DOI:
10.1039/D3RA03055E
(Paper)
RSC Adv., 2023,
13, 19140-19148
g-C3N4-modified Zr-Fc MOFs as a novel photocatalysis-self-Fenton system toward the direct hydroxylation of benzene to phenol†
Received
8th May 2023
, Accepted 12th June 2023
First published on 23rd June 2023
Abstract
In order to explore a green, economic, and sustainable phenol production process, a heterojunction semiconductor materials g-C3N4/Zr-Fc MOF was synthesized via an in situ synthesis method. With the synergistic effect of photocatalysis and the Fenton effect, the composite could effectively catalyze the direct hydroxylation of benzene to phenol under visible light irradiation. The yield of phenol and the selectivity were 13.84% and 99.38% under the optimal conditions, respectively, and it could still maintain high photocatalytic activity after 5 photocatalytic cycles. Therefore, the designed photocatalysis-self-Fenton system has great potential in the field of the direct hydroxylation of benzene to phenol.
Introduction
Phenol is an important chemical intermediate in the industrial field. At present, it is mainly produced by the three-step isopropyl benzene process, which has serious limitations, such as dangerous intermediates and high energy consumption.1 Therefore, a green, economic, and sustainable phenol production process is urgently needed. In recent years, the direct hydroxylation of benzene has attracted increasing attention. This is a mild, economically green solution.2 Gu et al. achieved promising results with a phenol regimen using a light-induced benzene efficient hydroxylation, with high selectivity of up to 99% and good phenol yields.3 Owing to its cost-effectiveness and environmental friendliness, photocatalysis could be applied as a competitive method to achieve benzene hydroxylation into phenols.
Recently, with the strong oxidation of hydroxyl radicals (·OH) the photocatalysis-self-Fenton process has been used by researchers to convert benzene into phenol.4 The process of photo-Fenton mainly involves the transformation between Fe(III) and Fe(II).5 Various iron-based MOFs have been used as photocatalyst for the direct hydroxylation of benzene to phenol. Xu proposed MIL-100(Fe) nanoparticles, which were synthesized by ethylene glycol with a uniform size and morphology to perform the photocatalytic direct hydroxylation of benzene experiments, and the result showed that the MIL-100(Fe) nanospheres possessed a good oxidation ability and high selectivity.6 The experiments into phenol production with iron-based MOFs reported by Wang et al. also confirmed the feasibility of benzene–phenol conversion using the Fenton effect of the Fe-based MOFs, whereby the high catalytic activity of iron-containing materials in the selective oxidation process enables the wide application of Fe-based MOFs for the direct hydroxylation of benzene to produce phenol.7 Owing to the tunable composition and structure of the iron-based MOF materials, they have great potential for the photocatalytic hydroxylation of benzene to produce phenol.8,9 To obtain better photocatalytic phenol performance, the iron-based MOF structure can be adjusted to extend the photoresponse range. Constructing heterojunctions is a promising strategy to improve the performance of the heterogeneous photo-Fenton reaction (PFR).10 As a photoresponse semiconductor, g-C3N4 has been often used to enhance the optical response range owing to its advantages of strong acid and alkali resistance, appropriate optical band range (2.76 eV), high thermal stability, and non-toxicity.11–13 Zehra Durmus et al. built a unique hybrid structure between Ce-MOFs and g-C3N4 to improve the photocatalytic performance.14 Devarayapalli et al. prepared g-C3N4/ZIF-67 heterojunction composites by a coupling cobalt–organic zeolite imidazole skeleton (ZIF-67) with g-C3N4 nanosheets synthesized by a simple microwave radiation method, which greatly improved the photocatalytic performance of the carbon nitride graphite.15 Reza Abazari synthesized Ti-MIL-125-NH2 modified with g-C3N4 by a simple-rapid ultrasonic method, which was then used as a visible-light photocatalyst with an improved photocatalytic performance.16 All these experimental protocols demonstrated that the combination of g-C3N4 and iron-based MOFs would enhance the photocatalytic performance.
In this investigation, we report an efficient photocatalysis-self-Fenton system, namely g-C3N4/Zr-Fc MOF, constructed by an in situ composite method. Thereinto, zirconium(IV), 1,1′-ferrocenedicarboxylic acid, and g-C3N4 were selected as the central metal ion, organic ligand, and support system, respectively. Due to the synergistic effect of the mixed metals in MOFs, Zr-Fc MOFs can provide more abundant electrons and holes.17–19 Besides, the heterojunction possesses a large specific surface area and stable structure. The hydroxyl radicals produced by the Fenton reaction between Fe(II) and hydrogen peroxide could have a synergistic effect with the presence of a bimetal to greatly improve the direct hydroxylation of benzene. At the same time, the band gap width of g-C3N4 is about 2.7 eV, which can effectively absorb sunlight and also it has a stable structure under light conditions.20 Therefore, the designed photocatalyst was expected to possess a better effect in the direct hydroxylation of benzene to phenol under visible light.
Experimental
Materials and chemicals
1,1′-Ferrocenedicarboxylic acid (98%) was purchased from Sun Chemical Technology (Shanghai) Co., Ltd. Zirconium tetrachloride (ZrCl4, 98%) was purchased from Guangdong Hachuring Chemical Reagent Co., Ltd. Melamine (99.5%) was purchased from Tianjin Guangfu Fine Chemical Research Institute. Acetic acid (AC) was purchased from Tianjin Tianli Chemical Reagent Co. Ltd. N-Dimethylformamide (AC) was purchased from Tianjin Fuyu Fine Chemical Co. Ltd. Benzene was purchased from Yantai Shuangshuang Chemical Co. Ltd. Ethanol (AC) was purchased from Tianjin Kermel Chemical Reagent Co., Ltd. Trifluoroacetic acid was purchased from Shanghai Macklin Biochemical Co., Ltd.
