al., J. Mater. Chem. B, 2017, 5, 2086–2095 RSC.
- Y. Z. Min, J. M. Li and F. Liu, et al., Angew. Chem., Int. Ed., 2014, 53, 1012–1016 CrossRef CAS PubMed.
- F. Li, T. Y. Li and W. Cao, et al., Biomaterials, 2017, 133, 208–218 CrossRef CAS PubMed.
- M. Mir, S. Ishtiaq and S. Rabia, et al., Nanoscale Res. Lett., 2017, 12, 500 CrossRef PubMed.
- W. Liu, M. Ruan and Y. Wang, et al., Small, 2018, 14, e1801754 CrossRef PubMed.
- N. G. Blanco, C. R. Maldonado and J. C. Mareque-Rivas, Chem. Commun., 2009, 5257–5259 RSC.
- I. Infante, J. M. Azpiroz and N. G. Blanco, et al., J. Phys. Chem. C, 2014, 118, 8712–8721 CrossRef CAS.
- C. Y. Xiang, W. Lu and M. Zhou, et al., Cancer Nanotechnol., 2018, 9, 6 CrossRef PubMed.
- Y. F. Yang, F. Y. Meng and X. H. Li, et al., J. Nanosci. Nanotechnol., 2019, 19, 7517–7525 CrossRef CAS PubMed.
- N. Rabiee and M. Rabiee, Curr. Nanomater., 2019, 3, 160–167 CrossRef.
- S. Nappini, S. Fogli and B. Castroflorio, et al., J. Mater. Chem. B, 2016, 4, 716–725 RSC.
- H. Q. Song, F. F. Quan and Z. Q. Yu, et al., J. Mater. Chem. B, 2019, 7, 433–442 RSC.
- P. A. Ma, H. H. Xiao and C. Yu, et al., Nano Lett., 2017, 17, 928–937 CrossRef CAS PubMed.
- C. H. Zhao, X. B. Song and W. G. Jin, et al., Anal. Chim. Acta, 2019, 1074, 150–151 CrossRef CAS PubMed.
- M. Namdari, M. Cheraghi and B. Negahdari, et al., Artif. Cells, Nanomed., Biotechnol., 2017, 45, 1051–1057 CrossRef CAS PubMed.
- C. Q. You, H. S. Wu and M. X. Wang, et al., Chemistry, 2017, 23, 5352–5360 CrossRef CAS PubMed.
- C. Q. You, H. S. Wu and M. X. Wang, et al., Nanotechnology, 2018, 29, 015601 CrossRef PubMed.
- C. Q. You, J. Yu and Y. Sun, et al., New J. Chem., 2017, 41, 773–785 RSC.
- C. You, M. Wang and H. Wu, et al., Mater. Sci. Eng., C, 2017, 80, 362 CrossRef CAS PubMed.
- W. P. Li, C. H. Su and Y. C. Chang, et al., ACS Nano, 2016, 10, 2017–2027 CrossRef CAS PubMed.
- F. Dilnawaz, A. Singh and C. Mohanty, et al., Biomaterials, 2010, 31, 3694–3706 CrossRef CAS PubMed.
- B. S. Chang, X. Y. Sha and J. Guo, et al., J. Mater. Chem., 2011, 21, 9239–9247 RSC.
- W. H. Chen, G. F. Luo and Q. Lei, et al., Biomaterials, 2016, 76, 87–101 CrossRef CAS PubMed.
- J. L. Nyalosaso, E. Rascol and C. Pisani, et al., RSC Adv., 2016, 6, S7275–S7283 RSC.
- K. Sun, C. Q. You and S. L. Wang, et al., Nanotechnology, 2018, 29, 285302 CrossRef PubMed.
- G. Zhiguo, L. Yao-Jia and Y. Chaoqun, et al., ACS Appl. Bio Mater., 2018, 1, 270–280 CrossRef PubMed.
- G. E. Choi, M. S. Kang and Y. J. Kim, et al., J. Nanosci. Nanotechnol., 2019, 19, 675–679 CrossRef CAS PubMed.
- J. Estelrich, E. Escribano and J. Queralt, et al., Int. J. Mol. Sci., 2015, 16, 8070–8101 CrossRef CAS PubMed.
- A. Pardo, S. Y. N. Ez and Y. Pieiro, et al., ACS Appl. Mater. Interfaces, 2020, 12, 9017–9031 CrossRef CAS PubMed.
- Z. Fu and J. Xiang, Int. J. Mol. Sci., 2020, 21, 9123 CrossRef CAS PubMed.
- D. Zhong, H. Y. Wu and Y. H. Wu, et al., Nanoscale, 2019, 11, 15091–15103 RSC.
- D. M. Liu, C. B. He and A. Z. Wang, et al., Int. J. Nanomed., 2013, 8, 3309–3319 Search PubMed.
- K. J. Haxton and H. M. Burt, J. Pharm. Sci., 2009, 98, 2299–2316 CrossRef CAS PubMed.
- Z. Yu, Q. Chen and Y. Yang, et al., Chem. Commun., 2018, 54, 5369–5372 RSC.
- L. He, M. Sun and X. Cheng, et al., J. Colloid Interface Sci., 2019, 541, 30–41 CrossRef CAS PubMed.
- Y. Min, C. Mao and D. Xu, et al., Chem. Commun., 2010, 46, 8424–8426 RSC.
- Y. Z. Min, C. Q. Mao and S. M. Chen, et al., Angew. Chem., Int. Ed., 2012, 51, 6742–6747 CrossRef CAS PubMed.
- B. S. Wong, S. L. Yoong and A. Jagusiak, et al., Adv. Drug Delivery Rev., 2013, 65, 1964–2015 CrossRef CAS PubMed.
