DOI:
10.1039/D3RA02504G
(Paper)
RSC Adv., 2023,
13, 17536-17544
Digital light processing 3D printing of dynamic magneto-responsive thiol-acrylate composites†
Received
15th April 2023
, Accepted 5th June 2023
First published on 9th June 2023
Abstract
Additive manufacturing is one of the most promising processing techniques for fabricating customized 3D objects. For the 3D printing of functional and stimuli-triggered devices, interest is steadily growing in processing materials with magnetic properties. Synthesis routes for magneto-responsive soft materials typically involve the dispersion of (nano)particles into a non-magnetic polymer matrix. Above their glass transition temperature, the shape of such composites can be conveniently adjusted by applying an external magnetic field. With their rapid response time, facile controllability, and reversible actuation, magnetically responsive soft materials can be used in the biomedical field (e.g. drug delivery, minimally invasive surgery), soft robotics or in electronic applications. Herein, we combine the magnetic response with thermo-activated healability by introducing magnetic Fe3O4 nanoparticles into a dynamic photopolymer network, which undergoes thermo-activated bond exchange reactions. The resin is based on a radically curable thiol-acrylate system, whose composition is optimized towards processability via digital light processing 3D printing. A mono-functional methacrylate phosphate is applied as a stabilizer to increase the resins' shelf life by preventing thiol-Michael reactions. Once photocured, the organic phosphate further acts as a transesterification catalyst and activates bond exchange reactions at elevated temperature, which render the magneto-active composites mendable and malleable. The healing performance is demonstrated by recovering magnetic and mechanical properties after the thermally triggered mending of 3D-printed structures. We further demonstrate the magnetically driven movement of 3D-printed samples, which gives rise to the potential use of these materials in healable soft devices activated by external magnetic fields.
1. Introduction
Since the beginning of the 1980s, 3D printing has become a fast-emerging technology, which has been successfully implemented world-wide in academic and several industrial sectors.1 Multi-functional objects with complex and customized architectures are easily formed into physical objects by layer-by-layer deposition of materials directly from computer-aided design without molds and heavy machining.2 Due to freedom in component design and production, the 3D printing technology is applied for fabricating parts used in industrial machines, consumer products (electronics), motor vehicles, and aerospace or medical (dental) sectors.3 However, significant progress has been achieved by introducing a shape-morphing capability into 3D-printed objects, also known as 4D printing. Initiated and termed by researchers from MIT4 in collaboration with Stratasys, Inc., the fourth dimension of 3D printed objects offers the ability to switch their geometric configuration in response to variations of the surrounding environment. This unlocks a new toolbox of application areas in 3D printing.5 Numerous external stimuli can be applied such as light,6,7 heat,6,8 temperature,9 solvent,10 pH value,11 electric field,12 magnetic field13 or humidity.14 Due to their multi-functional behavior (e.g. reconfigurable structure, shape memory effect, actuation, sensing), these composites can be used in a broad variety of applications15 including soft robotics,16 shape memory structures,17 sensors and actuators.18
In this context, great effort has been devoted to develop magneto-active materials for functional composites.19 By exposure to an external magnetic field, these materials undergo mechanical and rheological changes (e.g. damping, elasticity), and shape changes. Magneto-responsive materials are found in the form of a fluid, a gel or a solid.20 Solid magneto-active materials consist of a soft polymeric matrix, in which inorganic magnetic particles (e.g. ferrite particles Fe3O4, maghemite γ-Fe2O3 or neodymium–iron–boron particles NdFeB)21 are dispersed. Prominent examples of polymers are elastomers,22 plastomers23 and foams.24 A typical behavior of magneto-responsive elastomers (MREs) under the application of a magnetic field is their change of modulus. However, the range of applications is limited due to small magnetically induced actuation strain. Various additive manufacturing techniques, such as direct ink writing (DIW) and fused filament fabrication (FFF) have been employed to fabricate polymers containing high loads of magnetic fillers.25,26 Colorado and Restrepo demonstrated the printing of an epoxy resin loaded with magnetite particles up to 41 wt% via DIW. Results on compressive strength and ductility of tested printed composites showed their applicability in structural components.27 In 2018, Zhao et al. reported the 3D printing of elastomer composites filled with ferromagnetic microparticles prepared by direct ink writing. More precisely, they were able to program ferromagnetic domains in complexly designed 3D-printed materials by applying a magnetic field directly to the dispensing nozzle during the printing process.28
Recently, Rodionova et al. incorporated magnetic ferrite particles into polylactic acid and produced objects with tunable magnetic properties via FFF. Results set up the stage for the additive manufacturing of composites with controlled magnetic anisotropy, as well as, for achieving a spatial distribution of nanofillers in a non-magnetic polymeric matrix.29 One of the salient feature of DIW is that inks with high loads of magnetic fillers are applicable.25 Moreover, previous research showed that both DIW and FFF can be used to produce fast responding actuators30 as well as permanent magnets.31 Despite the mentioned advantages, objects printed by these techniques suffer from poor resolution. In addition, fillers may be not homogeneously dispersed, which leads to inhomogeneous magnetic response. In addition, high temperatures required during the FFF process may affect the fillers negatively.32