Synthesis of g-C3N4. An alumina crucible containing 10 g melamine was placed into a tubular furnace and heated to 600 °C at a heating rate of 2.3 °C min−1 under an air atmosphere and stabilized for 2 h. The yellow product in the crucible was carefully collected and ground into a powder. The prepared g-C3N4 powder was placed into an alumina crucible and annealed in an air stream at 550 °C at the rate of 2.3 °C min−1 for 3 h. The yellow-product layered g-C3N4 in the crucible was carefully collected.21
Synthesis of Zr-Fc MOFs. ZrCl4 (700 mg, 3 mmol) and 1,1′-ferrocenedicarboxylic acid (823.2 mg, 3 mmol) were added in to 90 mL N,N′-dimethylformamide, and dispersed for 15 min in an ultrasonic cell dispersion apparatus. Then, glacial acetic acid (8.6 mL) was pipetted in to the mixture and stirred for about 15 min until the solution was uniform. The mixture was transferred into a 200 mL Teflon reactor and reacted at 120 °C for 12 h. Then, the product was collected by centrifugation, and washed with DMF and ethanol three times. The product was dried in a vacuum oven.22
Preparation and synthesis of g-C3N4/Zr-Fc MOFs. ZrCl4 (700 mg, 3 mmol), 1,1′-ferrocenedicarboxylic acid (823.2 mg, 3 mmol), and g-C3N4 (0.2 g) were added in to 90 mL N,N′-dimethylformamide, and dispersed for 15 min in an ultrasonic cell dispersion apparatus. Then, glacial acetic acid (8.6 mL) was pipetted in to the mixture and stirred for about 15 min until the solution was uniform. The mixture was transferred into a 200 mL Teflon-lined reactor and reacted at 120 °C for 12 h. Then, the product was collected by centrifugation, and washed with DMF and ethanol three times. The product was dried in a vacuum oven.22
Characterization of the composite. The surface morphology and particle size of the samples were measured by scanning electron microscopy (SEM). The operating voltage was 10 k V. The silicon wafer containing the sample was fixed on the sample table with conductive tape, and then gold sputtering was applied. Finally, the sample was characterized by SEM. X-Ray diffraction spectroscopy was performed using an Ultima IV type X-ray diffraction instrument as the characterization instrument, with a nickel-filter Cu Kα target used as the incident light source, λ = 0.15418 nm as the incident wavelength, 5–80° as the scanning range, 40 kV as the scanning voltage, and 10° min−1 as the scanning speed. The crystal structure of the sample was obtained by X-ray diffraction spectrum analysis. The Fourier-transform infrared (FT-IR) information was investigated using a Nicolet iS50 system with measurements made at 4000–400 cm−1 as the detection range. The solid UV diffuse reflectance spectra were obtained through an integral sphere system equipped with a UV-2600 UV-visible spectrophotometer (Shimadzu, Japan). Barium sulfate powder was used as the reference to measure the baseline and then the DRS spectrum of the sample was determined.
Photocatalytic benzene hydroxylation experiments. The photocatalytic benzene direct hydroxylation experiments were carried out using the PCX-50C multi-channel photochemical reaction system (Beijing Perfectlight Technology Co., Ltd) as well as under visible-light irradiation. An LED lamp (5 W), which provided simulated sunlight full-spectrum irradiation (380–760 nm), was used as the light source. Benzene (1.6 mL), trifluoroacetic acid (0.1 g), and acetonitrile (11 mL) were added into the photoreactor. Then, the catalyst (20 mg) was added into the mixture and mixed evenly under magnetic stirring. The photocatalytic experiments were carried out at 60 °C, and hydrogen peroxide (0.6 mL) was added to the reactor within 30 min. The reaction was maintained for 4 h. After the reaction, the conversion of benzene and the yield of phenol were determined by gas chromatography (GC, Techcomp GC7900), for which the injection port temperature was 210 °C, FID was selected as the detector, and the temperature was set at 240 °C. To investigate the optimal reaction conditions for the experiments, the reaction conditions, such as the ratio of g-C3N4 and benzene, reaction time, and reaction temperature, were varied to explore the photocatalytic properties.The conversion rate of benzene, selectivity of phenol, and yield of phenol were calculated using the following three formulas:
Results and discussion
Sample structure characterization
The morphologies of g-C3N4, Zr-Fc MOF, and g-C3N4/Zr-Fc MOF were observed by SEM. As shown in Fig. 1, the prepared g-C3N4 with a large specific surface area possessed a sheet stacked structure with an irregular appearance and different sizes.23 The Zr-Fc MOF possessed irregular sheet-like structures without agglomerates, which were consistent with previously reported SEM images.24 The g-C3N4/Zr-Fc MOF was combined based on the g-C3N4 and Zr-Fc MOF. The Zr-Fc MOF particles were evenly dispersed over the g-C3N4, basically maintaining the original morphologies of g-C3N4 and Zr-Fc MOF, which indicated the successful composition of the g-C3N4/Zr-Fc MOF material (Fig. 1c).
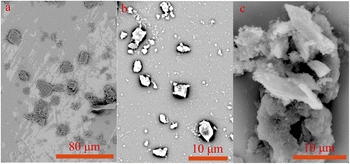 |
| Fig. 1 SEM spectra of g-C3N4 (a), Zr-Fc MOF, (b) and g-C3N4/Zr-Fc MOF (c). | |
The crystal structure of the sample as well as the crystallinity were investigated by X-ray diffraction, and the XRD profiles of g-C3N4, Zr-Fc MOF, and g-C3N4/Zr-Fc MOF are displayed in Fig. 2a. A strong diffraction peak occurring at 27.96° was the characteristic diffraction peak of the (002) crystal surface and corresponded to g-C3N4.25 The characteristic diffraction peaks of the Zr-Fc MOF were consistent with the previously reported literature. The diffraction peaks at 2θ = 6.35°, 8.93°, 14.01°, 19.94°, and 25.82° could be observed from the XRD pattern of Zr-Fc MOF, corresponding to the (100), (110), (210), (310), and (320) crystal faces of Zr-Fc MOF, respectively.26 The diffraction peaks of the g-C3N4/Zr-Fc MOF composites were consistent with the characteristic diffraction peaks of both g-C3N4 and Zr-Fc MOF, indicating that the g-C3N4/Zr-Fc MOF composites had been successfully prepared.
