DOI:
10.1039/D3RA01679J
(Paper)
RSC Adv., 2023,
13, 15833-15842
Strategies for designing low thermal quenching upconverting temperature sensors
Received
14th March 2023
, Accepted 1st May 2023
First published on 25th May 2023
Abstract
The Er3+/Yb3+:NaGd(WO4)2 phosphors and the phosphor-in-glass (PIG) have been synthesized employing a typical approach to investigate their structural, morphological and optical properties. Several PIG samples containing different amounts of NaGd(WO4)2 phosphor have been manufactured by sintering the phosphor and glass [TeO2–WO3–ZnO–TiO2] frit together at 550 °C, and its impact on the luminescence characteristics has been extensively studied. It has been noticed that the upconversion (UC) emission spectra of PIG under 980 nm excitation display similar characteristic emission peaks to the phosphors. The maximum absolute sensitivity of the phosphor and PIG is 17.3 × 10−3 K−1 @ 473 K and the maximum value of relative sensitivity is 10.0 × 10−3 K−1 @ 296 K and 10.7 × 10−3 K−1 @ 298 K, respectively. However, thermal resolution at room temperature has been improved in the case of PIG as compared to the NaGd(WO4)2 phosphor. As compared to the Er3+/Yb3+ codoped phosphor and glass, the less thermal quenching of luminescence has been observed in PIG.
1. Introduction
The existing era of luminescence-based applications is based mainly on rare-earth (RE) doped materials owing to their high luminescence efficiency, long lifetime, low power consumption, excellent stability, and flexible control of photometric properties.1–5 Several methodologies have been tried by the researchers to develop new types of RE-doped luminescent materials for better results in various applications. The RE-doped luminescent materials have two categories, i.e., downconverting and upconverting luminescent materials. Among these, the upconverting luminescent materials have some advantages over the downconverting ones, such as the absence of background autofluorescence, high penetration depth and cheap excitation source.6 The upconverting materials have shown encouraging results in applications including optical temperature sensing, solar cells, photodynamic therapy (PDT), and bioimaging.7–10 In search of better results, researchers have tried to synthesize different types of functionalized upconverting nanoparticles (UCNPs) such as core@shell NPs, dye-sensitized UCNPs, and heavily doped UCNPs for various applications.11 One such approach that is attracting researchers nowadays is phosphor-in-glass (PIG) based luminescent materials.
PIG, a mixture of a RE-doped phosphor and transparent glass sintered at low temperature (usually <800 °C), has garnered the attention of researchers because of its low production cost and customized synthesis.12 Compared to conventional binders such as silicones and organic resins, transparent glasses are more suitable for embedding phosphors as they prevent thermal degradation of phosphors due to low sintering temperature. A number of glass systems such as silicates, borates, phosphates, tellurites, and oxy-fluorides have been found suitable for synthesizing PIG structures.12 Also, unlike ceramic phosphor plate (CPP) or phosphor glass ceramic (PGC), the PIG structure uses a small amount of phosphor, which lowers the production cost. On the other hand, the PIG structure can be synthesized from a variety of phosphors depending on the type of transparent glass. Ever since its invention, PIG materials involving downconverting phosphors have shown promising results in high-power LEDs.12–14 Also, Zeng et al. have reported lifetime based optical temperature sensing using the Sm2+ doped SrB4O7:TeO2–ZnO PIG structure.15
But it is surprising that despite showing such commendable results with downconverting phosphors, reports on upconverting phosphor-based PIG structures are not found to be available to the best of our information. This knowledge gap serves as a basis of the present study, in which the optical thermometry and thermal quenching of luminescence in the Er3+/Yb3+ codoped NaGd(WO4)2:TeO2–WO3–ZnO–TiO2 (TWZTi) PIG structure have been studied extensively using 980 nm excitation. NaGd(WO4)2 is an A+B3+(WO4)2 alkali rare-earth double tungstate type host having a monoclinic or tetragonal system and scheelite like structure, where A+ = alkali ions (Li+, Na+, K+, Rb+ and Cs+) and B3+ = Y3+ and RE3+. In this type of structure, the RE and alkali ion occupy the same site, thereby increasing the structural disorder.16–19 Moreover, the NaGd(WO4)2 host matrix having relatively low phonon frequency is more stable and environment friendly in comparison to hosts like fluorides, sulfides, etc.20 This host has been selected due to its potential in the field of luminescence.21,22 On the other hand, TWZTi is a heavy-metal oxide based transparent glass, in which WO3, ZnO and TiO2 act as network modifiers. The addition of WO3 is well-known for enhancing the thermal stability of glass by forming strong Te__O__W linkages.23 The addition of ZnO will increase the density of the glass and decrease its optical bandgap, thereby increasing its refractive index as well. The last modifier TiO2 enhances the chemical durability of the glass. Moreover, the addition of TiO2 also decreases the optical bandgap of the glass, causing an increase in its refractive index.24,25
The current work consists of synthesis of Er3+/Yb3+:NaGd(WO4)2 phosphors and structural and optical studies such as XRD, FESEM, FTIR, Raman, UV-vis spectroscopy, UC emission, etc. The synthesized phosphors have also been utilized for anticounterfeiting purposes. The thermal stability of the prepared phosphors has been confirmed using the Arrhenius equation. Based on the observations, PIG using (TWZTi) glass has been developed at varying concentrations of optimized phosphors and made applicable for upconversion-based temperature sensing application for the first time. Also, the temperature dependent characteristics of the developed PIGs have been compared to those of the optimized phosphors and glass.
2. Material synthesis and characterization
2.1 Synthesis of phosphors
The Er3+/Yb3+:NaGd(WO4)2 phosphors have been synthesized by the standard solid-state reaction method. The precursors Na2CO3 (RANKEM > 99.5%), Gd2O3 (CDH, >99.9%), WO3 (CDH, 99.0%), Er2O3 (CDH, 99.99%) and Yb2O3 (Sigma-Aldrich, 99.9%) were used in the preparation of the phosphors according to the following chemical expressions:
Na2CO3 + Gd2O3 + 4WO3 → 2NaGd(WO4)2 + CO2 |
(100 − x)NaGd(WO4)2 + xEr2O3 |
where x = 0.1, 0.3, 0.5 and 0.7 mol%.
(100 − x − y)NaGd(WO4)2 + xEr2O3 + yYb2O3 |
where x = 0.5; y = 1.0, 3.0, 5.0 and 7.0 mol%.
