DOI:
10.1039/D3RA03136E
(Paper)
RSC Adv., 2023,
13, 18812-18815
Influence of the physical properties of the layered oxyselenides Bi2YO4Cu2Se2 through Ni doping
Received
11th May 2023
, Accepted 14th June 2023
First published on 20th June 2023
Abstract
We have synthesized a series of Ni-doped layered oxyselenides Bi2YO4Cu2−xNixSe2 (0 ≤ x ≤ 0.4). The crystal structure and physical properties were studied through X-ray diffraction, and electric and thermo transport measurements. We also performed DFT calculations to study the electric structure of the designed Bi2YO4Ni2Se2, which is similar to that of KNi2Se2.
Introduction
In recent decades, compounds with layered crystal structures have attracted people's increasing attention due to their interesting physical properties, such as superconductivity,1–3 charge density wave,4–9 topological insulation10 etc. In particular, the discovery of iron-based superconductors in 2008 aroused wide concern all over the world.2 Up to now, the mechanism of high-temperature superconductivity remains a mystery, which requires the joint efforts of theoretical and experimental researchers. According to previous research experience, high-temperature superconductors tend to adopt layered crystal structures. And it has been an important way to search for new superconductors through exploring new layered compounds.
Among the known iron-based superconductors, they all have the Fe2Pn2/Fe2Ch2 (Pn = P, As; Ch = S, Se, Te) conducting layer, which is believed playing an important role in the superconductivity. In fact, all the iron-based superconductors have analogues in CuCh based compounds. For example, the 1111-type LaOFeP/As and LaOCuS/Se adopt the same crystal structure.11,12 The 122-type KFe2Se2 and KCu2Se2, the 32
522-type Sr3Sc2O5Fe2As2 and Sr3Sc2O5Cu2S2 etc. All have the same situations.13–15 Many iron-based superconductors were discovered by substituting Fe2Pn2 layers for Cu2Ch2 layers in the known CuCh-based compounds. In 2002, a new layered CuSe-based compound Bi2YO4Cu2Se2 was reported and we studied the physical and electric properties lately.16–18 The Cu2Se2 layer is defined as the conducting layer and the Bi2YO4 layer is defined as the block layer. As every block layer provide one electron to conducting layer, which causes a mixed state of Cu2+/Cu+, it exhibits p-type metallic behaviour which is rare in CuCh-based compounds. Similarly, p-type metallic ground state is also found in KCu2Se2, in which the K layer also provides one electron to Cu2Se2 layer per unit cell. In 2012, the analogue KNi2Se2 was reported superconducting below 0.8 K, which has aroused people's extensive research interest.19 If the Cu2Se2 layer in Bi2YO4Cu2Se2 was replaced by Fe2Pn2 or Ni2Pn2 layers, we think new superconductors may be achieved. We try our best to synthesize Bi2YO4Ni2Se2 through solid state reaction. Unfortunately, all the attempts ended in failure. On the other hand, it is also valuable to study the influence of the physical proper-ties of the layered oxyselenide Bi2YO4Cu2Se2 through Ni doping at Cu site. In this paper, we successfully doped 20% Ni at Cu site in Bi2YO4Cu2Se2. The temperature coefficient of resistivity can be effectively adjusted through Ni doping. Furthermore, we studied the thermoelectric properties of the Ni-doped samples. Finally, we study the electric structure of the designed Bi2YO4Ni2Se2 through the first principle calculation, which exhibit similar electronic structure with KNi2Se2. This will tempt people to explore the synthesis of Bi2YO4Ni2Se2 through other methods.