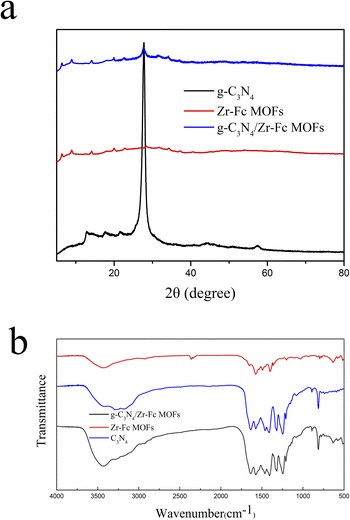 |
| Fig. 2 XRD patterns (a) and infrared spectra (b) of g-C3N4, Zr-Fc MOF, and g-C3N4/Zr-Fc MOF. | |
The structural information, such as chemical bonds and functional groups of g-C3N4, Zr-Fc MOF, and g-C3N4/Zr-Fc MOF, were acquired using the Fourier-transform infrared technique (Fig. 2b). For g-C3N4, the absorption peak at 813 cm−1 was attributed to the bending vibration peak of the triazine cycle in the g-C3N4 structure.27 The absorption peak at 487 cm−1 was assigned to the asymmetric expansion vibration peak of Zr-(OC),while the absorption peaks that appeared at 794, 1027, 1107, and 1492 cm−1 were attributed to the out-of-plane deformation vibration of C–H, C–H deformation vibration, C–C deformation vibration, and C
C stretching vibration on the cyclopentadienyl ring.28,29 This set of data suggested the formation of Zr-Fc MOF structures. The characteristic absorption peaks of ferrocene, Zr-(OC), and triazine cycle all appeared in the spectra of the composites, which indicated the successful preparation of the g-C3N4/Zr-Fc MOF.
To explore the photoresponse range of the sample and calculate the band gap width of the catalyst, characterization analysis was performed by solid ultraviolet-visible diffuse reflectance spectroscopy. As shown in Fig. 3a, the photoresponse range of Zr-Fc MOF was 250–400 nm, with two absorption peaks noted at 260 and 380 nm, respectively. However, when the Zr-Fc MOF was modified with g-C3N4, the photoresponse range of the Zr-Fc MOF was 250–400 nm with a red-shift. The band gap of semiconductors can be calculated according to the equation (αhν)1/n = A(hν − Eg) (Fig. 3b). The band gap widths of g-C3N4, Zr-Fc MOF and g-C3N4/Zr-Fc MOF were 2.40, 2.13, and 2.03 eV, respectively. The band gap width of these semiconductors was gradually reduced, which indicated that the photocatalytic performance was gradually enhanced under visible light. Hence, the g-C3N4/Zr-Fc MOF might be a desired photocatalyst for benzene hydroxylation to produce phenol.
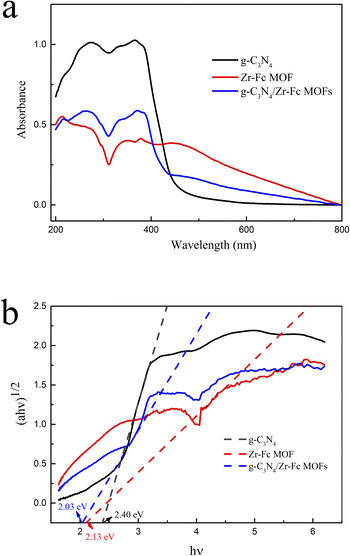 |
| Fig. 3 Solid UV diffuse reflection (a) and band gap diagram (c) of g-C3N4, Zr-Fc MOF, and g-C3N4/Zr-Fc MOF. | |
The photogenerated hole–charge separation effect is a key factor in the photocatalytic organic oxidation reaction, whereby the higher the separation efficiency, the stronger the photocatalytic activity. Therefore, the photoluminescence performances of g-C3N4 and g-C3N4/Zr-Fc MOF were investigated at the emission wavelength of 375 nm (Fig. 4a). Compared to g-C3N4, the composite g-C3N4/Zr-Fc MOF displayed a lower fluorescence intensity at the wavelength of 440 nm. This phenomenon suggested that the g-C3N4/Zr-Fc MOF possessed a higher photogenerated hole–charge separation efficiency.
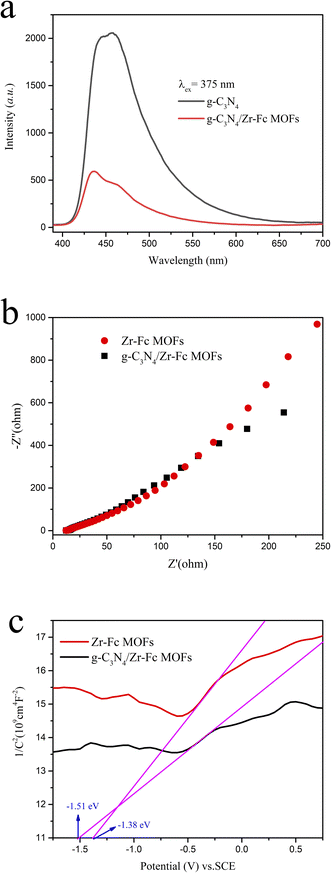 |
| Fig. 4 PL profiles (a) of g-C3N4 and g-C3N4/Zr-Fc MOF. Electrochemical impedance profiles (b) and Mott–Schottky diagram (c) of Zr-Fc MOF and g-C3N4/Zr-Fc MOF. | |
The effect of photogenerated charge–hole recombination could be further observed by electrochemical impedance spectroscopy. The EIS Nyquist curves of Zr-Fc MOF and g-C3N4/Zr-Fc MOF electrodes were detected under light avoidance conditions (Fig. 4b). When g-C3N4 was introduced to Zr-Fc MOF, the g-C3N4/Zr-Fc MOF showed a smaller radius of the Nyquist circle and smaller transfer resistance, which implied the composite possessed a lower recombination rate of photogenerated carriers. By fitting calculations, the charge-transfer resistance (Rct) and solution resistance (Rs) of the g-C3N4/Zr-Fc MOF were calculated to be 6.97 and 9.39 Ω, respectively. The charge-transfer resistance (Rct) and solution resistance (Rs) of Zr-Fc MOF were 38.33 and 96.16 Ω, respectively. Hence, the g-C3N4/Zr-Fc MOF displayed a higher photocatalytic performance.