Another promising printing approach is based on vat photopolymerization, in which the liquid photo-reactive resin is mixed with magnetic fillers. The method known as digital light processing (DLP) 3D printing is based on a layer-by-layer curing of the resin upon irradiation with a suitable light source. Compared to DIW and FFF, DLP offers several advantages, as it provides higher resolution and build speed, and it operates at room temperature. However, vat photopolymerization presents some drawbacks in terms of the maximum load of fillers in the resin. The photopolymerization process may be negatively affected by an increase in the content of fillers, which compromise on the optical properties of the resin. In addition, the stability and sedimentation of dispersed fillers in the liquid resin plays a vital role in achieving 3D-printed objects with a homogeneous magnetic response. In 2019, Lantean et al. developed magneto-responsive photopolymers based on a urethane-acrylate resin filled with Fe3O4 nanoparticles. Mechanical properties were tuned by adding butyl acrylate, which reduced the resin's viscosity, and magnetic properties were tailored by loading magnetic filler up to 6 wt%.33 In subsequent work, the authors studied the magnetically driven self-assembly of Fe3O4 nanoparticles into chain-like structures within the vat. The rotation and kinetics of chain formation depended on the viscosity, weight percentage of nanofillers, the intensity of the external magnetic field, and its application time. By applying magnetic fields of varying direction and intensity during the printing process, the authors could control the orientation and the length of the magnetic chains in each printed layer, which was exploited for the fabrication of magnetically driven devices.34
Recently, our group demonstrated the possibility to print soft magneto-active objects using thiol-click photopolymers as matrix. In particular, by advancing from pure acrylate to thiol-acrylate systems, we overcome shrinkage stress, oxygen inhibition and low monomer conversion of the filled photopolymers. Soft and flexible polymer composites were obtained by DLP 3D printing with 6 wt% of Fe3O4 nanoparticles at a reasonable build time.35
Herein, we extended the functionality of 3D printable magneto-responsive thiol-acrylate composites by introducing an additional healing and re-shaping function. In particular, we exploited thermo-activated bond exchange reactions following catalyzed transesterification to induce a material flow above the networks topological freezing temperature. We optimized the composition of the photocurable formulation in terms of viscosity and stability and were able to 3D print objects with a Fe3O4 loading of up to 6 wt%. As a proof of concept, magneto-responsive structures were DLP 3D printed, which were thermally healed and could be conveniently reshaped and activated by applying an external magnetic field.
2. Results and discussion
2.1. Network design and optimization of the photocurable resin containing Fe3O4 nanofillers
To prepare magnetically responsive dynamic photopolymers, we used our recently reported thiol-acrylate system, which has been loaded with magnetic Fe3O4 nanoparticles (d50 = 50 nm) as a basis.36
The system was composed of hydroxy-2-phenoxypropyl acrylate (HPPA) and glycerol 1,3-diglycerolate diacrylate (GDGDA) and provided ample –OH groups for the thermo-activated transesterification reaction (Fig. 1).
 |
| Fig. 1 Monomers, transesterification catalyst, photoinitiator and iron oxide nanoparticles (NPs) used in magneto-responsive resins processed via DLP 3D printing. | |
Trimethylolpropane tri(3-mercaptopropionate) (TMPMP) was added as a thiol crosslinker to switch the curing mechanism of pure acrylates following a chain-growth mechanism to a mixed mode one including chain-growth and step-growth reactions. In previous work, we further employed a mono-functional methacrylate phosphate as stabilizer to prevent thiol-Michael reactions and to increase the stability of the photo-curable resins during storage and printing. In another study we showed that this stabilizer is also able to catalyze transesterification reactions due to its Brønsted acidity.35 Herein, we exploited this feature to print magneto-active composites with additional functions such as thermo-activated mendability.
We varied the filler loading between 0 and 6 wt%, as previous work revealed that filler loadings >6 wt% negatively affect the cure kinetics and stability of the photocurable thiol-acrylate resins. In the present study, we additionally varied the content of the low viscous HPPA and the thiol crosslinker to reduce the viscosity of the formulations and increase the resins' stability, respectively (Table 1).
Table 1 Molar composition of the thiol-acrylate formulations under investigation
Compound |
Resin-1 (ref. 36) |
Resin-2 |
HPPA |
50 mol% |
65 mol% |
GDGDA |
25 mol% |
25 mol% |
TMPMP |
25 mol% |
10 mol% |
BAPO |
2 wt% |
5 wt% |
Miramer A99 |
5 wt% |
5 wt% |
As viscosity and shelf life of the resin are crucial parameters in vat photopolymerization 3D printing, we determined the viscosity of the new resin formulation (resin-2) with varying content of magnetic nanofillers (0–6 wt%) (Fig. 2b) and compared it with our previously published system (Fig. 2a). The measurements were performed over a prolonged time (15 min) to further get an idea of the resins' stability. Rheological data reveal that the viscosity increases with rising filler concentration but does not exceed 1000 mPa s, which is still within the range (250 and 5000 mPa s) of 3D DLP printable resins.37
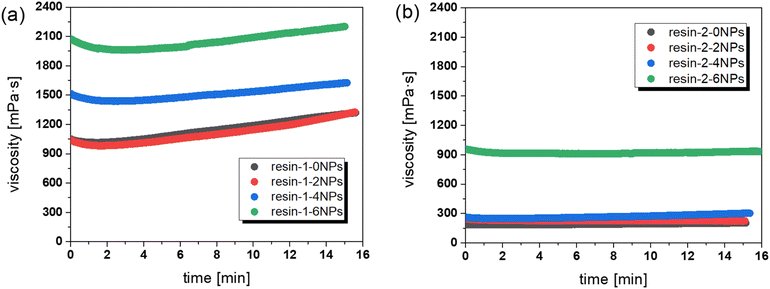 |
| Fig. 2 Stability of the resins filled with up to 6 wt% of nanofiller at a constant shear of 300 s−1: (a) published thiol-acrylate vitrimer system (resin-1)36 and (b) modified thiol-acrylate vitrimer system (resin-2). | |
By increasing the HPPA content from 50 mol% to 65 mol% and by decreasing the thiol content from 25 mol% to 10 mol%, the viscosity of the filled and unfilled resins could be significantly reduced. In particular, for the unfilled resin, a decrease in the viscosity from 1320 to 203 mPa s was observed. Interestingly, the addition of up to 4 wt% of nanofillers did not significantly affect the viscosity compared to the unfilled system (302 versus 203 mPa s). In contrast, the presence of 6 wt% of nanofillers resulted in a rapid increase in viscosity (934 mPa s) which is also reported for other systems.38 Due to the reduction of the thiol content in resin-2, the stability of the filled and unfilled compounds could be significantly improved compared to the previously studied resin systems.
