DOI:
10.1039/D3RA01294H
(Paper)
RSC Adv., 2023,
13, 13886-13891
Rugby ball-shaped magnetic microcapsule for tumor hyperthermia†
Received
25th February 2023
, Accepted 26th April 2023
First published on 9th May 2023
Abstract
Magnetic hyperthermia (MH) induced by magnetic particles has been widely used to treat tumors. However, the limited heating conversion efficiency inspires the design and synthesis of versatile magnetic materials for enhancing the performance of MH. Herein, we developed rugby ball-shaped magnetic microcapsules as efficient MH agents. The size and shape of the microcapsules can be precisely controlled by adjusting the reaction time and temperature without surfactant assistance. Because of their high saturation magnetization and uniform size/morphology, the microcapsules showed excellent thermal conversion efficiency, with a specific absorption rate of 2391 W g−1. Additionally, we performed in vivo anti-tumor studies on mice and found that MH mediated by magnetic microcapsules effectively inhibited the advancement of hepatocellular carcinoma. The microcapsules' porous structure might allow them to efficiently load different therapeutic drugs and/or functional species. These beneficial properties make microcapsules ideal candidates for medical applications, particularly in disease therapy and tissue engineering.
1. Introduction
Hepatocellular carcinoma (HCC) is one of the most common malignant tumors in the world,1 with high morbidity and mortality. Surgical resection, liver transplantation and physical ablation have been the traditional aggressive treatments for early-stage HCC, which can significantly improve the survival of HCC patients. Physical thermal ablation has been widely utilized in treating HCC because of its advantages of simple operation, less harm to the body and multiple therapy sessions. However, microwave, laser and radiofrequency therapeutic methods (1) cause uneven tissue heating, (2) lack selectivity in killing tumor cells and (3) make conformal ablation difficult, which limits their clinical efficacy. Furthermore, these modalities always induce a temperature higher than 65 °C, resulting in severe damage to normal cells.2 Therefore, continuous efforts should still be exerted to develop efficient HCC treatment approaches, as highlighted in tumor management.3
Magnetic hyperthermia (MH) is an effective anti-tumor modality. The European Union has authorized MH for treating glioblastoma multiforme.4–7 Recently, the U.S. Food and Drug Administration has initiated clinical trials to treat prostate8 and pancreatic9 cancers. The MH heating mechanism is based on the ability of magnetic particles to demonstrate hysteresis loss under an alternating magnetic field (AMF). Since tumor cells are more sensitive to high temperatures than normal cells, magnetic particles used as a heat source induce tumor apoptosis.10–12 Contrary to conventional thermal ablation, in which heating is targeted at the tissue level, MH is characterized by magnetic particles as a heat source and the intracellular effects of heating. Based on this, MH possesses the following unique advantages: (1) mild treatment temperature (39–45 °C); (2) uniform heating as particles are localized inside cells; (3) selective tumor cell killing; (4) well-defined biosafety and (5) deep tissue penetration. Undoubtedly, MH is an alternative and effective cancer treatment that deserves more research and enhancement.13–15 However, the limited heating conversion efficiency inspires the design and synthesis of versatile magnetic materials for improving the performance of MH.
In recent years, iron oxide materials have garnered considerable attention. Although many magnetic materials have been developed for MH, most studies only focus on its preparation methods, enhanced biocompatibility, low coercivity and colloidal stability.16–18 Most iron oxide nanoparticles require a low thermal conversion efficiency and a high injection dosage (milligram level).5 Many research efforts has shown the maximum specific absorption rate (SAR) value of ferric oxide particles with different sizes (7.6–416 nm) in the diplomatic magnetic field (80 kHz, 32.5 kA m−1) is 75.6 W g−1. And the SAR value decrease with the increase of particle size.19,20 It usually used template21 or etching method22 for fabrication of porous materials, however, the reaction process was complex and poorly controllable. Interestingly, researchers achieved iron oxide microparticles possessing high magnetic permeability, high saturation magnetization and low coercivity23 by designing their chemical components and modulating their size24 and shape,25 which have the potential to significantly improve heating conversion efficiency.26,27
In this study, rugby ball-shaped magnetic microcapsules with superior heating conversion efficiency were prepared for MH-triggered cancer treatment. The ‘hydrothermal and reduction’ method prepared microcapsules resembling rugby balls by adding inorganic salt to Fe3+ as a reaction precursor in a closed reactor while controlling the reaction time and temperature. Controlling the reaction parameters to modify the pore size and volume enabled a comprehensive investigation of the structural optimization of microcapsules for MH applications. The optimal magnetic microcapsules possessed a high SAR value and low coercivity, demonstrating a significant heating-generation capacity when exposed to AMF. When synthesized magnetic microcapsules were employed for in vivo anti-tumor treatment, the performance of magnetic agents in relieving HCC was achieved.