The information of the semiconductor type and flat band potential could be obtained via Mott–Schottky curves. In order to investigate the effect of g-C3N4 introduction to Zr-Fc MOF on the band structure, Mott–Schottky curves of Zr-Fc MOF and g-C3N4/Zr-Fc MOF semiconductors were obtained by the Mott–Schottky measurement method (Fig. 4c). The two curves revealed that the tangent line presented a positive slope at the frequency of 1000 Hz, which indicated that the Zr-Fc MOF and g-C3N4/Zr-Fc MOF were both typical N-type semiconductors. Further analysis showed that the flat band potential of the Zr-Fc MOF and g-C3N4/Zr-Fc MOF were about −1.38 and −1.51 V, respectively. Previous studies have reported that the conduction band (CB) potential of N-type semiconductors is negative 0.1 V compared with its flat band potential.30 It could be found that g-C3N4/Zr-Fc MOF had a more negative conduction band potential than Zr-Fc MOF, which demonstrated that the incorporation of g-C3N4 was valuable for the improvement of the photogenerated charge–hole separation efficiency. In conclusion, as the composite rate of the photocharged holes could be effectively suppressed, the photocarrier lifetime could be extended, and the photoelectrons could be effectively transferred to produce active free radicals, which could be applied for the photocatalytic benzene hydroxylation reaction.
Photocatalytic experiments
Effect of different catalysts. The enhancement of the photocatalytic performance was estimated by comparing the ability of the three photocatalytic materials for the direct hydroxylation of benzene under visible light. As shown in Table 1, a lower phenol yield could be detected in the post-reaction products catalyzed by g-C3N4 and Zr-Fc MOF, whereby the phenol yields were 4.78% and 8.80%, respectively. When the composite was prepared by the combination of g-C3N4 and Zr-Fc MOF, the yield was increased to 13.84%, and the selectivity was 99.38% when using the g-C3N4/Zr-Fc MOF as the catalyst under the same conditions. This might be due to the g-C3N4 having a photocatalytic ability, while the Zr-Fc MOF could activate hydrogen peroxide to generate free radicals (˙OH) via a Fenton reaction, and then the synergistic effect of photocatalysis and the Fenton reaction improved the direct hydroxylation of benzene to phenol. Therefore, in subsequent research experiments, the g-C3N4/Zr-Fc MOF was selected as the photocatalyst for benzene hydroxylation to phenol.
Table 1 Effect of different catalysts on catalytic benzene hydroxylationa
Sample |
Yield (%) |
Conversion (%) |
Selectivity (%) |
Reaction conditions: catalyst (1.5 mg mL−1), benzene (1.6 mL, 18.05 mmol), H2O2 (30 wt%) (0.6 mL), acetonitrile (11 mL), trifluoroacetic acid (0.1 g), time: 4 h, and T: 333 K. |
g-C3N4 |
4.78 |
4.92 |
97.15 |
Zr-Fc MOF |
8.80 |
8.95 |
98.32 |
g-C3N4/Zr-Fc MOF |
13.84 |
13.93 |
99.38 |
Effect of the catalyst dosage. In order to explore the effect of the quality of the as-prepared g-C3N4/Zr-Fc MOF on the benzene hydroxylation to phenol performance, catalyst samples of different amounts were investigated under the photocatalytic process. As displayed in Table 2, when there was no photocatalyst, it was hard for benzene to directly undergo hydroxylation under light irradiation, and only a little phenol was produced. With the increase in the photocatalyst amount, the performance of the benzene hydroxylation reaction was greatly improved, mainly because more catalysts could meet the quantity of Fe2+ demanded for the Fenton reaction, and more ·OH was generated to react with benzene to produce phenol.31,32 When the catalyst quality was further increased, the yield of phenol decreased instead. This might be due to the increased catalyst powder in the photocatalytic process, which might cause a shielding effect of light while the excess hydroxyl radicals could not participate in the reaction in time due to their short life. Therefore, considering all the above, the optimal catalyst mass was 20 mg.
Table 2 Effect of the amount of catalyst on catalytic benzene hydroxylationa
Entry |
m (mg mL−1) |
Yield (%) |
Conversion (%) |
Selectivity (%) |
Reaction conditions: benzene (1.6 mL, 18.05 mmol), H2O2 (30 wt%) (0.6 mL), acetonitrile (11 mL), trifluoroacetic acid (0.1 g), time: 4 h, and T: 333 K. |
1 |
0 |
4.87 |
5.03 |
96.81 |
2 |
0.75 |
7.69 |
7.96 |
96.61 |
3 |
1.50 |
13.84 |
13.93 |
99.38 |
4 |
2.25 |
8.48 |
8.85 |
95.82 |
Effect of the amount of H2O2. The amount of H2O2 is another vital factor in the direct hydroxylation of benzene under the photocatalysis. Hence, the amount of H2O2 was investigated to explore the appropriate conditions for the reaction. As illustrated in Table 3, the photocatalyst could obtain a better benzene hydroxylation to phenol performance, whereby the phenol yield and selectivity were 13.84% and 99.38%, respectively, under the volume ratio of n(H2O2)/n(benzene) = 8
:
3. However, when the amount of H2O2 was increased, the yield of phenol, the conversion rate of benzene, and the selectivity of phenol gradually decreased. This might be due to the H2O2 undergoing a self-decomposition during the reaction, and the self-decomposition rate was faster than that of benzene hydroxylation, which would cause the reaction solution to gradually become two phases, thus hindering the interaction between benzene and the catalyst.33 Furthermore, the effect of light on benzene hydroxylation to produce phenol was also explored under the same reaction conditions with the volume ratio of n(H2O2)/n(benzene) = 8
:
3. When there was no visible-light irradiation, the yield of phenol was 8.17%, which implied that only the Fenton reaction occurred under dark conditions. Hence, the Fenton reaction and photocatalysis cooperate to improve the benzene hydroxylation to phenol performance.