In contrast to previous work, we additionally increased the BAPO concentration from 2 to 5 wt% to accelerate the cure rate. BAPO as a classical radical photoinitiator absorbs in the visible region (λ = 360–440 nm) and forms up to four radicals by undergoing two subsequent Norrish type I reactions across the σ bond between the carbonyl groups and the phosphor atom.39 Since the formed radicals rapidly initiate radical-mediated polymerization reactions, BAPO is a commonly used photoinitiator for the photocuring of acrylate, thiol-acrylate and interpenetrating epoxy-acrylate resins.40
We studied the cure kinetics by Fourier-transform infrared (FTIR) spectroscopy, in which the C
C–H stretching band of the acrylate groups was monitored at 1616 and 1634 cm−1 upon exposure at 405 nm. It should be pointed out that the IR signal at 2575 cm−1, which belongs to the mercapto groups, was too weak to consider for quantitative evaluation. The IR spectrum of resin-2-0NPs (prior to and after light exposure) is provided in Fig. S1 in ESI.† We studied the acrylate conversion versus the filler content as Fe3O4 nanoparticles absorb light in the UV and visible region (internal filter effect). The results clearly show that the presence of the nanofillers slows down the reaction kinetics, albeit at a lower extend as expected (Fig. 3). For the unfilled resin, the monomer conversion amounted to 85% after 64 s of light exposure, whilst it decreased to 76% for the system containing 6 wt% filler.
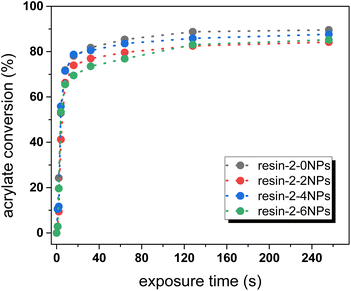 |
| Fig. 3 Monitoring the acrylate conversion of resin-2 as a function of the concentration of Fe3O4 nanoparticles. The lines are a guide for the eye. | |
2.2 3D printing and material characterization
Due to the decent cure rates, it was possible to DLP 3D print test specimen for dynamic mechanical thermal analysis (DMTA) and stress relaxation studies. DMTA was used to study the thermo-mechanical properties of the 3D-printed samples filled with nanofillers in the range of 0 to 6 wt% (Fig. 4). When nanoparticles were added to the photocurable formulation, the Tg of the printed composites increased from 45 °C (resin-2-0NPs) to 51 °C (resin-2-6NPs). In previous works, a reduction of the Tg for magneto-responsive nanocomposites based on urethane-acrylate resins was observed and explained by the decrease of the monomer conversion due to competition between the photoinitiator and nanofiller in absorbing radiation during the printing process.33
 |
| Fig. 4 (a) Loss factor (tan δ) and (b) storage modulus (E′) versus temperature as obtained from DMA measurements of resin-2 filled with nanofillers up to 6 wt%. | |
Interestingly, here we observed a slight increase in the Tg value by introducing nanofillers into the system. On the one hand, this can be explained by the presence of the thiol crosslinkers, which lead to higher carbon double bond conversion due to the mixed mode curing mechanism. Thiols act as chain-transfer agents for the chain-growth polymerization of acrylates and reduce the kinetic chain length. This results in lower diffusion limitations of the reacting monomers and a shift of the gel point to higher conversions.41
On the other hand, coordinative bonds might be formed between the Fe3O4 nanoparticles and the unreacted thiol groups, which anchor the filler to the photopolymer matrix.35 In the presence of the organic phosphate, the photopolymer composites are able to undergo thermo-activated dynamic bond exchange reactions across the functional –OH and ester groups (transesterification). The change in the viscoelastic properties was studied by rheometer experiments.
Fig. 5 provides the time-dependent evolution of the relaxation modulus for resin-2 with varying concentration of nanofillers at 180 °C. While temperature and catalyst in the thiol-acrylate systems have an impact on the stress relaxation kinetics,36 the results clearly indicate that the addition of nanofillers (and the related slight shift to higher Tg values) does not significantly affect the bond exchange rate within the investigated range of filler concentration.