2. Experimental
2.1 Synthesis of magnetic particles
The ellipsoidal magnetic microcapsules were synthesized by hydrothermal growth of ellipsoidal hematite (α-Fe2O3) microcapsules and then used a gas reduction process to convert α-Fe2O3 into the other phase. The α-Fe2O3 microcapsules were synthesized by hydrothermally treating 3.0 mL of aqueous FeCl3 solution (0.5 M) and 0.72 mL of aqueous NH4H2PO4 (0.02 M) solution at 220 °C for different times. Distilled water was added to a final volume of 40 mL. After stirring for 10 min, the mixture was transferred into a Teflon hydrothermal reaction kettle with a capacity of 50 mL for hydrothermal treatment at 220 °C. The autoclave was naturally cooled down to room temperature about 25 °C. The precipitate was collected by centrifugation, washed with anhydrous ethanol and de-ionized water and dried under vacuum at 60 °C.
Then, 100 mg of α-Fe2O3 microcapsules were annealed in a horizontal quartz tube furnace at 430 °C, 450 °C and 500 °C under a constant flow of 5% H2/95% Ar for 120 min, respectively. The tube furnace was cooled to room temperature while maintaining the same gas atmosphere.
Microcapsules after reduction was coated with oleic acid. 30 mg microcapsules, 10 g octadecene and 0.4 mL oleic acid were mixed and heated to 280 °C for 50 min in argon environment. After the temperature of reaction system dropped to room temperature, anhydrous ethanol was added to the system, and then centrifuged at 8000 rpm min−1 for 10 min. The sediment was retrieved, and then it was washed three times with hexane and anhydrous ethanol. In order to better use in a physical environment, we convert the oil phase microcapsules into water phase by dopamine. 30 mg wrapped in oleic acid, 4 mL tetrahydrofuran and 200 mg dopamine were mixed and heated to 60 °C for 6 h in argon environment. After the completion of reaction, the tetrahydrofuran was volatilized and cleaned to obtain a completely dry microcapsules powder. The dried particles were then dispersed in water.
2.2 Characterization of magnetic microcapsules
The crystal phase of magnetic microcapsules was verified by X-ray diffraction (XRD, Bruker D8 Advanced Diffractometer System). The morphology and size of the microcapsules were observed via transmission electron microscopy (TEM, FEI Tecnai F30) and scanning electron microscopy (SEM, S-570, Hitachi). X-ray photoelectron spectroscopy (XPS) measurement was performed using a PHI-5000 VersaProbe III XPS (ULVAC-PHI, Inc., Japan) with Al Kα X-ray radiation as the X-ray source. The magnetic properties of magnetic microcapsules were characterized using a vibrating sample magnetometer (VSM, Beijing Xinke Technology Developments Co., Ltd.).
2.3 Measurement of SAR value
Magnetic microcapsules with 0.2 mg mL−1 Fe were placed in a 1.5 mL EP tube and inserted in a water-cooled magnetic induction coil. A fibre probe (SPG-10AB-II) connected to a computer was used to monitor the real-time temperature. The SAR value was calculated using the following equation:
where C is the specific heat capacity of agarose gel, ΔT/Δt is the initial slope of the temperature vs. time dependence, and mFe is the weight fraction of Fe.
2.4 Pore analysis
The surface area of magnetic microcapsules was measured by the Brunauer–Emmett–Teller (BET) method, followed analyzed by Tristar II 3020 for the absorption of nitrogen. The pore size and volume of microcapsules were calculated using the BJH method.
2.5 Cell culture
Mouse H22 hepatoma cells were supplied by the American type culture collection and cultured in RPMI 1640 complete media (Gibco, Carlsbad, CA, USA) supplemented with 10% (v/v) heat-inactivated fetal bovine serum (Biological Industries, USA) and 100 U mL−1 penicillin–streptomycin solutions (Hyclone Laboratories Inc., USA) at 37 °C in a humidified atmosphere containing 5% CO2.