Table 3 Effect of the added amount of H2O2 on catalytic benzene hydroxylationa
Entry |
n(H2O2)/n(benzene) |
Yield (%) |
Conversion (%) |
Selectivity (%) |
Reaction conditions: catalyst (1.5 mg mL−1), benzene (1.6 mL, 18.05 mmol), acetonitrile (11 mL), trifluoroacetic acid (0.1 g), time: 4 h, and T: 333 K. No visible-light exposure. |
1 |
2/8 |
7.39 |
7.57 |
97.62 |
2 |
3/8 |
13.84 |
13.93 |
99.38 |
3 |
4/8 |
9.85 |
9.98 |
98.70 |
4 |
5/8 |
8.66 |
8.80 |
98.40 |
5b |
3/8 |
8.17 |
8.28 |
98.67 |
Effect of trifluoroacetic acid dosage. As a cocatalyst, trifluoroacetic acid plays a key role in the direct hydroxylation of benzene, whereby it can enhance the activity of the ferrous ions in g-C3N4/Zr-Fc MOF.34 As displayed in Table 4, when the cocatalyst was not added into the photocatalytic process, the yield of phenol was only 6.27%. With the increase in trifluoroacetic acid, the yield of phenol gradually increased. When the addition of trifluoroacetic acid reached 0.1 g, the result of the reaction was optimal, and the phenol yield, benzene conversion rate, and selectivity were 13.84%, 13.93%, and 99.38%. However, when the amount of trifluoroacetic acid was further increased, the photocatalytic performance declined. This might be due to the presence of trifluoroacetic acid inhibiting the occurrence of peroxidation reactions, so the more trifluoroacetic acid, the stronger the inhibition, and the smaller the phenol yield. The effects of other acidic additives were studied when used in place of trifluoroacetic acid, including acetic acid, formic acid, hydrochloric acid, and phosphoric acid. The results showed that trifluoroacetic acid had the best co-catalytic effect among these acids (Fig. S6†).
Table 4 Effect of the amount of trifluoroacetic acid on catalytic benzene hydroxylationa
Entry |
Mass of TFA (g) |
Yield (%) |
Conversion (%) |
Selectivity (%) |
Reaction conditions: catalyst (1.5 mg mL−1), benzene (1.6 mL, 18.05 mmol), H2O2 (30 wt%) (0.6 mL), acetonitrile (11 mL), time: 4 h, and T: 333 K. |
1 |
0 |
6.27 |
6.52 |
96.16 |
2 |
0.05 |
7.45 |
7.79 |
95.64 |
3 |
0.10 |
13.84 |
13.93 |
99.38 |
4 |
0.15 |
10.86 |
11.23 |
96.71 |
5 |
0.20 |
7.96 |
8.03 |
99.13 |
Effect of the reaction time and temperature. The effect of different reaction times on the selective catalytic oxidation of benzene by g-C3N4/Zr-Fc MOF is illustrated in Table 5. The reaction time plays a vital role in the direct hydroxylation of benzene to phenol, whereby initially the yield increased with the increase in reaction time, and then the yield decreased as the time was further increased. The yield of phenol reached to 13.84%, when the reaction time was 4 h. Later, the photocatalytic activity decreased slightly with the extension of reaction time, which might be due to some peroxidation reactions of phenol and hydroxyl groups occurring to produce benzoquinone byproducts with the increase in reaction time.
Table 5 Effect of the reaction time on catalytic benzene hydroxylationa
Entry |
t (h) |
Yield (%) |
Conversion (%) |
Selectivity (%) |
Reaction conditions: catalyst (1.5 mg mL−1), benzene (1.6 mL, 18.05 mmol), H2O2 (30 wt%) (0.6 mL), acetonitrile (11 mL), trifluoroacetic acid (0.1 g), and T: 333 K. |
1 |
3 |
9.53 |
10.06 |
94.73 |
2 |
4 |
13.84 |
13.93 |
99.38 |
3 |
5 |
10.61 |
11.37 |
93.31 |
The effect of different reaction temperatures on the selective catalytic oxidation of benzene by g-C3N4/Zr-Fc MOF is illustrated in Table 6. Initially the yield increased with the increase in reaction time, and then the yield decreased as the time was further increased. The ideal benzene conversion and phenol selectivity were obtained at 60 °C. The reason for this might be because the higher reaction temperature was conducive to the generation of active free radicals required in the photocatalytic process, which accelerated the photocatalytic reaction and increased the benzene conversion and yield of phenol to 13.84% and 13.93%, respectively. When the reaction temperature was raised close to the boiling point of benzene, the volatilization of the reactants was easier.35 Also, the self-decomposition of hydrogen peroxide and the deep oxidation of phenol might be caused by high temperature. Therefore, the optimum time for benzene hydroxylation was 4 h and the optimum temperature was 60 °C.