Results and discussion
The crystal structure of Bi2YO4Cu2Se2 is shown in Fig. 1(a), which is alternatively stacked by Bi2YO4 layer and Cu2Se2 layer. Fig. 1(b) shows the crystal structure of KNi2Se2, which has the ThCr2Si2-type structure.20 Comparing these two structures, it is not difficult to find that their crystal structure is the same except the block layer. Fig. 2 shows the X-ray diffraction patterns of Bi2YO4Cu2−xNixSe2. All the diffraction peaks of the doped samples match the Bragg peaks of Bi2YO4Cu2Se2, which indicates high quality samples. The fitting lattice parameters of Bi2YO4Cu2−xNixSe2 are shown in Fig. 2(b). The lattice parameter a and c for Bi2YO4Cu2Se2 are 3.862 and 24.31 Å respectively, which is corresponding to previous results.16,17 The lattice parameter a is decreased with increasing Ni content, while the lattice parameter c is the opposite. It indicates that the in-plane interaction is strengthened and the interlayer interactions are weakened. The reported lattice parameters a and c of KCu2Se2 are 4.008 and 13.643 Å respectively.21 Compared with KCu2Se2, the thicker block layer in Bi2YO4Cu2Se2 makes the parameter a smaller. In other words, the in-plane interaction of Bi2YO4Cu2Se2 is stronger than that of KCu2Se2. For KNi2Se2, the fitted lattice parameter a and c according to experiment result are 3.89295 and 13.3158 Å respectively and the calculated lattice parameter a and c according to DFT calculation are 3.9680 and 13.0479 Å respectively.19,20,22 The lattice parameter a of KNi2Se2 is larger than that of Bi2YO4Cu2Se2. According to the above results, the lattice parameter a of Bi2YO4Ni2Se2, if it exists, should be larger than that of KNi2Se2. This may be the reason we cannot doped much more Ni in Bi2YO4Cu2Se2.
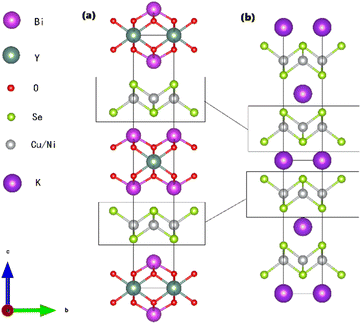 |
| Fig. 1 The crystal structure of Bi2YO4(Cu,Ni)2Se2 (a) and KNi2Se2 (b). | |
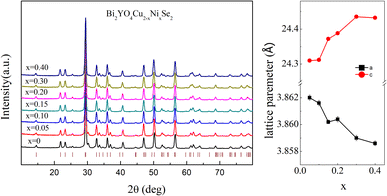 |
| Fig. 2 The X-ray diffraction patterns and the fitted lattice parameters of Bi2YO4Cu2−xNixSe2 measured at 300 K. | |
Fig. 3 shows the temperature dependence of the electric resistivity of Bi2YO4Cu2−xNixSe2. For Bi2YO4Cu2Se2, the resistivity increases with increasing temperature from 2 to 300 K, which indicates a metallic transport behaviour and is similar with previous results.17 The value of resistivity is increased with increasing Ni content. Compared with KNi2Se2, the resistivity is relatively higher. Unfortunately, no superconducting behaviour was found until 2 K for all the Ni doped Bi2YO4Cu2Se2. According to previous study,17 the metallic behaviour originates from the mixed valences of Cu ion in Cu2Se2 layer. Every Bi2YO4 unit provides one electron to Cu2Se2 unit, so every two Cu atoms only pro-vide 3 electrons to keep balance. While when Ni is doped at Cu site, every Ni atom can provide two electrons, namely electronic doping. For undoped Bi2YO4Cu2Se2, the carriers are mainly p-type. Regardless of other factors, Ni doping at Cu site decreases the carrier con-centration and the resistivity would increase with increasing Ni content. Our experimental results are consistent with the viewpoint. As shown in Fig. 3, the reduced resistivity increases with increasing Ni content. Furthermore, the Bi2YO4Cu1.6Ni0.4Se2 exhibits semiconducting/insulating transport behaviour from 2 to 300 K. The resistivity (x = 0–0.2) obey the Fermi liquid behaviour from 2 K to 50 K. We fitted the resistivity curves through the Fermi liquid behaviour equation ρ = ρ0 + AT2. Where ρ0 represents the residual resistivity. The results are shown in the inset (a) of Fig. 3. As can be seen, the residual resistivity in-creases with x. As known, the residual resistivity ρ0 is in direct proportion to m*ℏ/nτ0, where m*, n and ℏ/τ0 represent the carrier effective mass, carrier concentration and the scattering rate related to the disordered potential. For Bi2YO4Cu1.6Ni0.4Se2, it exhibits semiconducting transport behaviour that the resistivity decreases with arising temperature. Further study is needed to clarify the ground state of Ni doped Bi2YO4Ni2Se2. According to previous study, the ground state of KNi2Se2 is metallic.20 Our following DFT calculation result also indicates the metallic ground state of Bi2YO4Ni2Se2. It should not turn it to semiconductor through Ni doping. The semiconducting-like phenomenon should not be intrinsic, which may be caused by defect or grain boundary introduced with Ni doping.