2.6 In vitro cell cytotoxicity
The Fe ion concentration was determined using ICP-MS. The 100 μL per well H22 cells suspension (1 × 104 cells per well) was inoculated into a 96-well plate for culturing another 24 h. Different concentration of magnetic microcapsules suspension (10, 20, 30, 40, 50, 75, 100 μg mL−1) were added to each well, and the cells were incubated for 8 h. To remove excess suspension material, cells were washed by PBS. Cell viability was determined by CCK-8, and the absorbance was measured at 570 nm by microplate reader (Thermo Fisher Scientific, Wilmington, DE, USA).
2.7 H22 tumor-bearing mice model
Six-week-old female BALB/c mice were purchased from Beijing Vital River Laboratory Animal Technology Co., Ltd. (Beijing, China). The Animal Care and Use Committee of Northwest University approved the animal experiment protocols and complied with all relevant ethical regulations. H22 cells (5 × 105 cells per mice) were subcutaneously inoculated at the back of BALB/c mice (right side) to establish the subcutaneous H22 tumor model. When the tumor volumes reached approximately 100 mm3, mice were randomly divided into four groups: PBS (isotype control), microcapsules only, AMF only and microcapsules plus AMF (seven mice for each group). Tumor-bearing mice were subjected to one injection of microcapsules (0.1 mg of Fe per cm3) and three AMF treatments on days 1, 3 and 5. The mice were anaesthetized with 2% (v/v) isoflurane and then exposed to an AMF with a frequency of 365 kHz for 10 min. The tumor volume was calculated by the equation (length × width2)/2.
All animal experimental protocols were reviewed and approved by the Animal Care and Use Committee of the Institute of Process Engineering, Northwest University, and they complied with all relevant ethical regulations.
3. Result and discussion
3.1 Synthesis and characterization of α-Fe2O3 microcapsules
The magnetic microcapsules resembling rugby balls were synthesized using the hydrothermal and reduction method. The hematite (α-Fe2O3) microcapsules were prepared and then thermally reduced to produce Fe3O4 or iron-containing microparticles. The morphology of α-Fe2O3 microcapsules with different reaction times at 220 °C was observed via SEM. As shown in Fig. 1a–c, the size of the obtained α-Fe2O3 microcapsules did not significantly change during the prolonged reaction time. However, numerous pores appeared on the surface of α-Fe2O3 microcapsules at 60 h, and the pores were glaring. The surface area of α-Fe2O3 microcapsule was calculated according to the BET, and pore size were measured using the BJH method. As shown in Fig. 1d, the BET surface area reached 23 m2 g−1 under a reaction time of 0.5 h, which could be due to the various minute particles maintained in the mixture for inadequate reaction times. After that, remarkably, the specific surface area of the microcapsule generally increased within 10 h and reached its maximum when the reaction time was prolonged to 60 h. The pore size of the α-Fe2O3 microcapsules increased in a similar trend as the reaction time increased (Fig. 1e).
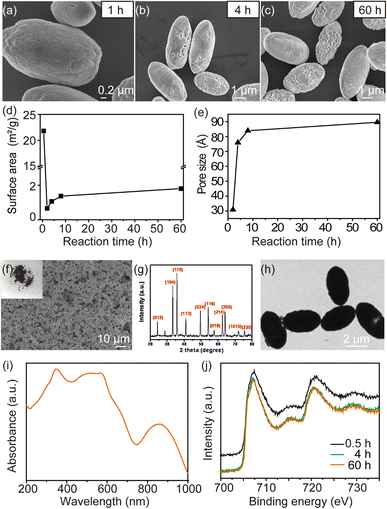 |
| Fig. 1 SEM images of the α-Fe2O3 microcapsules after different reaction times at 220 °C for (a) 1 h, (b) 4 h and (c) 60 h. (d) BET surface area and (e) pore size of the α-Fe2O3 microcapsules with different reaction times. (f) SEM image under low magnification of the α-Fe2O3 microcapsules with a photograph of α-Fe2O3 powder positioned in the upper-left corner. (g) XRD pattern, (h) TEM image, (i) UV absorption spectra and (j) Fe 2p spectra of α-Fe2O3 microcapsules fabricated at 220 °C for 60 h. | |
The α-Fe2O3 microcapsules prepared under 220 °C for 60 h showed uniform elliptical spherical shape and size distribution, suggesting good experimental reproducibility (Fig. 1f). The XRD results showed that the synthesized α-Fe2O3 microcapsules exclusively corresponded to the trigonal α-Fe2O3 (JCPDS 87-1165), and no other impurity peak was observed (Fig. 1g). Particularly, TEM images showed the obtained microcapsule had a rugby ball-shaped pattern with ∼2 μm in width and ∼3 μm in length (Fig. 1h), respectively. It was also observed that the aspect ratio of microcapsules resembling rugby balls was approximately 1.5.