Table 6 Effects of the reaction temperature on catalytic benzene hydroxylationa
Entry |
T (°C) |
Yield (%) |
Conversion (%) |
Selectivity (%) |
Reaction conditions: catalyst (1.5 mg mL−1), benzene (1.6 mL, 18.05 mmol), H2O2 (30 wt%) (0.6 mL), acetonitrile (11 mL), trifluoroacetic acid (0.1 g), and time: 4 h. |
1 |
50 |
9.30 |
9.64 |
96.47 |
2 |
60 |
13.84 |
13.93 |
99.38 |
3 |
70 |
5.22 |
5.39 |
96.85 |
For the catalyst, its regeneration and reusability are also an important factor to be investigated. Thus here, after each photocatalytic reaction, the composite was collected by centrifugation and washed with ethanol, as shown in Fig. 5, the photocatalytic performance of g-C3N4/Zr-Fc MOF could be well maintained after five cycles, and the yield of phenol and the selectivity were 12.49% and 94.88%, respectively. This suggested that the semiconductor photocatalyst was relatively stable and could achieve the goal of being recycled and reused.
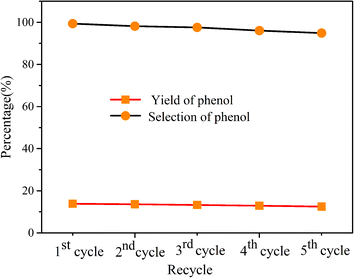 |
| Fig. 5 Regeneration and reusability of g-C3N4/Zr-Fc MOF. Reaction conditions: catalyst (1.5 mg mL−1), benzene (1.6 mL, 18.05 mmol), H2O2 (30 wt%) (0.6 mL), acetonitrile (11 mL), trifluoroacetic acid (0.1 g), time: 4 h, and T = 333 K. | |
A comparative study of g-C3N4/Zr-Fc MOF and other photocatalysts previously reported for the photocatalytic hydroxylation of benzene to phenol was conducted, and the results are shown in Table 7. The phenol selectivity (99%) and yield (13.84%) obtained using the g-C3N4/Zr-Fc MOF photocatalyst were higher than or comparable with those obtained using other photocatalysts.
Table 7 Comparison of the photocatalytic activity of g-C3N4/Zr-Fc MOF with other reported photocatalysts for the photocatalytic hydroxylation of benzene to phenol
Sample |
Amount |
Time (h) |
Yield (%) |
Ref. |
Fe–Cu/beta |
5 mg mL−1 |
6 |
10.5 |
36 |
Fe–g-C3N4/SBA-15 |
5.5 mg mL−1 |
4 |
11.9 |
37 |
Fe–g-C3N4 |
5.5 mg mL−1 |
4 |
8.3 |
38 |
UiO-66-NH2-SA-V |
1.5 mg mL−1 |
4 |
15.3 |
39 |
5% Cr–CdS/ZnO |
5 mg mL−1 |
4 |
11.1 |
40 |
g-C3N4/Zr-Fc MOFs |
1.5 mg mL−1 |
4 |
13.84 |
This work |
The h+, e−, and ·OH were the main active substances involved in the photocatalytic reaction.41 In order to illuminate the mechanism of g-C3N4/Zr-Fc MOF in the process of the direct hydroxylation of benzene to phenol under photocatalysis, the active substances that played a vital role in the reaction were determined by free-radical-capture experiments under the optimal conditions. Thereinto, isopropanol (IPA), potassium dichromate (K2Cr2O7), and disodium ethylenediaminetetraacetate (EDTA-2Na) were selected as scavengers of hydroxyl radicals (·OH), electrons (e−), and holes (h+), respectively. As shown in Fig. 6, when the capture agents were added into the reaction mixture, the yield of phenol decreased to some extent in all cases. Thereinto, the yield of phenol was greatly inhibited with the addition of IPA. This phenomenon implied that the direct hydroxylation of benzene to phenol was primarily driven by the participation of hydroxyl radicals.
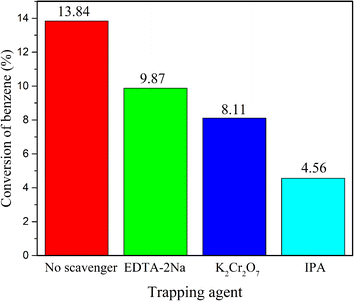 |
| Fig. 6 Free-radical-trapping experiments. Reaction conditions: catalyst (1.5 mg mL−1), benzene (1.6 mL, 18.05 mmol), H2O2 (30 wt%) (0.6 mL), solvent (acetonitrile: 11 mL), trifluoroacetic acid (0.1 g), t = 4 h, and T = 333 K. | |
Basic reaction mechanism
According to the aforementioned free-radical-trapping experiment results, a possible photocatalytic mechanism of benzene hydroxylation to phenol is proposed in Fig. 7. In the visible-irradiated Fenton system, electrons and holes are mainly generated from the semiconductor g-C3N4/Zr-Fc MOF under visible light, and hydroxyl radicals (·OH) are primarily produced by the Fenton reaction, in which hydrogen peroxide is reduced by Fe2+ in the photocatalyst. Furthermore, ·OH also could be generated by the interaction between hydrogen peroxide, electrons, and holes. First, the H2O2 reacts with electrons to produce OH−, and then, the generated OH− could be oxidized by h+ to give ·OH. All the obtained ·OH could then oxidize benzene molecules to phenol.42,43
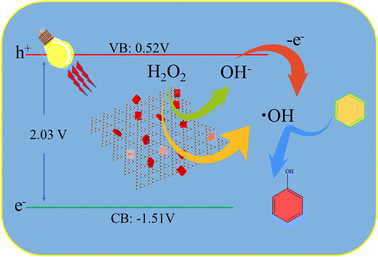 |
| Fig. 7 Schematic diagram of the proposed photocatalytic mechanism. | |
Conclusions
In summary, a novel photocatalytic semiconductor material g-C3N4/Zr-Fc MOFs was successfully prepared via an in situ synthesis method. Compared with g-C3N4 and Zr-Fc MOF, the photocatalytic performance of the composite was significantly improved, and it could effectively catalyze the direct hydroxylation of benzene to phenol under visible-light irradiation. The yield of phenol and selectivity were 13.84% and 99.38% under the optimal conditions, respectively. The performance and structure of the catalyst were relatively stable, and it could still maintain high photocatalytic activity after 5 photocatalytic cycles. Based on these excellent properties, the g-C3N4/Zr-Fc MOF can be expected to be widely used in the field of photocatalytic benzene direct hydroxylation to phenol.