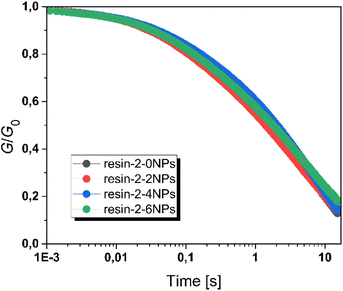 |
| Fig. 5 Normalized stress-relaxation curves (obtained at 180 °C) of resin-2 with varying filler concentration. | |
2.3 Thermal healing and magnetic properties
Although resin-2 was printed with up to 6 wt% of nanofillers, we performed further printing studies with resin-2-4NPs to avoid premature gelation during the printing process. To demonstrate the magnetic response of the filled thiol-acrylate vitrimer network, a printed stripe was fixed on one side with tweezers. As is shown in Fig. 6a, by applying an external magnetic field (1.24 T), the stick containing 4 wt% of nanofillers could be activated even without physical contact between the magnet and the stick. Moreover, we printed a stickman where every single part was activated separately. It should be noted that we were not able to print more complex structures as premature gelation occurred at a filler concentration of 4 wt% upon prolonged printing time. In addition, the viscosity of the filled resin was too high to print objects with feature sizes lower than 1 mm.
 |
| Fig. 6 Activation of objects DLP 3D-printed with resin-2 containing 4 wt% of Fe3O4 nanoparticles. | |
Thermal healing of magneto-responsive photopolymers was demonstrated on 3D-printed dumbbell test specimens. An appropriate aligning of thin broken test bars is challenging and thus, test bars with a circular-shaped hole in the center were printed together with the circular-shaped counterpart (Fig. 7b).
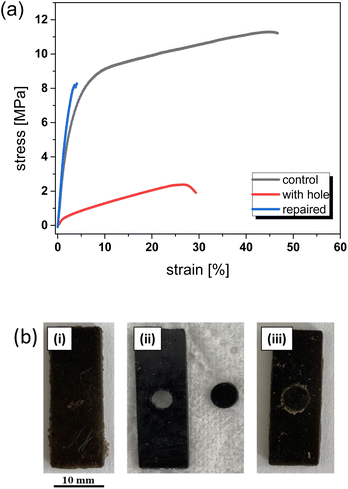 |
| Fig. 7 (a) Stress–strain curves of test specimens DLP 3D-printed with resin-2 containing 4 wt% of Fe3O4 nanoparticles prior to and after a thermal healing at 180 °C for 4 h, (b) DLP 3D-printed test specimen used for thermal healing experiments: (i) DLP 3D-printed control sample. (ii) DLP 3D-printed test specimen with a circular-shaped hole in the center and DLP 3D-printed circular shaped counterpart. (iii) Test specimen after fitting the circular-shaped counterpart in the hole and subsequent thermal treatment. | |
For the healing step, the disc was fitted in the hole and a thermal healing/welding at 180 °C for 4 h was carried out. Due to the macroscopic reflow, the boundaries between the two parts efficiently welded together and the original tensile strength (defect-free bar) could be partly recovered. The defect-free control samples (Fig. 7b) resulted in a tensile strength of 11.2 MPa and an ultimate elongation of 46%. In contrast, the tensile strength of the sample, which contained the hole, was drastically reduced to 2.3 MPa with an elongation of 27.9%.
Due to the thermally triggered repair process, the tensile strength could be regained to a large extend (8.1 MPa), whilst the elongation (3.9%) was even lower than the value measured for the sample containing the hole. Whilst the test bars were breaking nearly in the center (Fig. S2 in ESI†), the fracture behavior changed. Since this behavior was not observed in our previous studies on the healing of unfilled dynamic photopolymer networks, we assume that this change in damage behavior is mainly related to the fillers present in the dynamic thiol-acrylate composite.
Along with mechanical performance, the magnetic properties were determined prior to and after thermally induced welding. Room-temperature hysteresis loops of 3D-printed control and welded samples are shown in Fig. 8. The hysteresis curves and the magnetic characteristics of both types of samples (control and welded) are comparable. The coercivities are around 80 Oe (control sample) and 75 Oe (welded sample). Due to the low coercivity at room temperature, one can assume a superparamagnetic behavior, which does not change due to the thermo-activated healing process. The results indicate that the magnetic properties are not affected by the thermal healing step, which has been carried out at 180 °C for 4 h.
 |
| Fig. 8 Room temperature hysteresis loops of 3D-printed magnetic composites from resin-2-4NPs prior to and after welding at 180 °C for 4 h. | |
3. Experimental
3.1 Material and chemicals
2-Hydroxy-2-phenoxypropyl acrylate (HPPA), glycerol 1,3-diglycerolate diacrylate (GDGDA), trimethylolpropane tri(3-mercaptopropionate) (TMPMP), Fe(II,III) oxide nanoparticles (d50 = 50 nm), and phenylbis(2,4,6-trimethylbenzoyl) phosphine oxide (BAPO) were purchased from Sigma-Aldrich. Miramer A99 was used as stabilizer and transesterification catalyst, and was received from Miwon Speciality Chemical (Korea). All chemicals were used without further purification.
3.2 Preparation of resins
3.2.1 Preparation of resin-1. HPPA (50 mol%) was mixed with GDGDA (25 mol%) and 5 wt% Miramer A99. 0.05 wt% Sudan II was added and the formulation was ultra-sonicated until the photoabsorber was dissolved. 3 wt% phenylbis(2,4,6-trimethylbenzoyl)phosphine oxide and TMPMP (25 mol%) were added and dissolved by stirring the formulation at room temperature for 30 min.
3.2.2 Preparation of resin-2. HPPA (65 mol%) was mixed with GDGDA (25 mol%) by means of a magnetic stirrer at room temperature. Subsequently, 5 wt% of phenylbis(2,4,6-trimethylbenzoyl) phosphine oxide and 5 wt% Miramer A99 were added and the formulations were ultra-sonicated at 40 °C until all components were dissolved. After cooling to room temperature, TMPMP (10 mol%) and Fe3O4 nanoparticles were added. The prepared formulation was manually stirred with a spatula and sonicated for 1 min at room temperature.