The mixture was brought to a stoichiometric ratio and pulverized using acetone in a mortar and pestle for 90 minutes. The mixed reagents were maintained at 1000 °C for three hours to facilitate high temperature synthesis in a muffle furnace. After naturally cooling to ambient temperature, the powder phosphors were out of the way for further investigations.
2.2 Preparation of glass
As reported,26,27 a TeO2-based glass system with specifications such as a low phonon energy of 800 cm−1, refractive index of 1.97–2.14 and melting temperature of 800 °C has been selected. The bare glass with the 70TeO2 + 15WO3 + 10ZnO + 5TiO2 (TWZTi) molar composition has been prepared with the melt-quenching technique. In this procedure, the high purity (in mol%) powder forms of the glass precursors TeO2, WO3, ZnO and TiO2 were weighed to yield 4 g of glass compositions, and the mixture was then ground in an agate mortar for two hours to obtain a fine and uniform mixture. The raw mixed material compositions were put into alumina crucibles and then heated to a temperature of 900 °C in a high-temperature electric furnace until the entire mixture became a transparent liquid. By pouring the obtained melt into a pre-heated brass mould and covering it with a hot flat brass plate, the melt was quickly quenched. A transparent glass of ∼3 mm thickness was obtained, as shown in Fig. 2(f), and used for PIG development. Also, a separate optimized Er3+/Yb3+:TWZTi glass was synthesized for comparative study. The dopant concentration was kept the same as that of phosphors.
2.3 Preparation of PIG
The obtained undoped TWZTi glass was crushed into powders (glass frits) and mixed with optimized Er3+/Yb3+:NaGd(WO4)2 phosphors. The phosphors in glass with the concentration of 1.0, 5.0, 10.0 and 20.0 weight% were named PIG1, PIG2, PIG3 and PIG4, respectively. The mixture powder of 0.7 g was used to make pellets and sintered at 550 °C for 45 min. The as-prepared PIGs were used for the photoluminescence study.
2.4 Characterization
To determine the crystal formation and lattice parameters, the optimized Er3+/Yb3+:NaGd(WO4)2 phosphors were analyzed using an X-ray diffractometer in the 10° ≤ 2θ ≤ 80° range. FESEM consisting of an airlock compartment has been used for morphological analysis. The vibrational bands have been detected with FTIR. XPS confirms the valence states and binding energies of the elements in phosphors. Diffuse reflectance spectra (DRS) have been monitored in the UV-Vis-NIR region. The continuous wave (CW) laser source has been used to achieve the frequency UC. The monochromator used for this purpose consists of a triple grating and a photomultiplier tube. Thermal stability experiments have been carried out using a multimeter, thermocouple, and a small heater that is maintained by altering the voltage. Calculations of CIE coordinates have been performed using GoCIE software.
3. Results and discussion
3.1 Structural characterization
The crystal formation of the developed Er3+/Yb3+:NaGd(WO4)2 phosphors has been confirmed with the XRD pattern {Fig. 1(a)}. The peak positions coincide with the JCPDS file 25-0829 with no impurity phase. The NaGd(WO4)2 has a scheelite-like tetragonal structure with the I41/a space group and cell parameters of a = b = 5.22 Å, c = 11.32 Å, α = β = γ = 90° and volume = 309.21 Å3. The XRD peaks of the phosphors correspond to (101), (112), (103), (004), (200), (211), (114), (105), (213), (204), (220), (301), (116), (215), (312), (321), (305), (323), (400), (208) and (332) (hkl) planes. The crystallite size (D) can be given by the Debye–Scherrer equation,28 |
 | (1) |
where ‘λ’ denotes the wavelength of X-ray used (λ = 1.5406 Å), ‘β’ signifies the full width at half maximum and ‘θ’ represents the Bragg's diffraction angle. The crystallite sizes of ∼74.65 nm, ∼67.67 nm and ∼59.70 nm of the NaGd(WO4)2, Er3+:NaGd(WO4)2 and Er3+/Yb3+:NaGd(WO4)2 phosphors have been calculated, respectively. Again, using the relation between the radius of the host cation ‘Rm(CN)’ and doped ion ‘Rd(CN)’, the acceptable percent of doping (Dr) can be confirmed as29 |
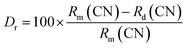 | (2) |
where the ionic radii of Na+, Gd3+, W6+, Er3+ and Yb3+ are 1.02 Å, 0.93 Å, 0.60 Å, 0.89 Å and 0.86 Å in VI coordination, respectively.30 The value of Dr was found to be 4% for Er3+ and 7% for Yb3+ doping in Gd3+ sites of the NaGd(WO4)2 crystal, which are in agreement with the fact Dr < 30%. The substitution of rare earths with a similar ionic radius and the same ionic charge does not disturb the crystal structure.
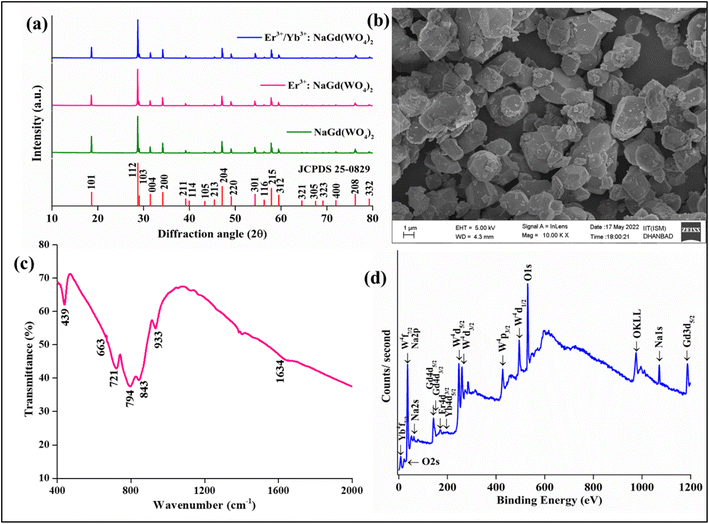 |
| Fig. 1 (a) XRD spectra of NaGd(WO4)2, Er3+:NaGd(WO4)2 and Er3+/Yb3+:NaGd(WO4)2 phosphors, (b) FESEM of optimized Er3+/Yb3+:NaGd(WO4)2 phosphors, (c) FTIR spectrum of the NaGd(WO4)2 host and (d) XPS of Er3+/Yb3+:NaGd(WO4)2 phosphors. | |
The FESEM image of prepared Er3+/Yb3+:NaGd(WO4)2 phosphors is shown in Fig. 1(b) on the 1 μm scale with almost circular shape particles of various sizes.