As shown in Fig. 1i, compared with the superparamagnetic iron oxide (Fig. S1†), α-Fe2O3 microcapsules had the absorption at 860 nm. The samples were characterized using XPS to further verify the Fe element valence state of the obtained α-Fe2O3 species. The high-resolution Fe 2p spectra showed that two peaks at 724 eV (Fe 2p1/2) and 710 eV (Fe 2p3/2) were assigned to a Fe3+ species (Fig. 1j), which was consistent with the previous report.28–30
3.2 Characterization of magnetic microcapsules after reduction of α-Fe2O3 microcapsule
The XRD patterns of the prepared microcapsules under different reduction temperatures are shown in Fig. 2a. Clearly, the reduction temperature would have a significant impact on the formation of the final products. The Fe and Fe3O4 appeared when the reduction temperature was between 430 °C and 500 °C. At 450 °C, all of the diffraction peaks can be exclusively indexed as the cubic inverse spinel Fe3O4 (JCPDS no. 19-0629), and no other impurities were observed. Therefore, we propose that the composition-controlled synthesis of microcapsules could be achieved by adjusting the reduction temperature.
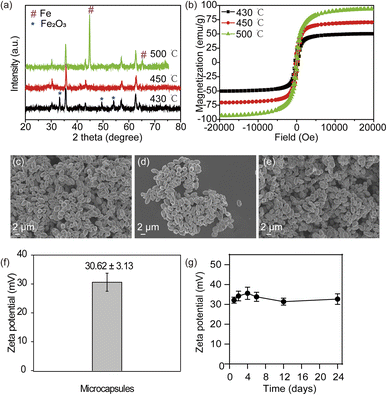 |
| Fig. 2 (a) XRD of the microcapsules after the reduction with different temperatures (430 °C, 450 °C and 500 °C). (b) Hysteresis loop of the microcapsules at 300 K after the reduction with different temperatures (430 °C, 450 °C and 500 °C). SEM image of microcapsules with different reduction temperatures (c) 430 °C, (d) 450 °C and (e) 500 °C. (f) Zeta potential of magnetic microcapsules in solution. (g) Zeta potential measured as a function of time upon incubation in solution. | |
Next, the morphology of microcapsules at various reduction temperatures was examined using SEM. As the SEM images are shown in Fig. 2c, all the porous microcapsules were uniform in their rugby ball shape. The large-scale microcapsules were observed clearly with uneven and rough surfaces at reduction temperature of 430 °C. When the reduction temperature increased to 450 °C, the high-magnification SEM image of the microcapsules showed that the size of the microcapsules was uniform and well dispersed, with an average width of ∼2 and ∼3 μm for length (Fig. 2d). Interestingly, the micropores appeared on the surface of the microcapsules and became noticeably rougher when the reduction temperature was changed to 500 °C (Fig. 2e). However, the morphology of microcapsules was not significantly changed.
The magnetic hysteresis loop of microcapsules was characterized using a VSM in order to examine the underlying relationship between the magnetic properties and the reduction temperature. As shown in Fig. 2b, the highest saturation magnetization (Ms) was recorded at ∼94 emu g−1 under the 500 °C reduction temperature. And microcapsules magnetized from 1500 to −1500 Oe, which lead to magnetic field energy loss and heat generation by hysteresis (Fig. S2†). Meanwhile, as the temperature was reduced, the Ms of microcapsules increased while the coercivity decreased (Table 1). As the Ms is the dominant factor for higher magnetic heating generation, the magnetic microcapsules synthesized under a 500 °C reduction temperature were chosen for the following experiments.