3.3 Characterization
Fourier transform infrared spectroscopy was studied on a Vertex 70 spectrometer (Bruker, USA). Spectra were taken in transmittance mode over a wavenumber range from 4000 to 700 cm−1. All spectra were accumulated from 16 scans at a resolution of 4 cm−1 and the absorption peak areas were calculated with OPUS software. For sample preparation, 1.5 μL of resin was drop cast between two CaF2 discs. Samples were irradiated with a light emitting diode lamp (zgood® wireless LED curing lamp) with a power density of 3.3 mW cm−2 (λ = 420–450 nm). The conversion at corresponding exposure times were calculated with OPUS software by evaluating the decrease of the characteristic acrylate infrared absorption band at 1620–1636 cm−1.
The viscosity of the formulations was determined with a modular compact rheometer MCR 102 from Anton Paar (Austria) in a parallel plate set up with a 25 mm diameter. Each measurement was carried out with 0.5 mL formulation at room temperature and a shear rate ranging between 0.1 and 300 s−1. The same measuring setup was utilized to determine the stability of the resins by applying a shear rate of 300 s−1 over 14 min.
Dynamic mechanical analysis (DMA) was carried out on a Mettler Toledo DMA/SDTA861e analyzer. Mechanical loss factors (tan
δ) and storage moduli (E′) were monitored over a temperature range from −25 to 70 °C at a heating rate of 3 °C min−1, with a frequency of 1 Hz, in a displacement-controlled mode with maximum amplitude 10 μm and maximum force of 5 N. The glass transition temperature (Tg) was determined by the temperature at the maximum of the loss factor. For sample preparation, test specimen (30 mm × 4 mm × 1 mm) were 3D printed with resin-2 using a commercial printer from Anycubic (China).
Mechanical properties were characterized by a ZwickRoell (Germany) Z1.0 static materials testing machine with a crosshead speed of 250 mm min−1. Tensile tests were performed on 3D printed dumbbell specimens (resin-2 filled with 4 wt% nanoparticles) with the dimensions of 2 × 12.5 × 75 mm.
Stress relaxation experiments were carried out at 180 °C on an Anton Paar Physica MCR 501 rheometer (Austria) with parallel plate geometry. Printed samples (10 × 1 mm) of resin-2 were equilibrated to the set temperature and a specified constant normal force of 20 N was applied for 15 min. Subsequently, 3% step strain was applied and the decreasing stress was recorded over time.
3.4 DLP 3D printing
3D printing was performed on an Anycubic Photo Mono printer (China) with a LED 405 m light source. Two bottom layers were exposed for 24 s, whereas the other layers were illuminated for 5 s. The layer height was set to 50 μm with a building speed of 6 mm s−1 and a retracting speed of 1 mm s−1.
3.5 Thermo-activated healing
Both, the control sample and the defect sample with a hole had dimensions of 30 × 10 × 1.5 mm and were printed together with the corresponding disc (d = 5 mm) via DLP 3D printing using resin-2 filled with 4 wt% of nanofillers. For the thermal healing, the printed disc was fitted inside the hole and the samples were heated to 180 °C for 4 h.
3.6 Welding experiments and magnetic properties
Three discs (10 × 1 mm) were printed with resin-2 containing 4 wt% of nanofillers. Welding of two discs was achieved by pressing the discs over each other and heating at 180 °C for 4 h. Magnetic properties of control and welded samples were measured with a vibrating sample magnetometer (8600 Series VSM System, Lake Shore) at room temperature. The magnetic field was applied perpendicular to the sample surface in a range between ±1 T.
4. Conclusions
In summary, we have prepared DLP 3D printable thiol-acrylate resins containing magneto-active fillers and dynamic covalent bonds, which rendered the cured photopolymers malleable and thermally mendable. Whilst viscosity and Tg increased with rising filler content, the cure kinetics decreased due to the competition between the nanofiller and the photoinitiator resulting in a slight delay in the photopolymerization process. At a concentration of 4 wt% nanofillers, objects with a simple geometry could be DLP 3D printed and their activation by an external magnetic field was demonstrated. Due to thermo-activated transesterifications, the photopolymers were weldable and thermally healable. Both control and welded samples showed supermagnetic behavior after thermal treatment at 180 °C for 4 hours and the magnetic properties did not significantly change during the welding step. In addition, thermal healing of printed test bars was demonstrated giving rise to the versatility and functionality of the developed resin system.
Conflicts of interest
There are no conflicts to declare.
Acknowledgements
The research work was performed within the COMET-Module project “Chemitecture” (project-no. 21647048) at the Polymer Competence Center Leoben GmbH (PCCL, Austria) within the framework of the COMET-program of the Federal Ministry for Transport, Innovation and Technology and the Federal Ministry for Digital and Economic Affairs with contributions by Montanuniversitaet Leoben, Politecnico di Torino, Helios TBLUS and bto-epoxy. Funding is provided by the Austrian Government and the State Government of Styria. In addition, the authors thank David Reisinger (PCCL) for performing stress-relaxation experiments and Walter Alabiso (PCCL) for carrying out the DMA measurements.