The vibrational bands at ∼439 cm−1, ∼663 cm−1, ∼721 cm−1, ∼794 cm−1, ∼843 cm−1, ∼933 cm−1 and ∼1634 cm−1 have been detected in the FTIR spectrum of the host NaGd(WO4)2 {Fig. 1(c)}. The broad peak at ∼1634 cm−1 is due to the stretching vibration of the hydroxyl (-OH) group. The peak at ∼933 cm−1 corresponds to W–O asymmetric stretching vibrations.31 The band at ∼843 cm−1 is resulted from O–W–O stretching of the WO4 tetrahedron, which confirms that the prepared phosphors are AWO4 type scheelite oxides and S4 site symmetry for the (WO4)2 groups.31,32 The vibrational energy of phosphors represents the maximum possible non-radiative transitions during excitation and emission processes. The other stretching vibrations of the WO4 tetrahedron have been observed at 663 cm−1, 721 cm−1 and 794 cm−1. The 439 cm−1 peak is related to the bending mode of the WO4 tetrahedron.32 The detected FTIR bands in the 400–2000 cm−1 range are well matched with those of the double tungstate structure.31,32
Fig. 1(d) displays the XPS spectrum of Er3+/Yb3+:NaGd(WO4)2 phosphors. The spectrum confirms the elements present in the prepared phosphors. The calibration has been performed with respect to the C 1s (∼284 eV) peak. The XPS peaks of oxygen are observed at ∼21 eV (O 2s) and ∼530 eV (O 1s). One Auger peak at ∼974 eV (OKLL) has also been detected in the XPS spectrum.33 The peaks for tungsten are found at ∼35 eV (4f7/2), ∼247 eV (4d5/2), ∼259 eV (4d3/2), ∼426 eV (4p3/2) and ∼495 eV (4p1/2).33,34 The XPS peaks of sodium are detected at ∼35 eV (2p), ∼62 eV (2s) and ∼1071 eV (1s).34 The two overlapped peaks of gadolinium are at ∼143 eV and ∼147 eV due to 4d5/2 and 4d5/2 states.34,35 One more peak of Gd has been found at ∼1186 eV (3d5/2).34 The binding energy peak for Er3+ has been found at ∼170.0 eV (4d3/2). The ∼7.0 eV and ∼184 eV peaks correspond to 4f5/2 and 4d5/2 states due to Yb3+ ions.33
3.2 Optical study
The UV-vis spectra of the NaGd(WO4)2 and optimized 0.5 mol% Er3+/3.0 mol% Yb3+:NaGd(WO4)2 phosphors have been recorded in the 200–1800 nm wavelength range {Fig. 2(a)}. The spectra were calibrated with the standard BaSO4 powder. The host NaGd(WO4)2 does not contain any absorption peak. The Er3+/Yb3+:NaGd(WO4)2 phosphor consists of various absorption peaks at ∼366 nm, ∼378 nm, ∼488 nm, ∼520 nm, ∼529 nm, ∼544 nm, ∼551 nm, ∼655 nm, ∼677 nm, ∼799 nm, ∼974 nm and ∼1498 nm. The respective transitions originated from the ground state (4I15/2) of Er3+ ions to the 2G9/2, 4G11/2, 4F7/2, 2H11/2 (I), 2H11/2 (II), 4S3/2 (I), 4S3/2 (II), 4F9/2, 4I9/2, 4I11/2 and 4I13/2 excited states. The intense peak at 974 nm has been observed due to the overlapping transitions from the Yb3+ and Er3+ ions. Further, the DRS spectra can be used to find the bandgap of the developed phosphors using the following Kubelka–Munk function F(R∞) and Tauc relationship by36 |
[F(R∞)hν] = C(hν − Eg)n
| (3) |
|
F(R)= (1 − R)2/2R = K/S
| (4) |
where F(R∞) represents the reflectance of the infinitely thick sample with respect to the reference at each wavelength, ‘C’ is a constant, ‘hν’ signifies the photon energy, ‘Eg’ indicates the bandgap and n = ½ for direct bandgap. ‘R’ designates the diffuse reflectance of the spectrum, ‘K’ signifies the absorption coefficient and ‘S’ is the scattering coefficient. The calculated bandgaps for NaGd(WO4)2 and Er3+/Yb3+:NaGd(WO4)2 phosphors are ∼4.2 eV and ∼4.3 eV, respectively, with negligible difference {Fig. 2(a), inset}.