Conflicts of interest
There are no conflicts to declare.
Acknowledgements
This project was financially supported by 2018 Funding Plan for Young Core Teachers of Zhongyuan University of Technology, Discipline Strength Improvement Program of Zhongyuan University of Technology: Program for Cultivating Disciplinary Young Master Supervisor (SD202204), the Basic Scientific Research Program of Zhongyuan University of Technology (K2018QN018).
Notes and references
- W. Han, W. Xiang and J. Shi, et al., Recent advances in the heterogeneous photocatalytic hydroxylation of benzene to phenol, Molecules, 2022, 27(17), 5457 CrossRef CAS PubMed.
- S. M. Hosseini, M. Ghiaci and S. A. Kulinich, et al., Au-Pd@g-C3N4 as an efficient photocatalyst for visible-light oxidation of benzene to phenol: experimental and mechanistic study, J. Phys. Chem. C, 2018, 122(48), 27477–27485 CrossRef CAS.
- Y. Gu, Q. Li and D. Zang, et al., Light-induced efficient hydroxylation of benzene to phenol by quinolinium and polyoxovanadate-based supramolecular catalysts, Angew. Chem., Int. Ed., 2021, 60(24), 13310–13316 CrossRef CAS PubMed.
- R. Liu, Y. Xu and B. Chen, Self-assembled nano-FeO(OH)/reduced graphene oxide aerogel as a reusable catalyst for photo-Fenton degradation of phenolic organics, Environ. Sci. Technol., 2018, 52(12), 7043–7053 CrossRef CAS PubMed.
- W. Q. Li, Y. X. Wang and J. Q. Chen, et al., Boosting photo-Fenton process enabled by ligand-to-cluster charge transfer excitations in iron-based metal organic framework, Appl. Catal., B, 2022, 302, 120882 CrossRef CAS.
- B. Xu, Z. Chen and B. Han, et al., Glycol assisted synthesis of MIL-100(Fe) nanospheres for photocatalytic oxidation of benzene to phenol, Catal. Commun., 2017, 98, 112–115 CrossRef CAS.
- D. Wang, M. Wang and Z. Li, Fe-based metal–organic frameworks for highly selective photocatalytic benzene hydroxylation to phenol, ACS Catal., 2015, 5(11), 6852–6857 CrossRef CAS.
- B. L. Xiang, L. Fu and Y. Li, et al., Preparation of Fe(II)/MOF-5 catalyst for highly selective catalytic hydroxylation of phenol by equivalent loading at room temperature, J. Chem., 2019, 2019, 1–10 Search PubMed.
- Q. Zhao, L. Zhang and M. Zhao, et al., Vanadium oxyacetylacetonate grated on metal organic framework as catalyst for the direct hydroxylation of benzene to phenol, ChemistrySelect, 2020, 5(22), 6818–6822 CrossRef CAS.
- D. Wang and Z. Li, Iron-based metal–organic frameworks (MOFs) for visible-light-induced photocatalysis, Res. Chem. Intermed., 2017, 43, 5169–5186 CrossRef CAS.
- X. Wang, S. Blechert and M. Antonietti, Polymeric graphitic carbon nitride for heterogeneous photocatalysis, ACS Catal., 2012, 2(8), 1596–1606 CrossRef CAS.
- S. Cao, J. Low and J. Yu, et al., Polymeric photocatalysts based on graphitic carbon nitride, Adv. Mater., 2015, 27(13), 2150–2176 CrossRef CAS PubMed.
- J. Liu, H. Wang and M. Antonietti, Graphitic carbon nitride “reloaded”: emerging applications beyond (photo) catalysis, Chem. Soc. Rev., 2016, 45(8), 2308–2326 RSC.
- Z. Durmus, R. Köferstein and T. Lindenberg, et al., Preparation and characterization of Ce-MOF/g-C3N4 composites and evaluation of their photocatalytic performance, Ceram. Int., 2023, 49, 24428–24441 CrossRef.
- K. C. Devarayapalli, S. V. P. Vattikuti and T. V. M. Sreekanth, et al., Hydrogen production and photocatalytic activity of g-C3N4/Co-MOF (ZIF-67) nanocomposite under visible light irradiation, Appl. Organomet. Chem., 2020, 34(3), e5376 CrossRef CAS.
- R. Abazari, A. R. Mahjoub and G. Salehi, Preparation of amine functionalized g-C3N4@ H/SMOF NCs with visible light photocatalytic characteristic for 4-nitrophenol degradation from aqueous solution, J. Hazard. Mater., 2019, 365, 921–931 CrossRef CAS PubMed.
- P. Xiao, R. Osuga and Y. Wang, et al., Bimetallic Fe–Cu/beta zeolite catalysts for direct hydroxylation of benzene to phenol: effect of the sequence of ion exchange for Fe and Cu cations, Catal. Sci. Technol., 2020, 10(20), 6977–6986 RSC.
- Y. Wu, X. Zhang and F. Wang, et al., Synergistic effect between Fe and Cu species on mesoporous silica for hydroxylation of benzene to phenol, Ind. Eng. Chem. Res., 2021, 60(23), 8386–8395 CrossRef CAS.
- L. Zeng, H. Liang and P. An, et al., Carbon encapsulated bimetallic FeCo nanoalloys for one-step hydroxylation of benzene to phenol, Appl. Catal., A, 2022, 633, 118499 CrossRef CAS.
- M. Garrido, M. Barrejón and J. A. Berrocal, et al., Polyaromatic cores for the exfoliation of popular 2D materials, Nanoscale, 2022, 14(25), 8986–8994 RSC.
- J. Du, S. Li and Z. Du, et al., Boron/oxygen-codoped graphitic carbon nitride nanomesh for efficient photocatalytic hydrogen evolution, Chem. Eng. J., 2021, 407, 127114 CrossRef CAS.