- S. Dhar, W. L. Daniel and D. A. Giljohann, et al., J. Am. Chem. Soc., 2010, 132, 17335 CrossRef CAS.
- H. Choy, C. Park and M. Yao, Clin. Cancer Res., 2008, 14, 1633–1638 CrossRef CAS PubMed.
- J. P. Quinones, J. Jokinen and S. Keinanen, et al., Eur. Polym. J., 2018, 99, 384–393 CrossRef CAS.
- H. D. Shi, Q. Q. Cheng and S. M. Yuan, et al., Chemistry, 2015, 21, 16547–16554 CrossRef CAS PubMed.
- Y. K. Li, Y. C. Li and X. Zhang, et al., Theranostics, 2016, 6, 1293–1305 CrossRef CAS PubMed.
- T. J. Ji, J. Y. Lang and J. Wang, et al., ACS Nano, 2017, 11, 8668–8678 CrossRef CAS PubMed.
- S. Wang, P. Huang and X. Y. Chen, Adv. Mater., 2016, 28, 7340–7364 CrossRef CAS PubMed.
- Y. Liu, H. C. van der Mei and B. R. Zhao, et al., Adv. Funct. Mater., 2017, 27, 1701974 CrossRef.
- T. T. Wang, D. G. Wang and J. P. Liu, et al., Nano Lett., 2017, 17, 5429–5436 CrossRef CAS PubMed.
- B. J. Du, J. H. a Liu and G. Y. Ding, et al., Nano Res., 2017, 10, 2280–2295 CrossRef CAS.
- W. Jiang, J. L. Wang and J. B. Yang, et al., Nano Res., 2018, 11, 5716–5734 CrossRef CAS.
- J. J. Liu, X. M. Guo and Z. Luo, et al., Nanoscale, 2018, 10, 13737–13750 RSC.
- W. Zhang, Y. Li and J. H. Sun, et al., Chem. Commun., 2015, 51, 1807–1810 RSC.
- F. J. Ai, T. Y. Sun and Z. F. Xu, et al., Dalton Trans., 2016, 45, 13052–13060 RSC.
- J. W. Li, Z. L. Lyv and Y. L. Li, et al., Biomaterials, 2015, 51, 12–21 CrossRef CAS PubMed.
- C. Q. You, H. S. Wu and M. X. Wang, et al., Mater. Sci. Eng., C, 2018, 92, 453–462 CrossRef CAS PubMed.
- H. Bi, Y. Dai and P. Yang, et al., Small, 2018, 14, e1703809 CrossRef PubMed.
- J. R. Peng, T. T. Qi and J. F. Liao, et al., Biomaterials, 2013, 34, 8726–8740 CrossRef CAS PubMed.
- K. Kurd, A. A. Khandagi and S. Davaran, et al., Artif. Cells, Nanomed., Biotechnol., 2016, 44, 1031–1039 CAS.
- Q. Zhou, C. Q. You and Y. Ling, et al., Life Sci., 2019, 217, 41–48 CrossRef CAS PubMed.
- R. Atluri, R. Atmaramani and G. Tharaka, et al., Nanomaterials, 2018, 8, 774 CrossRef PubMed.
- C. You, H. Wu and M. Wang, et al., Chemistry, 2017, 23, 5352 CrossRef CAS PubMed.
- D. Coluccia, C. A. Figueiredo and M. Y. J. Wu, et al., Nanomedicine, 2018, 14, 1137–1148 CrossRef CAS PubMed.
- H. C. He, A. W. Cattran and T. Nguyen, et al., Biomaterials, 2014, 35, 9546–9553 CrossRef CAS PubMed.
- X. Y. Cheng, D. J. Li and A. Q. Lin, et al., Int. J. Nanomed., 2018, 13, 3661–3677 CrossRef CAS PubMed.
- M. Pooresmaeil and H. Namazi, Eur. Polym. J., 2021, 142, 110126 CrossRef CAS.
- L. L. Tan, N. Song and S. X. A. Zhang, et al., J. Mater. Chem. B, 2016, 4, 135–140 RSC.
- A. S. Timin, A. R. Muslimov and K. V. Lepik, et al., J. Mater. Chem. B, 2016, 4, 7270–7282 RSC.
- G. L. Ma, W. F. Lin and Z. F. Yuan, et al., J. Mater. Chem. B, 2017, 5, 935–943 RSC.
- M. Hegazy, P. Zhou and G. Y. Wu, et al., Polym. Chem., 2017, 8, 5852–5864 RSC.
- S. Chen, Q. Bian and P. J. Wang, et al., Polym. Chem., 2017, 8, 6150–6157 RSC.
- T. M. Don, K. Y. Lu and L. J. Lin, et al., Mol. Pharm., 2017, 14, 4648–4660 CrossRef CAS PubMed.
- S. Ghavami, G. R. Bardajee, A. Mirshokraie and K. Didehban, et al, Polym. Sci., Ser. B, 2019, 61, 376–386 CrossRef.
- C. Siangsanoh, S. Ummartyotin and K. Sathirakul, et al., J. Mol. Liq., 2018, 256, 90–99 CrossRef CAS.
- Z. Fa, H. W. Ming and D. Liang, et al., ACS Appl. Mater. Interfaces, 2018, 10, 21939–21949 CrossRef PubMed.
- M. Shu, J. J. Tang and L. L. Chen, et al., Biomaterials, 2021, 268, 120574 CrossRef CAS PubMed.
- J. J. Liu, Q. Chen and W. W. Zhu, et al., Adv. Funct. Mater., 2017, 27, 1605926 CrossRef.
|
This journal is © The Royal Society of Chemistry 2023 |
Click here to see how this site uses Cookies. View our privacy policy here.