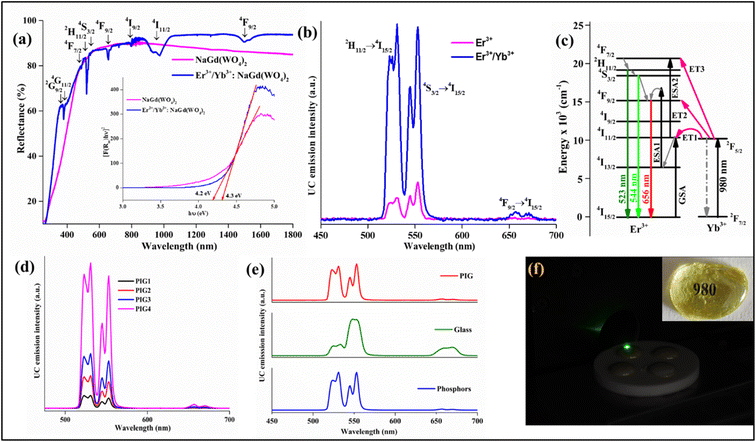 |
| Fig. 2 (a) DRS spectra and calculated bandgap of the NaGd(WO4)2 host and optimized Er3+/Yb3+:NaGd(WO4)2 phosphors, (b) UC emission of optimized Er3+ and Er3+/Yb3+:NaGd(WO4)2 phosphors, (c) energy level diagram of the Er3+/Yb3+ system, (d) UC emission from PIGs with variation of phosphor contents (1.0, 5.0, 10.0 and 20.0 weight%), (e) comparative UC emission spectra for phosphors, glass and PIG and (f) developed PIGs on 980 nm excitation; the inset shows the transparency of the as-prepared undoped glass. | |
Frequency upconversion emission studies. The UC emission spectra of the prepared phosphors have been recorded at room temperature upon 980 nm excitation over the 450–700 nm {Fig. 2(b)} region and the optimal concentrations of Er3+ and Yb3+ have been found to be 0.5 and 3.0 mol%, respectively. The 0.5 mol% Er3+:NaGd(WO4)2 phosphors contain green emission peaks at ∼523 nm and ∼544 nm, corresponding to the 2H11/2 (H) → 4I15/2 and 4S3/2 (S) → 4I15/2 transitions, and a red emission peak at ∼656 nm due to the 4F9/2 → 4I15/2 transition. Beyond 0.5 mol% concentration, quenching of luminescence intensity has been observed in the prepared phosphors. To understand the reason behind the quenching, the critical distance has been calculated using Blesse's equation.29 The critical distance represents the value up to which the energy transfer between two Er3+ ions can take place. The obtained value of critical distance is ∼6.6 Å, which is greater than 5 Å, indicating that the quenching has occurred due to multipolar interaction.29 Further, to get an insight into the type of multipolar interaction, Dexter and Van Uitert's relationship has been used.29 The obtained slope value (∼8) indicates that the multipolar interaction between the Er3+ ions is electric dipole–quadrupole in nature. This interaction is mainly responsible for the concentration quenching in the doped NaGd(WO4)2 phosphors. Codoping with Yb3+ ions enhances the luminescence intensity by ∼7 times by transferring the excitation energy from the Yb3+ to Er3+ ions.The pump-power dependent UC emission spectra of the optimized Er3+ and Er3+/Yb3+:NaGd(WO4)2 phosphors have been recorded by varying the pump power density from 1.36 to 75.9 W cm−2. With the increase in pump power density, the UC emission intensity increases. However, in Er3+/Yb3+:NaGd(WO4)2 phosphors, the UC emission intensity beyond 66.9 W cm−2 decreases. This decrease can be attributed to the thermal quenching effect generated in the samples due to the exposure to 980 nm CW laser radiation.37 The UC emission intensity (I) and pump power (p) are related by the expression I ∝ pn, where ‘n’ is the pump photons necessary for populating energy levels. The ‘n’ values for H and S levels in the case of 0.5 mol% Er3+ doped NaGd(WO4)2 phosphors are ∼1.7 and ∼1.5, respectively. The deviation of the slope value from two is due to the involvement of ESA and cross relaxation processes.19 In the case of 0.5 Er3+/3.0 Yb3+ doped NaGd(WO4)2 phosphors the ‘n’ values are ∼1.2 and ∼1.0. The decrease in the slope value from the standard value (∼2) is due to the competition between the linear decay and the upconversion processes. This competition has been theoretically explained in detail on the basis of rate-law equations.37,38
Further, PIG has been developed with varying contents of optimized phosphors. With the increase of phosphor content, the UC emission intensity increases. With the increase in pump power density as well, the UC emission intensity increases and the calculated slope value ‘n’ for PIG is ∼1.4 and ∼1.2 for H and S levels, respectively. However, the less intensity of PIG compared to phosphors is mainly due to the smaller composition (20%) of phosphors in the glass frits {Fig. 2(d)}. The UC emission spectra of developed phosphors, PIG and glass have been recorded for comparative study {Fig. 2(e)}. The PIG contains the characteristic peak shape of the developed phosphors. In the optimized glass, the intensity of the red emission band has increased, but the stark levels in green bands are not visible. The UC emission from the developed PIG upon 980 nm excitation is shown in Fig. 2(f).
The UC mechanism upon 980 nm excitation in the Er3+, Er3+/Yb3+ system has been explained with the energy level diagram {Fig. 2(c)}. In Er3+ doped NaGd(WO4)2 phosphors, the energy levels are populated by GSA/ESA processes. By absorbing 980 nm photons, Er3+ ions are excited from the ground state (4I15/2) to the excited state (4I11/2) through the GSA process. From there, some of the ions decay via a non-radiative process to the 4I13/2 level and then again excited to the 4F9/2 level via the ESA1 process. The radiative transition from the 4F9/2 level gives red emission at 656 nm. Apart from this, few ions in the 4I11/2 levels get excited to the 4F7/2 level by sequential absorption via the ESA2 process. From the 4F7/2 level the ions relax further to the 2H11/2 and 4S3/2 levels. The radiative transitions from the 2H11/2 and 4S3/2 levels result in green emissions at 523 nm and 544 nm, respectively. The codoping with Yb3+ ions enhances the UC emission intensity associated with the following energy transfer processes:
ET1: 2F5/2 (Yb3+) + 4I15/2 (Er3+) → 2F7/2 (Yb3+) + 4I11/2 (Er3+) |
ET2: 2F5/2 (Yb3+) + 4I13/2 (Er3+) → 2F7/2 (Yb3+) + 4F9/2 (Er3+) |
ET3: 2F5/2 (Yb3+) + 4I11/2 (Er3+) → 2F7/2 (Yb3+) + 4F7/2 (Er3+), 4F7/2 (Er3+) → NRR → 2H11/2, 4S3/2. |
Photometric characterization and security ink application. The colour emission from the phosphors has been visualized with CIE coordinates. The color coordinates for Er3+:NaGd(WO4)2 and Er3+/Yb3+:NaGd(WO4)2 phosphors were calculated to be (0.23, 0.73) and (0.20, 0.76), respectively {Fig. 3(a)}. The CIE coordinates shifted towards the greenish region with the incorporation of Yb3+ ions in the Er3+:NaGd(WO4)2 phosphors. The UC emission from codoped phosphors upon 980 nm excitation is shown in Fig. 3(b). The developed phosphors have been applied for making security inks. The letters ‘Ψ’ and ‘IIT’ have been written on white paper by dissolving phosphors into ethanol {Fig. 3(c and e)}. The letters were invisible under natural day light illumination, but upon 980 nm excitation the letters became visible {Fig. 3(d and f)}. In this way, the present upconverting phosphors can protect from counterfeiting threats by keeping confidential documents and valuable products secured.