References
-
(a) J. Saroia, Y. Wang, Q. Wei, M. Lei, X. Li, Y. Guo and K. Zhang, Int. J. Adv. Manuf. Technol., 2020, 106, 1695–1721 CrossRef;
(b) S. C. Ligon, R. Liska, J. Stampfl, M. Gurr and R. Mülhaupt, Chem. Rev., 2017, 117, 10212–10290 CrossRef CAS PubMed;
(c) M. Hofmann, ACS Macro Lett., 2014, 3, 382–386 CrossRef CAS PubMed;
(d) M. Nadgorny and A. Ameli, ACS Appl. Mater. Interfaces, 2018, 10, 17489–17507 CrossRef CAS PubMed.
-
(a) B. C. Gross, J. L. Erkal, S. Y. Lockwood, C. Chen and D. M. Spence, Anal. Chem., 2014, 86, 3240–3253 CrossRef CAS PubMed;
(b) R. L. Truby and J. A. Lewis, Nature, 2016, 540, 371–378 CrossRef CAS PubMed;
(c) T. D. Ngo, A. Kashani, G. Imbalzano, K. T. Q. Nguyen and D. Hui, Composites, Part B, 2018, 143, 172–196 CrossRef CAS;
(d) B. Cao, N. Boechler and A. J. Boydston, Polymer, 2018, 152, 4–8 CrossRef CAS.
-
(a) W. Associates, Wohlers report 2016. 3D printing and additive manufacturing state of the industry : annual worldwide progress report, Wohlers Associates, Fort Collins (Colo.), 2016 Search PubMed;
(b) N. Shahrubudin, T. C. Lee and R. Ramlan, Procedia Manuf., 2019, 35, 1286–1296 CrossRef.
- S. Tibbits, Archit. Design, 2014, 84, 116–121 CrossRef.
-
(a) A. Ahmed, S. Arya, V. Gupta, H. Furukawa and A. Khosla, Polymer, 2021, 228, 123926 CrossRef CAS;
(b) Z. Zhang, K. G. Demir and G. X. Gu, Int. J. Smart Nano Mater., 2019, 10, 205–224 CrossRef;
(c) M. Y. Khalid, Z. U. Arif, R. Noroozi, A. Zolfagharian and M. Bodaghi, J. Manuf. Process., 2022, 81, 759–797 CrossRef.
- A. Kotikian, R. L. Truby, J. W. Boley, T. J. White and J. A. Lewis, Adv. Mater., 2018, 30, 1706164 CrossRef PubMed.
- H. Yang, W. R. Leow, T. Wang, J. Wang, J. Yu, K. He, D. Qi, C. Wan and X. Chen, Adv. Mater., 2017, 29, 1701627 CrossRef PubMed.
- Z. Ding, C. Yuan, X. Peng, T. Wang, H. J. Qi and M. L. Dunn, Sci. Adv., 2017, 3, e1602890 CrossRef PubMed.
-
(a) A. Lendlein and S. Kelch, Angew. Chem., Int. Ed., 2002, 41, 2034–2057 CrossRef CAS;
(b) D. Crespy and R. M. Rossi, Polym. Int., 2007, 56, 1461–1468 CrossRef CAS.
-
(a) A. S. Gladman, E. A. Matsumoto, R. G. Nuzzo, L. Mahadevan and J. A. Lewis, Nat. Mater., 2016, 15, 413–418 CrossRef PubMed;
(b) J. J. Schwartz and A. J. Boydston, Nat. Commun., 2019, 10, 791 CrossRef CAS PubMed;
(c) H. Meng and G. Li, Polymer, 2013, 54, 2199–2221 CrossRef CAS;
(d) Y. Dong, S. Wang, Y. Ke, L. Ding, X. Zeng, S. Magdassi and Y. Long, Adv. Mater. Technol., 2020, 5, 2000034 CrossRef CAS.
- R. Marcombe, S. Cai, W. Hong, X. Zhao, Y. Lapusta and Z. Suo, Soft Matter, 2010, 6, 784–793 RSC.
-
(a) Z. Varga, G. Filipcsei, A. Szilágyi and M. Zrínyi, Macromol. Symp., 2005, 227, 123–134 CrossRef CAS;
(b) M. Hossain, D. K. Vu and P. Steinmann, Arch. Appl. Mech., 2015, 85, 523–537 CrossRef;
(c) G. Li, X. Chen, F. Zhou, Y. Liang, Y. Xiao, X. Cao, Z. Zhang, M. Zhang, B. Wu, S. Yin, Y. Xu, H. Fan, Z. Chen, W. Song, W. Yang, B. Pan, J. Hou, W. Zou, S. He, X. Yang, G. Mao, Z. Jia, H. Zhou, T. Li, S. Qu, Z. Xu, Z. Huang, Y. Luo, T. Xie, J. Gu, S. Zhu and W. Yang, Nature, 2021, 591, 66–71 CrossRef CAS PubMed.
-
(a) M. Sitti, Nat. Rev. Mater., 2018, 3, 74–75 CrossRef;
(b) A. K. Bastola and M. Hossain, Mater. Des., 2021, 211, 110172 CrossRef;
(c) L. V. Nikitin, D. G. Korolev, G. V. Stepanov and L. S. Mironova, J. Magn. Magn. Mater., 2006, 300, e234–e238 CrossRef CAS;
(d) K. Zimmermann, V. A. Naletova, I. Zeidis, V. A. Turkov, E. Kolev, M. V. Lukashevich and G. V. Stepanov, J. Magn. Magn. Mater., 2007, 311, 450–453 CrossRef CAS;
(e) P. V. Komarov, P. G. Khalatur and A. R. Khokhlov, Polym. Adv. Technol., 2021, 32, 3922–3933 CrossRef CAS;
(f) L. Vítková, L. Musilová, E. Achbergerová, R. Kolařík, M. Mrlík, K. Korpasová, L. Mahelová, Z. Capáková and A. Mráček, Int. J. Mol. Sci., 2022, 23, 9633 CrossRef PubMed.