- X. Ma, Z. Deng and Z. Li, et al., A photothermal and Fenton active MOF-based membrane for high-efficiency solar water evaporation and clean water production, J. Mater. Chem. A, 2020, 8(43), 22728–22735 RSC.
- W. Yan, L. Yan and C. Jing, Impact of doped metals on urea-derived g-C3N4 for photocatalytic degradation of antibiotics: structure, photoactivity and degradation mechanisms, Appl. Catal., B, 2019, 244, 475–485 CrossRef CAS.
- Z. Deng, H. Yu and L. Wang, et al., Ferrocene-based metal-organic framework nanosheets loaded with palladium as a super-high active hydrogenation catalyst, J. Mater. Chem. A, 2019, 7(26), 15975–15980 RSC.
- X. Wang, F. Wang and C. Bo, et al., Promotion of phenol photodecomposition and the corresponding decomposition mechanism over g-C3N4/TiO2 nanocomposites, Appl. Surf. Sci., 2018, 453, 320–329 CrossRef CAS.
- Z. Deng, T. Wan and D. Chen, et al., Photothermal-Responsive Microporous Nanosheets Confined Ionic Liquid for Efficient CO2 Separation, Small, 2020, 16(34), 2002699 CrossRef CAS PubMed.
- M. Lu, Z. Pei and S. Weng, et al., Constructing atomic layer g-C3N4-CdS nanoheterojunctions with efficiently enhanced visible light photocatalytic activity, Phys. Chem. Chem. Phys., 2014, 16(39), 21280–21288 RSC.
- B. Van de Voorde, I. Stassen and B. Bueken, et al., Improving the mechanical stability of zirconium-based metal–organic frameworks by incorporation of acidic modulators, J. Mater. Chem. A, 2015, 3(4), 1737–1742 RSC.
- J. Shimei and W. Yue, Vibrational spectra of an open ferrocene and a half-open ferrocene, Spectrochim. Acta, Part A, 1999, 55(5), 1025–1033 CrossRef.
- S. Samanta, R. Yadav and A. Kumar, et al., Surface modified C, O co-doped polymeric g-C3N4 as an efficient photocatalyst for visible light assisted CO2 reduction and H2O2 production, Appl. Catal., B, 2019, 259, 118054 CrossRef CAS.
- X. Wei, N. Zhu and X. Huang, et al., Efficient degradation of sodium diclofenac via heterogeneous Fenton reaction boosted by Pd/Fe@Fe3O4 nanoparticles derived from bio-recovered palladium, J. Environ. Manage., 2020, 260, 110072 CrossRef CAS PubMed.
- P. P. Xu, L. Zhang and X. Jia, et al., A novel heterogeneous catalyst NH2-MIL-88/PMo10V2 for the photocatalytic activity enhancement of benzene hydroxylation, Catal. Sci. Technol., 2021, 11(19), 6507–6515 RSC.
- Y. Fang, L. Zhang and Q. Zhao, et al., Highly selective visible-light photocatalytic benzene hydroxylation to phenol using a new heterogeneous photocatalyst UiO-66-NH2-SA-V, Catal. Lett., 2019, 149, 2408–2414 CrossRef CAS.
- G. Grigoropoulou, J. H. Clark and J. A. Elings, Recent developments on the epoxidation of alkenes using hydrogen peroxide as an oxidant, Green Chem., 2003, 5(1), 1–7 RSC.
- S. Farahmand, M. Ghiaci and S. Asghari, Oxo-vanadium(IV) phthalocyanine implanted onto the modified SBA-15 as a catalyst for direct hydroxylation of benzene to phenol in acetonitrile-water medium: A kinetic study, Chem. Eng. Sci., 2021, 232, 116331 CrossRef CAS.
- P. Xiao, Y. Wang and J. N. Kondo, et al., Iron-and copper-exchanged Beta zeolite catalysts for hydroxylation of benzene to phenol with H2O2, Chem. Lett., 2018, 47(9), 1112–1115 CrossRef CAS.
- X. Chen, J. Zhang and X. Fu, et al., Fe-g-C3N4-catalyzed oxidation of benzene to phenol using hydrogen peroxide and visible light, J. Am. Chem. Soc., 2009, 131(33), 11658–11659 CrossRef CAS PubMed.
- Z. Ding, X. Chen and M. Antonietti, et al., Synthesis of transition metal-modified carbon nitride polymers for selective hydrocarbon oxidation, ChemSusChem, 2011, 4(2), 274–281 CAS.
- Y. Fang, L. Zhang and Q. Zhao, et al., Highly Selective Visible-Light Photocatalytic Benzene Hydroxylation to Phenol Using a New Heterogeneous Photocatalyst UiO-66-NH2-SA-V, Catal. Lett., 2019, 149, 2408–2414 CrossRef CAS.
- W. Han, W. Xiang and Z. Meng, et al., A novel Cr-doped CdS/ZnO nanocomposite for efficient photocatalytic hydroxylation of benzene to phenol, Colloids Surf., A, 2023, 670, 131529 CrossRef CAS.
- B. Han, X. Li and Z. Zhang, et al., A novel strategy to research the mechanism of rutile TiO2 with excellent photocatalytic performance, Nanotechnology, 2021, 33(3), 035704 CrossRef PubMed.
- F. Zhu, S. Zhou and M. Sun, et al., Heterogeneous activation of persulfate by Mg doped Ni(OH)2 for efficient degradation of phenol, Chemosphere, 2021, 131647 Search PubMed.
- S. J. Feng, B. Yue and Y. Wang, et al., Hydroxylation of benzene over V-HMS catalysts with the addition of Fe as the second metal component, Acta Phys.-Chim. Sin., 2011, 27(12), 2881–2886 CAS.
|
This journal is © The Royal Society of Chemistry 2023 |
Click here to see how this site uses Cookies. View our privacy policy here.