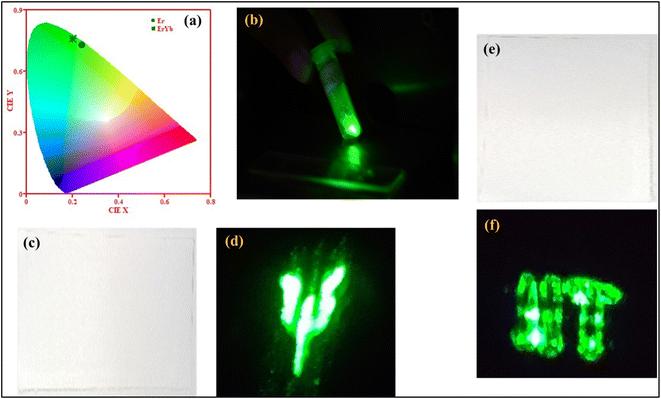 |
| Fig. 3 (a) CIE diagram of Er3+:NaGd(WO4)2 and Er3+/Yb3+:NaGd(WO4)2 phosphors, (b) green emission from optimized Er3+/Yb3+:NaGd(WO4)2 phosphors, and (c) and (e) the letter ‘ψ’ and letters ‘IIT’ upon natural day light illumination, and (d) and (f) upon 980 nm excitation. | |
Temperature sensing. The thermally coupled energy levels (TCLs) present in the Er3+ can be explored for temperature sensing application using the fluorescence intensity ratio (FIR) approach. The temperature dependent UC emission spectra of the Er3+/Yb3+:NaGd(WO4)2 phosphors (296–623 K), glass (296–623 K) and PIG (298–523 K) have been recorded. In both cases, the population at the H level increases up to 403 K and then decreases with respect to temperature. The TCL-based FIR technique can be applied to the UC peaks at 523 nm (2H11/2) and 544 nm (4S3/2) of Er3+ ions, as the energy difference between the 2H11/2 and 4S3/2 levels is ∼738 cm−1. The effect of rising temperature on the TCLs (H and S), i.e., temperature-induced population redistribution ability (PRA), can be analyzed with the expression36,39 |
 | (5) |
PRA for phosphors varies from 0.58 to 0.85 (296–623 K), from 0.53 to 0.82 (296–523 K) for PIG and 0.22 to 0.55 (301–623 K) for glass. The population of TCLs follows the Boltzmann distribution and FIR is given by the relation36,39
|
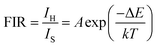 | (6) |
where
IH and
IS are the integrated intensities of thermally coupled green bands, ‘
A’ is the proportionality constant, Δ
E is the energy difference between these two levels and
k is Boltzmann's constant (0.695 K
−1 cm
−1). Δ
E between the
2H
11/2 and
4S
3/2 levels can be determined by linear fitting {
Fig. 4(d)}. For phosphors, the obtained Δ
E value is ∼612.31 cm
−1, for PIG it is ∼956.85 cm
−1 and for glass it is ∼902 cm
−1. Further, error ‘
δ’, the discrepancy between the fitted energy difference (Δ
E) and the experimental energy difference (Δ
E0), can be used to assess the accuracy of temperature sensing using the expression
39 |
 | (7) |
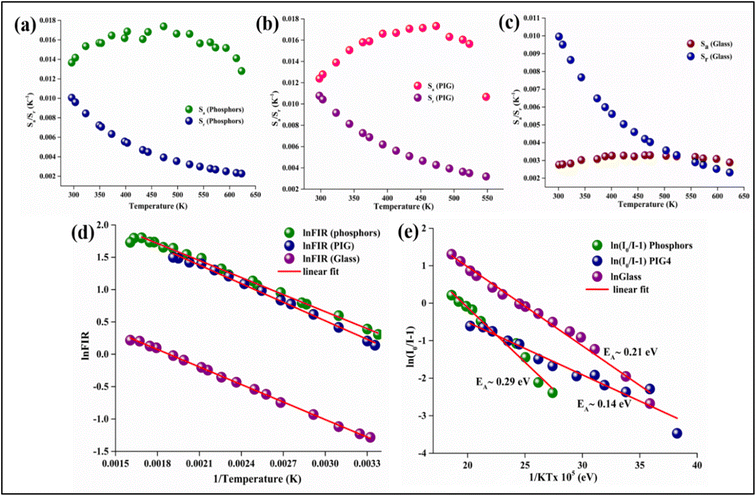 |
| Fig. 4 Absolute and relative sensitivity of Er3+/Yb3+:NaGd(WO4)2 (a) phosphors, (b) PIG, and (c) glass, (d) plot of ln FIR vs. 1/T (K) and (e) activation energy of phosphors, PIG and glass. | |
The calculated errors for phosphors, PIG and glass come out to be 0.20, 0.10 and 0.15, respectively. The obtained value of δ is small and is minimum in the case of PIG. Thus, the weak energy transfer between the two thermally coupled levels and other levels can be neglected.41
The obtained ΔE can be used to find the sensitivities, i.e., how often a sensor can detect even the smallest temperature changes. The relative (Sr) and absolute (Sa) sensitivities can be estimated by the following expressions21,42
|
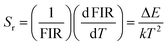 | (8) |
|
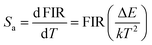 | (9) |
The graph for Sr and Sa demonstrates that the absolute sensitivity rises with temperature, reaching a maximum sensitivity, and then decreases {Fig. 4(a)–(c)}. For both phosphors and PIG, the Sa is 17.3 × 10−3 K−1 @ 473 K. However, the relative sensitivity is found to be 10.0 × 10−3 K−1 @ 296 K for phosphors and a maximum of 10.7 × 10−3 K−1 @ 298 K for the PIG. Although there is a difference in ΔE value for phosphors and PIG, no detectable change in the sensitivities has been observed. The low intensity observed for PIG does not affect the sensitivity. Thus, both phosphors and PIG can be used for sensor development. Besides, for Er3+/Yb3+:glass the values of Sa and Sr are found to be 3.29 × 10−3 K−1 @ 473 K and 9.96 × 10−3 K−1 @ 301 K, respectively.