Table 1 The Ms and coercivity of the magnetic microcapsules with different temperature reductions (430 °C, 450 °C and 500 °C)
Reduction temperature |
Ms (emu g−1) |
Coercivity (Oe) |
430 °C |
50 |
419 |
450 °C |
70 |
324 |
500 °C |
94 |
241 |
The zeta potential of magnetic microcapsules is 30.62 ± 3.13 mV (Fig. 2f), which suggested the successful surface modification. Fig. 2g showed that the zeta potential of the magnetic microcapsules did not change for 24 days, indicating the good colloidal stability of the magnetic microcapsules.
3.3 Magnetic hyperthermia properties of magnetic microcapsules
To evaluate the heat induction capability of magnetic microcapsules under AMF, the temperature of magnetic microcapsules dispersed in agarose gel was monitored using an induction heating system. Briefly, 1 mL of magnetic microcapsules with a concentration of 0.2 mg mL−1 [Fe] were subjected to AMF with a frequency fixed at 365 kHz and an amplitude varying from 20 to 50 kA m−1. As shown in Fig. 3a, the temperature of magnetic microcapsules increased rapidly at different amplitudes. When the amplitude was set to 50 kA m−1, the temperature of the microcapsules could reach above 47 °C within 30 s. The calculated SAR of magnetic microcapsules based on temperature–time curves is shown in Fig. 3b. The larger SAR value can be detected with the higher amplitude of AMF. The largest SAR value of magnetic microcapsules was obtained for 2391 W g−1 with a Fe concentration of 0.2 mg mL−1 under AMF (365 kHz, 50 kA m−1), which is more significantly higher than the reported previously. These results indicated that magnetic microcapsules possessed an attractive magnetic heating generation ability.
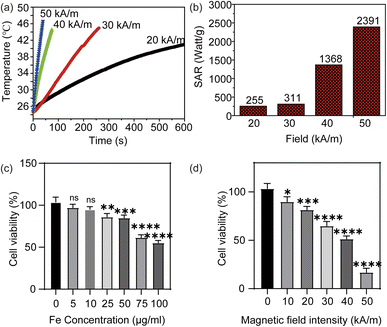 |
| Fig. 3 (a) Temperature versus time graphs and (b) SAR of magnetic microcapsules under different field intensities. (c) Cell viability of H22 exposed to magnetic microcapsules. 5–100 μg mL−1 concentration of Fe incubated with H22 for 24 h. (d) Cell viability of H22 cells subjected to magnetic microcapsules mediated hyperthermia under different magnetic field intensity. The frequency was 365 kHz. | |
We utilized H22 cells to evaluate whether magnetic microcapsules induced concentration-dependent cytotoxicity (Fig. 3c). After H22 cells being incubated with microcapsules at different Fe concentration, it was found that the cell viabilities of H22 was above 85% from 5 to 50 μg mL−1 of Fe concentration, which indicated that cytotoxicity below 50 μg mL−1 is negligible. Quantitative study of cellular uptake at a dosage of 50 μg mL−1 showed that the maximum accumulation of magnetic microcapsules occurred at a co-incubation time of 8 h, which could be the optimal time for cell hyperthermia (Fig. S3†). As shown in Fig. 3d, after co-incubation with 50 μg mL−1 microcapsules for 12 h, and cells exposed to different magnetic field intensity for 10 min, it showed magnetic field intensity-dependent cell death trends. At a magnetic field intensity of 40 kA m−1, the cell viabilities decreased to ∼48%.
3.4 Antitumor efficacy of magnetic microcapsule-mediated magnetic hyperthermia in hepatoma H22-bearing mice
Magnetic microcapsules with high SAR values can be used for many applications. To investigate the potential of magnetic microcapsules to induce a magnetic hyperthermia-mediated anti-tumor effect, in vivo experiments were performed on H22 tumor-bearing mice. In several experimental groups (n = 7), H22 cancer cells were xenografted onto the back of BALB/c mice (right side). Magnetic microcapsules were injected subcutaneously into the tumor (100 mm3). The mouse was placed in a water-cooled magnetic induction coil. An a.c. magnetic field of 365 kHz at 40 kA m−1 was applied for 10 min. The experimental design for the animal is shown in Fig. 4a. Following treatment, the tumor burden was monitored for up to 20 days. For the untreated control group of mice, tumor size increased 3-fold by day 19 (Fig. 4b). The mice treated with either AMF or magnetic microcapsules showed growth behaviors similar to the untreated control. However, for the group that received the hyperthermia treatment with magnetic microcapsule nanoparticles, the tumor growth was delayed during the same period. We also photographed the tumor tissues at the end of treatment (Fig. 4c), which visually confirmed that magnetic microcapsule plus AMF was most effective in suppressing tumor growth.