- A. K. Bastola, N. Rodriguez, M. Behl, P. Soffiatti, N. P. Rowe and A. Lendlein, Mater. Des., 2021, 202, 109515 CrossRef CAS.
- M. Mrinalini and S. Prasanthkumar, ChemPlusChem, 2019, 84, 1103–1121 CrossRef CAS PubMed.
-
(a) S. Li, H. Bai, R. F. Shepherd and H. Zhao, Angew. Chem., Int. Ed., 2019, 58, 11182–11204 CrossRef CAS PubMed;
(b) D. Schönfeld, D. Chalissery, F. Wenz, M. Specht, C. Eberl and T. Pretsch, Molecules, 2021, 26, 522 CrossRef PubMed;
(c) L. Hines, K. Petersen, G. Z. Lum and M. Sitti, Adv. Mater., 2017, 29, 1603483 CrossRef PubMed.
- X. Kuang, D. J. Roach, J. Wu, C. M. Hamel, Z. Ding, T. Wang, M. L. Dunn and H. J. Qi, Adv. Funct. Mater., 2019, 29, 1805290 CrossRef.
-
(a) X. Liu, J. Liu, S. Lin and X. Zhao, Mater. Today, 2020, 36, 102–124 CrossRef CAS;
(b) M. Cianchetti, C. Laschi, A. Menciassi and P. Dario, Nat. Rev. Mater., 2018, 3, 143–153 CrossRef;
(c) A. Lendlein and O. E. C. Gould, Nat. Rev. Mater., 2019, 4, 116–133 CrossRef;
(d) Y. S. Lui, W. T. Sow, L. P. Tan, Y. Wu, Y. Lai and H. Li, Acta Biomater., 2019, 92, 19–36 CrossRef CAS PubMed.
-
(a) R. Ahamed, S.-B. Choi and M. M. Ferdaus, J. Intell. Mater. Syst. Struct., 2018, 29, 2051–2095 CrossRef;
(b) Q. Cao, Q. Fan, Q. Chen, C. Liu, X. Han and L. Li, Mater. Horiz., 2020, 7, 638–666 RSC;
(c) K. J. Merazzo, A. C. Lima, M. Rincón-Iglesias, L. C. Fernandes, N. Pereira, S. Lanceros-Mendez and P. Martins, Mater. Horiz., 2021, 8, 2654–2684 RSC;
(d) M. Rafiee, R. D. Farahani and D. Therriault, Adv. Sci., 2020, 7, 1902307 CrossRef CAS PubMed.
-
(a) M. R. Jolly, J. D. Carlson and B. C. Muñoz, J. Intell. Mater. Syst. Struct., 1996, 5, 607–614 CrossRef CAS;
(b) J. D. Carlson and M. R. Jolly, Mechatronics, 2000, 10, 555–569 CrossRef.
-
(a) P. Allia, G. Barrera, P. Tiberto, T. Nardi, Y. Leterrier and M. Sangermano, J. Appl. Phys., 2014, 116, 113903 CrossRef;
(b) J. Amici, P. Allia, P. Tiberto and M. Sangermano, Macromol. Chem. Phys., 2011, 212, 1629–1635 CrossRef CAS;
(c) C. Albornoz, E. E. Sileo and S. E. Jacobo, Phys. B, 2004, 354, 149–153 CrossRef CAS;
(d) Q. Ze, X. Kuang, S. Wu, J. Wong, S. M. Montgomery, R. Zhang, J. M. Kovitz, F. Yang, H. J. Qi and R. Zhao, Adv. Mater., 2020, 32, e1906657 CrossRef PubMed;
(e) T. N. Do, H. Phan, T. -Q. Nguyen and Y. Visell, Adv. Funct. Mater., 2018, 28, 1800244 CrossRef;
(f) S. Di Zhang, Y. C. Zhai and Z. F. Zhang, AMR, 2011, 287–290, 2032–2035 Search PubMed.
-
(a) M. A. Moreno, J. Gonzalez-Rico, M. L. Lopez-Donaire, A. Arias and D. Garcia-Gonzalez, J. Magn. Magn. Mater., 2021, 224, 109148 CAS;
(b) A. K. Bastola and M. Hossain, Composites, Part B, 2020, 200, 108348 CrossRef CAS;
(c) A. Hooshiar, A. Payami, J. Dargahi and S. Najarian, Mech. Syst. Signal Process., 2021, 161, 107918 CrossRef.
-
(a) S. Xuan, Y. Xu, T. Liu and X. Gong, Int. J. Smart Nano Mater., 2015, 6, 135–148 CrossRef CAS;
(b) Y. Xu, G. Liao and T. Liu, in Nanofluid Flow in Porous Media, ed. M. Sheikholeslami Kandelousi, S. Ameen, M. Shaheer Akhtar and H.-S. Shin, IntechOpen, 2020 CrossRef.
-
(a) L. A. Makarova, Y. A. Alekhina, A. S. Omelyanchik, D. Peddis, V. V. Spiridonov, V. V. Rodionova and N. S. Perov, J. Magn. Magn. Mater., 2019, 485, 413–418 CrossRef CAS;
(b) M. D'Auria, D. Davino, R. Pantani and L. Sorrentino, Smart Mater. Struct., 2016, 25, 55014 CrossRef.