The sensitivities for different optical temperature sensing materials are listed in Table 1. Further, the temperature where a sensor performs best, i.e., maximum sensitivity Smax and the temperature Tmax can be obtained by putting dSa/dT = 0 as38,43
|
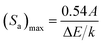 | (10) |
|
 | (11) |
Table 1 Comparison of sensitivities in different Er3+/Yb3+ doped tungstate materials using the FIR technique in 2H11/2 and 4S3/2 levels
Phosphors/glass/phosphors in glass |
Temperature range (K) |
Sa (×10−3 K−1) |
Sr (×10−3 K−1) |
Reference |
Er3+/Yb3+:BaWO4 phosphors |
293–393 |
10.7 @ 293 K |
14.7 @ 393 K |
44 |
Er3+/Yb3+:MgWO4 phosphors |
302–623 |
14.4 @ 473 K |
9.72 @ 302 K |
45 |
Er3+/Yb3+:SrWO4 phosphors |
300–518 |
14.9 @ 403 K |
5.3 @ 403 K |
46 |
Er3+/Yb3+:Ca2MgWO6 phosphors |
303–573 |
8.2 @ 453 K |
9.2 @ 303 K |
47 |
Er3+/Yb3+:GdBiW2O9 phosphors |
303–498 |
17.4 @ 450 K |
1.2 @ 303 K |
48 |
Er3+/Yb3+:NaBi(WO4)2 phosphors |
298–373 |
12.7 @ 373 K |
12.4 @ 298 K |
49 |
Er3+/Yb3+:NaY(WO4)2 phosphors |
298–573 |
14.6 @ 523 K |
3.9 @ 523 K |
50 |
Er3+/Yb3+:NaGd(WO4)2 glass |
303–573 |
12.0 @ 542 K |
1.1 @ 303 K |
51 |
Er3+/Yb3+:NaGd(WO4)2 phosphors |
296–623 |
17.3 @ 473 K |
10.0 @ 296 K |
Present work |
Er3+/Yb3+:NaGd(WO4)2 PIG |
298–523 |
17.3 @ 473 K |
10.7 @ 298 K |
Present work |
Er3+/Yb3+:NaGd(WO4)2 glass |
301–623 |
3.29 @ 473 K |
9.96 @ 301 K |
Present work |
Smax and Tmax for Er3+/Yb3+:NaGd(WO4)2 phosphors have been determined to be 16.6 × 10−3 K−1 and 440 K, respectively. These values are closely in agreement with the outcome of the experimental calculation using eqn (8) and (9). Eqn (10) states that for higher sensitivity, a larger pre-exponential factor A and a smaller energy gap between the two levels are needed, whereas eqn (11) demonstrates that for higher Tmax, a large energy gap between the two levels is required.
Additionally, the least amount of temperature variation felt by an optical sensor, or the thermal resolution (uncertainty; T) in measurements of sensing parameters, can be determined using the following equation40
|
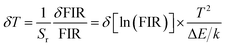 | (12) |
where
δ [ln(FIR)] is the standard deviation of the linear fit to ln(FIR). The obtained value shows that the thermal resolution at room temperature has been improved from 0.50 and 0.49 to 0.42 for glass and phosphors to PIG.
Thermal stability. Highly efficient phosphors in terms of thermal stability are required for the applications of solid-state lighting and temperature sensors. As the chromaticity, color shift, color rendering index, service life, absorption intensity and transition probability can be affected by the increase in temperature, the thermal stability of phosphors becomes a significant parameter.21 The quenching intensity and the spectral shape with rising temperature are two factors that can be used to assess thermal stability. Therefore, temperature dependent UC emission of the Er3+/Yb3+:NaGd(WO4)2 phosphors (296–623 K), PIG (300–573 K) and glass (301–623 K) has been recorded. No change in the shape of the UC emission spectra has been observed. However, thermal quenching with the increase in temperature has been noticed, which is a major challenge of photoluminescence of any material. In actuality, at high temperatures, energy transmission becomes active due to lattice vibrations of the host to rare earth ions. Unexpectedly, the green band of the as-prepared phosphors exhibits a very low luminescence quenching behavior. Their UC emission intensity retains 92% when measured at 423 K of the initial intensity measured at 296 K and it is found to be 84% for the PIG and only 62% for glass. The behavior of the temperature dependent UC emission intensity has also been observed on further increasing the temperature and does not diminish even at 573 K. The UC intensity retains 54%, 65% and 30% of the initial intensity at 573 K for the phosphors, PIG and glass, respectively. Here, an improvement of 10% intensity compared to that of phosphors can be noticed with the PIG, which is 30% compared to that of glass. Thus, PIG can be an effective method to improve thermal quenching at higher temperatures. The temperature scale without much intensity loss has been enlarged using the phosphors as well as PIG (Table 2). The comparative study of the retained luminescence intensity with different phosphors shows that the initial intensity of the present Er3+/Yb3+:NaGd(WO4)2 phosphor reduces by only 8% at 423 K, thereby exhibiting excellent thermal stability.
Table 2 The percentage of UC emission intensity retained at 423 K due to thermal quenching has been compared to other reported works
Phosphors/glass/phosphors in glass |
Retained intensity at 423 K |
References |
RaBa2(PO3)5:Eu2+ phosphors |
89.5% |
52 |
CaMgSi2O6:Cr3+ phosphors |
88.4% |
53 |
NaGd(MoO4)2:Er3+–Yb3+ phosphors |
87% |
40 |
Sr3LiTaO6:Mn4+ phosphors |
76.7% |
54 |
Bi4Ti3O12:Er3+–Yb3+–Al3+ phosphors |
73% |
38 |
YPO4:Ho3+–Tm3+–Yb3+ phosphors |
56% |
55 |
YAG:Ce3+ phosphor-in SiO2–Na2O–B2O3–CaO glass |
70% at 503 K |
56 |
SrGa2S4:Eu2+ phosphor-in SiO2–B2O3–ZnO–Al2O3–K2O glass |
87% at 423 K |
57 |
YAG:Ce3+ phosphor-in SiO2–B2O3–RO (R = Ba, Zn) glass |
93% at 473 K |
58 |
YAG:Ce3+ phosphor-in SiO2–Al2O3–B2O3–ZnO–BaO glass |
93.6% at 423 K and 88.6% at 473 K |
59 |
NaGd(WO4)2: Er3+/Yb3+ phosphors |
92% at 423 K |
54% at 573 K |
Present phosphors |
NaGd(WO4)2: Er3+/Yb3+ phosphor-in TeO2–WO3–ZnO–TiO2 glass |
84% at 423 K |
65% at 573 K |
Present PIG |
TeO2–WO3–ZnO–TiO2: Er3+/Yb3+ glass |
62% at 423 K |
30% at 573 K |
Present glass |
Again, the activation energy has been determined using the Arrhenius equation,60,61
|
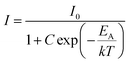 | (13) |
where the luminescence intensities
I0 and
I are defined at initial temperature
T0 and temperature
T (K),
EA stands for the activation energy, ‘
C’ is a constant and ‘
k’ signifies the Boltzmann constant (8.629 × 10
−5 eV K
−1). The calculated activation energies (
EA) are ∼0.29 eV for the phosphors, ∼0.14 eV for PIG and ∼0.21 eV for the glass {
Fig. 4(e)}. The probable non-radiative transition occurring per unit time (
α) can be estimated by the relation
62 |
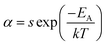 | (14) |
where
s is a constant. It becomes clear that higher activation leads to less non-radiative transition.