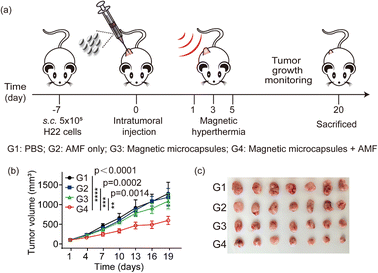 |
| Fig. 4 (a) Schematic illustration of experimental design. (b) Tumor images from each group after the animals were sacrificed. (c) H22 tumor growth curves after different treatment. The error bars represent the means ± SD. Differences among groups are determined using one-way ANOVA analysis **p < 0.01, ***p < 0.001, ****p < 0.0001. | |
4. Conclusions
We have successfully constructed a magnetic microcapsule resembling a rugby ball with high Ms and magnetic thermal conversion properties. The composition and morphology of microcapsules could be accurately controlled by controlling the reaction time and temperature, demonstrating superior dispersibility and dimensional homogeneity. As a customized magnetic induction hyperthermia agent for tumor treatment, magnetic microcapsules resembling rugby balls obtained a well-defined heating generation efficiency. In an in vivo experiment, microcapsules induced an efficient hepatocellular carcinoma inhibition effect, indicating magnetic microcapsules could be used as a promising magnetic hyperthermia agent. Therefore, our synthetic rugby ball-shaped magnetic microcapsules provide an alternative agent for future biological applications.
Ethical statement
All animal procedures were performed in accordance with the Guidelines for Care and Use of Laboratory Animals of Northwest University and approved by the Animal Ethics Committee of Northwest University. And we declare all experiments were performed in compliance with relevant laws or guidelines and all experiments followed institutional guidelines.
Conflicts of interest
There are no conflicts to declare.
Acknowledgements
This work was supported by National Key Research and Development Program of China (grant number 2022YFC2408000), National Natural Science Foundation of China (NSFC) projects (grant numbers 82072063, 32001005, 32101136, and 82202306), Key Research and Development Program of Shaanxi Province (grant number 2023-YBSF-132), Shaanxi Province Youth Science and Technology New Star (grant number 2022KJXX-09), Natural Science Foundation of Shaanxi Province (grant numbers 2020JQ610), and Science Foundation of Nanjing Chia Tai Tianqing Project (grant number TQ202215).
References
- H. Sung, J. Ferlay, R. L. Siegel, M. Laversanne, I. Soerjomataram, A. Jemal and F. Bray, Ca-Cancer J. Clin., 2021, 71, 209–249 CrossRef PubMed.
- S. L. Liauw, P. P. Connell and R. R. Weichselbaum, Sci. Transl. Med., 2013, 5, 1–32 CrossRef PubMed.
- T. Hehr, R. Wust, M. Bamberg and W. Budach, Onkologie, 2003, 26, 295–302 CAS.
- M. Johannsen, U. Gneveckow, K. Taymoorian, B. Thiesen, N. Waldofner, R. Scholz, K. Jung, A. Jordan, P. Wust and S. A. Loening, Int. J. Hyperthermia, 2007, 23, 315–323 CrossRef CAS PubMed.
- K. Maier-Hauff, F. Ulrich, D. Nestler, H. Niehoff, P. Wust, B. Thiesen, H. Orawa, V. Budach and A. Jordan, J. Neuro-Oncol., 2011, 103, 317–324 CrossRef PubMed.
- R. T. Gordon, J. R. Hines and D. Gordon, Med. Hypotheses, 1979, 5, 83–102 CrossRef CAS PubMed.
- A. Jordan, P. Wust, R. Scholz, B. Tesche, H. Fahling, T. Mitrovics, T. Vogl, J. Cervos-Navarro and R. Felix, Int. J. Hyperthermia, 1996, 12, 705–722 CrossRef CAS PubMed.
- K. Mahmoudi, A. Bouras, D. Bozec, R. Ivkov and C. Hadjipanayis, Int. J. Hyperthermia, 2018, 34, 1316–1328 CrossRef PubMed.
- S. V. Spirou, M. Basini, A. Lascialfari, C. Sangregorio and C. Innocenti, Nanomaterials, 2018, 8, 1–22 Search PubMed.