- A. Hodaei, O. Akhlaghi, N. Khani, T. Aytas, D. Sezer, B. Tatli, Y. Z. Menceloglu, B. Koc and O. Akbulut, ACS Appl. Mater. Interfaces, 2018, 10, 9873–9881 CrossRef CAS PubMed.
-
(a) A. Dey, I. N. Roan Eagle and N. Yodo, J. Manuf. Mater. Process., 2021, 5, 69 CAS;
(b) M. A. S. R. Saadi, A. Maguire, N. T. Pottackal, M. S. H. Thakur, M. M. Ikram, A. J. Hart, P. M. Ajayan and M. M. Rahman, Adv. Mater., 2022, 34, e2108855 CrossRef CAS PubMed.
- J. J. Restrepo and H. A. Colorado, J. Compos. Mater., 2020, 54, 647–657 CrossRef CAS.
- Y. Kim, H. Yuk, R. Zhao, S. A. Chester and X. Zhao, Nature, 2018, 558, 274–279 CrossRef CAS PubMed.
- A. Amirov, A. Omelyanchik, D. Murzin, V. Kolesnikova, S. Vorontsov, I. Musov, K. Musov, S. Khashirova and V. Rodionova, Processes, 2022, 10, 2412 CrossRef CAS.
-
(a) D. Kokkinis, M. Schaffner and A. R. Studart, Nat. Commun., 2015, 6, 8643 CrossRef PubMed;
(b) A. K. Bastola, M. Paudel and L. Li, Polymer, 2018, 149, 213–228 CrossRef CAS;
(c) L. Lu, P. Guo and Y. Pan, J. Manuf. Sci. Eng., 2017, 139, 071008 CrossRef.
-
(a) E. M. Palmero, J. Rial, J. de Vicente, J. Camarero, B. Skårman, H. Vidarsson, P.-O. Larsson and A. Bollero, Sci. Technol. Adv. Mater., 2018, 19, 465–473 CrossRef CAS PubMed;
(b) C. Huber, C. Abert, F. Bruckner, M. Groenefeld, S. Schuschnigg, I. Teliban, C. Vogler, G. Wautischer, R. Windl and D. Suess, Sci. Rep., 2017, 7, 9419 CrossRef PubMed.
-
(a) J. A. Cuenca, K. Bugler, S. Taylor, D. Morgan, P. Williams, J. Bauer and A. Porch, J. Condens. Matter Phys., 2016, 28, 106002 CrossRef PubMed;
(b) J. H. Kim, S. Lee, M. Wajahat, H. Jeong, W. S. Chang, H. J. Jeong, J.-R. Yang, J. T. Kim and S. K. Seol, ACS Nano, 2016, 10, 8879–8887 CrossRef CAS PubMed.
- S. Lantean, G. Barrera, C. F. Pirri, P. Tiberto, M. Sangermano, I. Roppolo and G. Rizza, Adv. Mater. Technol., 2019, 4, 1900505 CrossRef CAS.
-
(a) S. Lantean, I. Roppolo, M. Sangermano, M. Hayoun, H. Dammak and G. Rizza, Addit. Manuf., 2021, 47, 102343 CAS;
(b) S. Lantean, I. Roppolo, M. Sangermano, M. Hayoun, H. Dammak, G. Barrera, P. Tiberto, C. F. Pirri, L. Bodelot and G. Rizza, Adv. Mater. Technol., 2022, 7, 2200288 CrossRef CAS.
- E. Rossegger, R. Höller, K. Hrbinič, M. Sangermano, T. Griesser and S. Schlögl, Adv. Eng. Mater., 2022, 2200749 Search PubMed.
- E. Rossegger, R. Höller, D. Reisinger, J. Strasser, M. Fleisch, T. Griesser and S. Schlögl, Polym. Chem., 2021, 12, 639–644 RSC.
- C. Hinczewski, S. Corbel and T. Chartier, Rapid Prototyp. J., 1998, 4, 104–111 CrossRef.
- J.-H. Lee, C.-M. Um and I.-b. Lee, Dent. Mater., 2006, 22, 515–526 CrossRef CAS PubMed.
- A. Huber, A. Kuschel, T. Ott, G. Santiso-Quinones, D. Stein, J. Bräuer, R. Kissner, F. Krumeich, H. Schönberg, J. Levalois-Grützmacher and H. Grützmacher, Angew. Chem., Int. Ed., 2012, 51, 4648–4652 CrossRef CAS PubMed.
-
(a) M. Sangermano, W. Carbonaro, G. Malucelli and A. Priola, Macromol. Mater. Eng., 2008, 293, 515–520 CrossRef CAS;
(b) E. Rossegger, D. Nees, S. Turisser, S. Radl, T. Griesser and S. Schlögl, Polym. Chem., 2020, 11, 3125–3135 RSC.
-
(a) N. B. Cramer and C. N. Bowman, J. Polym. Sci., Part A: Polym. Chem., 2001, 39, 3311–3319 CrossRef CAS;
(b) M. Sahin, S. Ayalur-Karunakaran, J. Manhart, M. Wolfahrt, W. Kern and S. Schlögl, Adv. Eng. Mater., 2017, 19, 1600620 CrossRef.
Footnote |
† Electronic supplementary information (ESI) available: FTIR spectra and photographs of printed test structures after testing. See DOI: https://doi.org/10.1039/d3ra02504g |
|
This journal is © The Royal Society of Chemistry 2023 |
Click here to see how this site uses Cookies. View our privacy policy here.