4. Conclusion
Er3+/Yb3+:NaGd(WO4)2 phosphors have been successfully synthesized and phosphors in glass at various concentrations of phosphors have been developed. The UC emission intensity of Er3+ doped NaGd(WO4)2 phosphors has been enhanced ∼7 times with the addition of Yb3+ ions. The prepared phosphors have been made applicable for security purposes. The intensity of the UC emission from PIG rises with the increase in phosphor content. No significant improvement or deterioration has been observed in the absolute and relative sensitivities of PIG as compared to the optimized phosphor. However, the thermal resolution at room temperature has been improved significantly in the case of PIG as compared to the phosphor. Also, at higher temperatures, the luminescence quenching in PIGs has been improved up to 10% and 30% as compared to that of the Er3+/Yb3+:NaGd(WO4)2 phosphor and Er3+/Yb3+:TWZTi glass, respectively. Thus, without much luminescence intensity loss, phosphors and the concept of designing PIG both are applicable for optical temperature sensing and solid-state lighting applications.
Conflicts of interest
There are no conflicts to declare.
Acknowledgements
Ms Manisha Prasad is thankful to IIT(ISM), Dhanbad for providing the research fellowship.
References
- S. Lin, M. Chen, Z. Wang, Y. Zhang, R. Yuan, X. Liang, W. Xiang and Y. Zhou, Chem. Eng. J., 2017, 324, 194–202 CrossRef CAS.
- Z. Zhou, N. Zhou, M. Xia, M. Yokoyama and H. B. Hintzen, J. Mater. Chem. C, 2016, 4, 9143–9161 RSC.
- T. Tamaya, A. Ishikawa, T. Ogawa and K. Tanaka, Phys. Rev. Lett., 2016, 116, 016601 CrossRef CAS PubMed.
- W. Ying, Y. Mao, X. Wang, Y. Guo, H. He, Z. Ye, S. T. Lee and X. Peng, ChemSusChem, 2017, 10, 1346–1350 CrossRef CAS PubMed.
- J. Zhong, D. Chen, Y. Zhou, Z. Wan, M. Ding and Z. Ji, J. Eur. Ceram. Soc., 2016, 36, 1705–1713 CrossRef CAS.
- S. Hu, H. Cao, X. Wu, S. Zhan, Q. Wu, Z. Tang and Y. Liu, J. Nanomater., 2016, 2016, 1–9 Search PubMed.
- A. Kumari, A. K. Soni and V. K. Rai, Mater. Focus, 2016, 5, 187–194 CrossRef CAS.
- B. S. Richards, Sol. Energy Mater. Sol. Cells, 2006, 90, 2329–2337 CrossRef CAS.
- Y. Yang, C. Mi, F. Jiao, X. Su, X. Li, L. Liu, J. Zhang, F. Yu, Y. Liu and Y. Mai, J. Am. Ceram. Soc., 2014, 97, 1769–1775 CrossRef CAS.
- S. Wu, Y. Ning, J. Chang and S. Zhang, J. Lumin., 2013, 143, 492–497 CrossRef CAS.
- B. Chen and F. Wang, Trends Chem., 2020, 2, 427–439 CrossRef CAS.
- W. J. Chung and Y. H. Nam, ECS J. Solid State Sci. Technol., 2020, 9, 016010 CrossRef.
- J. Zhong, D. Chen, W. Zhao, Y. Zhou, H. Yu, L. Chen and Z. Ji, J. Mater. Chem. C, 2015, 3, 4500–4510 RSC.
- J. Huang, X. Hu, J. Shen, D. Wu, C. Yin, R. Xiang, C. Yang, X. Liang and W. Xiang, CrystEngComm, 2015, 17, 7079–7085 RSC.
- P. Zeng, Z. Cao, Y. Chen and M. Yin, J. Rare Earths, 2017, 35, 783–786 CrossRef CAS.
- Q. Meng, L. Chen, S. Zhang, L. Huang, R. Lei, S. Zhao and S. Xu, J. Lumin., 2019, 216, 116727 CrossRef CAS.
- A. Durairajan, D. Balaji, K. Kavi Rasu, S. Moorthy Babu, M. A. Valente, D. Thangaraju and Y. Hayakawa, J. Lumin., 2016, 170, 743–748 CrossRef CAS.
- J. Liao, L. Nie, Q. Wang, S. Liu, H. R. Wen and J. Wu, RSC Adv., 2016, 6, 35152–35159 RSC.
- Upconverting Nanoparticles: From Fundamentals to Applications, ed. V. K. Rai, John Wiley & Sons, 2022 Search PubMed.
- H. Wang, G. Jia, F. Yang, Y. Wei, Z. You, Y. Wang, J. Li, Z. Zhu, X. Lu and C. Tu, Appl.
Phys. B, 2006, 83, 579–585 CrossRef CAS.
- Q. Meng, L. Chen, S. Zhang, L. Huang, R. Lei, S. Zhao and S. Xu, J. Lumin., 2019, 216, 116727 CrossRef CAS.
- N. Yuan, D. Y. Liu, X. C. Yu, H. X. Sun, C. G. Ming, W. H. Wong, F. Song, D. Yu, E. Y. Pun and D. L. Zhang, Mater. Lett., 2018, 218, 337–340 CrossRef CAS.
- V. Kesarwani and V. K. Rai, J. Appl. Phys., 2022, 132, 113102 CrossRef CAS.
- S. Karlsson, L. G. Bäck, P. Kidkhunthod, K. Lundstedt and L. Wondraczek, Opt. Mater. Express, 2016, 6, 1198–1216 CrossRef CAS.
- A. Marzuki and D. E. Fausta, IOP Conf. Ser.: Mater. Sci. Eng., IOP Publishing, 2019, vol. 578, No. 1, p. 012043 Search PubMed.