- X. L. Liu, Y. Yang, C. T. Ng, L. Y. Zhao, Y. Zhang, B. H. Bay, H. M. Fan and J. Ding, Adv. Mater., 2015, 27, 1939–1944 CrossRef CAS PubMed.
- P. G. Dai, W. J. Zhu, B. Yan, Y. Q. Miao, S. S. Hu, X. Gao, X. L. Liu, Y. F. Zhang, G. L. Li, T. B. Zhang, H. Zhang and H. M. Fan, Adv. Ther., 2021, 4, 200291 Search PubMed.
- B. Hildebrandt, P. Wust, O. Ahlers, A. Dieing, G. Sreenivasa, T. Kerner, R. Felix and H. Riess, Crit. Rev. Oncol. Hematol., 2002, 43, 33–56 CrossRef PubMed.
- A. Hervault and N. T. K. Thanh, Nanoscale, 2014, 6, 11553–11573 RSC.
- J. Pan, Y. Xu, Q. Wu, P. Hu and J. Shi, J. Am. Chem. Soc., 2021, 143, 8116–8128 CrossRef CAS PubMed.
- E. A. Perigo, G. Hemery, O. Sandre, D. Ortega, E. Garaio, F. Plazaola and F. J. Teran, Appl. Phys. Rev., 2015, 2, 041302 Search PubMed.
- R. Hergt, S. Dutz and M. Roder, J. Phys.: Condens. Matter, 2008, 20, 385214 CrossRef PubMed.
- S. Laurent, S. Dutz, U. O. Hafeli and M. Mahmoudi, Adv. Colloid Interface Sci., 2011, 166, 8–23 CrossRef CAS PubMed.
- J. Dulinska-Litewka, A. Lazarczyk, P. Halubiec, O. Szafranski, K. Karnas and A. Karewicz, Materials, 2019, 12, 12040617 CrossRef PubMed.
- M. Ma, Y. Wu, J. Zhou, Y. Sun, Y. Zhang and N. Gu, J. Magn. Magn. Mater., 2004, 268, 33–39 CrossRef CAS.
- T. Hosono, H. Takahashi, Y. Sato, K. Tohji and B. Jeyadevan, AIP Conf. Proc., 2007, 898, 135–138 CrossRef CAS.
- L. Wang, D. Z. Ni, D. Yang, K. B. Zhou and S. Yang, Chem. Lett., 2010, 39, 451–453 CrossRef CAS.
- Y. Q. Shen, F. Zhang, P. F. Song, Y. C. Zhang, T. Zhang, X. Q. Wen, J. Q. Ma, D. Zhang and X. Y. Du, J. Alloys Compd., 2022, 903, 163971 CrossRef CAS.
- S. K. Boda, A. V. Anupama, B. Basu and B. Sahoo, J. Phys. Chem. C, 2015, 119, 6539–6555 CrossRef CAS.
- A. A. Taheri and M. Taghilou, Int. J. Eng., 2021, 34, 263–271 Search PubMed.
- H. X. Gao, T. B. Zhang, Y. F. Zhang, Y. M. Chen, B. Liu, J. P. Wu, X. L. Liu, Y. D. Li, M. L. Peng, Y. Zhang, G. Xie, F. Q. Zhao and H. M. Fan, J. Mater. Chem. B, 2020, 8, 515–522 RSC.
- L. Lai, H. Zhou and B. Lai, Chem. Eng. J., 2018, 349, 633–645 CrossRef CAS.
- J. Li, J. Yan, G. Yao, Y. Zhang, X. Li and B. Lai, Chem. Eng. J., 2019, 361, 1317–1332 CrossRef CAS.
- Y. Wang, C. Xie, D. Liu, X. Huang, J. Huo and S. Wang, ACS Appl. Mater. Interfaces, 2016, 8, 18652–18657 CrossRef CAS PubMed.
- D. Li, J. Zhou, X. Chen and H. Song, ACS Appl. Mater. Interfaces, 2016, 8, 30899–30907 CrossRef CAS PubMed.
- Z. Yu, H. Li, J. Lu, X. Zhang, N. Liu and X. Zhang, Electrochim. Acta, 2015, 158, 264–270 CrossRef CAS.
|
This journal is © The Royal Society of Chemistry 2023 |
Click here to see how this site uses Cookies. View our privacy policy here.