- M. Azam and V. K. Rai, Solid State Sci., 2017, 66, 7–15 CrossRef CAS.
- J. Huang, L. Zhang, L. Xia, X. Shen, W. Wei and W. You, Opt. Mater., 2020, 108, 110387 CrossRef CAS.
- B. D. Cullity, Answers to problems: Elements of X-ray diffraction, Addison-Wesley Publishing Company, 1978 Search PubMed.
- S. Pattnaik and V. K. Rai, Mater. Res. Bull., 2020, 125, 110761 CrossRef CAS.
- R. D. Shannon, Acta Crystallogr., Sect. A: Cryst. Phys., Diffr., Theor. Gen. Crystallogr., 1976, 32, 751–767 CrossRef.
- F. Ben Bacha, K. Guidara, M. Dammak and M. Megdiche, J. Mater. Sci. Mater. Electron., 2017, 28, 10630–10639 CrossRef CAS.
- K. K. Rasu, D. Balaji and S. M. Babu, J. Lumin., 2016, 170, 547–555 CrossRef CAS.
- M. Prasad and V. K. Rai, Methods Appl. Fluoresc., 2022, 10, 034004 CrossRef PubMed.
- C. L. Jia, S. Li and X. X. Song, Appl. Phys. A, 2017, 123, 1–7 CrossRef.
- N. Zhang, D. Chen, F. Niu, S. Wang, L. Qin and Y. Huang, Sci. Rep., 2016, 6, 26467 CrossRef CAS PubMed.
- M. Prasad and V. K. Rai, J. Alloys Compd., 2020, 837, 155289 CrossRef CAS.
- Y. Lei, H. Song, L. Yang, L. Yu, Z. Liu, G. Pan, X. Bai and L. Fan, J. Chem. Phys., 2005, 123, 174710 CrossRef PubMed.
- S. Pattnaik and V. K. Rai, Methods Appl. Fluoresc., 2022, 10, 034002 CrossRef PubMed.
- W. Ran, G. Sun, X. Ma, Z. Zhang and T. Yan, Dalton Trans., 2022, 51, 8749–8756 RSC.
- S. Pattnaik, M. Mondal, L. Mukhopadhyay, S. Basak, V. K. Rai, R. Giri and V. Singh, New J. Chem., 2022, 46, 10897–10906 RSC.
- M. Haouari, A. Maaoui, N. Saad and A. Bulou, Sens. Actuators, A, 2017, 261, 235–242 CrossRef CAS.
- A. Kumar Soni, V. Kumar Rai and S. Kumar, Sens. Actuators, B, 2016, 229, 476–482 CrossRef CAS.
- M. Haouari, A. Maaoui, N. Saad and A. Bulou, Sens. Actuators, A, 2017, 261, 235–242 CrossRef CAS.
- L. Xu, J. Liu, L. Pei, Y. Xu and Z. Xia, J. Mater. Chem. C, 2019, 7, 6112–6119 RSC.
- M. Prasad and V. K. Rai, Methods Appl. Fluoresc., 2022, 10, 034004 CrossRef PubMed.
- A. Pandey, V. K. Rai, V. Kumar, V. Kumar and H. C. Swart, Sens. Actuators, B, 2015, 209, 352–358 CrossRef CAS.
- Y. Jiang, Y. Tong, S. Chen, W. Zhang, F. Hu, R. Wei and H. Guo, Chem. Eng. J., 2021, 413, 127470 CrossRef CAS.
- S. Dutta, S. Som and T. M. Chen, ACS.Omega, 2018, 3, 11088–11096 CrossRef CAS PubMed.
- J. Gao, X. Ren, K. Yang, S. Zhao, L. Huang and S. Xu, Optik, 2021, 242, 167280 CrossRef CAS.
- Z. Zou, T. Wu, H. Lu, Y. Tu, S. Zhao, S. Xie, F. Han and S. Xu, RSC Adv., 2018, 8, 7679–7686 RSC.
- Q. Meng, L. Chen, S. Zhang, L. Huang, R. Lei, S. Zhao and S. Xu, J. Lumin., 2019, 216, 116727 CrossRef CAS.
- Q. Zhang, X. Wang and Y. Wang, J. Alloys Compd., 2021, 886, 161217 CrossRef CAS.
- D. Wen, H. Liu, Y. Guo, Q. Zeng, M. Wu and R. S. Liu, Angew. Chem., Int. Ed. Engl., 2022, 61, e202204411 CAS.
- Y. Yang, L. Fu, X. Ren, Y. Zhu, J. Zhu, Y. Wu, X. Pu and Y. Zhang, J. Lumin., 2021, 238, 118234 CrossRef CAS.
- L. Mukhopadhyay and V. K. Rai, Mater. Res. Bull., 2020, 121, 110628 CrossRef CAS.
- Y. Liang, Y. Zhang, H. Yang, Y. Zhang, J. Zhang, L. Wang, X. Liang, J. Zhong and W. Xiang, Appl. Phys. Lett., 2021, 119, 033303 CrossRef CAS.
- Y. H. Kim, P. Arunkumar and W. B. Im, Ceram. Int., 2015, 41, 5200–5204 CrossRef CAS.
- Y. K. Lee, J. S. Lee, J. Heo, W. B. Im and W. J. Chung, Opt. Lett., 2012, 37, 3276–3278 CrossRef CAS PubMed.
- X. Zhang, J. Yu, J. Wang, B. Lei, Y. Liu, Y. Cho, R. Xie, H. Zhang, Y. Li, Z. Tian, Y. Li and Q. Su, ACS Photonics, 2017, 4, 986–995 CrossRef CAS.
- M. Janulevicius, P. Marmokas, M. Misevicius, J. Grigorjevaite, L. Mikoliunaite, S. Sakirzanovas and A. Katelnikovas, Sci. Rep., 2016, 6, 1–12 CrossRef PubMed.
- V. Kesarwani and V. K. Rai, Opt. Laser Technol., 2022, 146, 107535 CrossRef CAS.
- J. Chen, N. Zhang, C. Guo, F. Pan, X. Zhou, H. Suo, X. Zhao and E. M. Goldys, ACS Appl. Mater. Interfaces, 2016, 8, 20856–20864 CrossRef CAS PubMed.
|
This journal is © The Royal Society of Chemistry 2023 |
Click here to see how this site uses Cookies. View our privacy policy here.