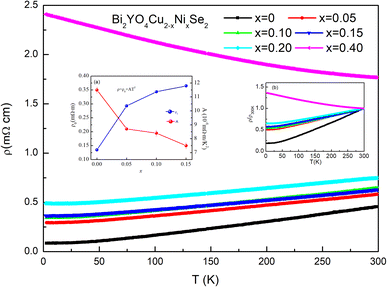 |
| Fig. 3 The temperature dependence of the resistivity of Bi2YO4Cu2−xNixSe2 from 2 to 300 K. Inset (a) is the parameter ρ0 and A fitted through the Fermi liquid behavior equation with different Ni con-tent. Inset (b) is the reduced resistivity of Bi2YO4Cu2−xNixSe2. | |
Fig. 4 shows the thermoelectric properties of Bi2YO4Cu2−xNixSe2 (x = 0, 0.2, 0.3). Fig. 4(b) shows the Seebeck coefficient curves of Bi2YO4Cu2−xNixSe2. The Seebeck coefficient also exhibits metallic behaviour from 100 to 300 K, increasing with temperature linearly according to the equation
where
kB is the Boltzmann constant,
m* is the effective mass of carrier, and
n is the carrier concentration, which applies for metals or degenerate semiconductors under assumption of parabolic band and acoustic phonon scattering approximation. The Seebeck coefficients are positive for all the samples ranging from 5 to 330 K, indicating p-type carriers dominated in these materials, which is consistent with previous results. The Seebeck coefficient decreases with increasing Ni content.
Fig. 3(c) shows the total thermal conductivity curves. The total thermal conductivity decreases with increasing Ni content. The total thermal conductivity can be divided into the electron component (
κe) and the lattice component (
κl):
κ =
κe +
κl. The electron component is proportional to the electrical conductivity according to Wide–Franz law,
κe =
LσT =
LT/
ρ. The decreasing resistivity indicates the electron component of the total thermal conductivity increase. Then the lattice thermal conductivity decreases with increasing Ni content, which originates from the increasing defect scattering induced by Ni. The dimensionless figure of merit is defined as
ZT =
S2/
ρκT, which is the measure of the efficiency of a thermoelectric material. We calculated the
ZT of the synthesized samples, which is shown in
Fig. 4(d). Although the induce of Ni de-crease the resistivity of the sample, the
ZT decrease with increasing Ni content because of the great degree reduction of the Seebeck coefficient. The introduction of Ni is unfavourable to the improvement of thermoelectric properties in Bi
2YO
4Cu
2Se
2.
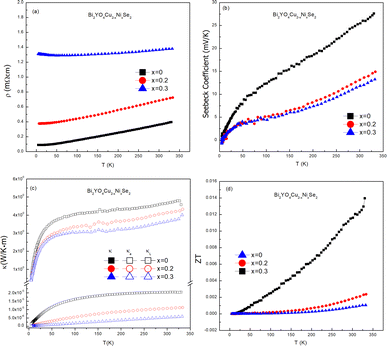 |
| Fig. 4 The thermoelectric properties of Bi2YO4Cu2−xNixSe2. | |
The plane-wave projector augmented method as implement in the Vienna ab initio simulation package (VASP) was used to calculate the density of states (DOS) and energy band. The Perdew–Bruke–Ernzerhof (PBE) form of generalized gradient approximation (GGA) was chosen as the exchange–correlation potential.23 Due to the ex-change–correlation effects of the strongly localized Cu and Ni 3d electrons, we adopted the DFT + U method. We tested different values of the screened Coulomb parameter U. The added Coulomb interactions do not change the ground state. An energy cut-off of 520 eV was used in all cases. The convergence criterion for energy was set to be 10−6 eV per unit cell and the forces on all relaxed atoms were less than 0.01 eV A−1. According to previous study, nonmagnetic states is adopted to calculate the electronic band structure. Fig. 5 shows the band structure of Bi2YO4Cu2Se2 and Bi2YO4Ni2Se2. The calculated band structure of Bi2YO4Cu2Se2 is consistent to previous result. The Fermi energy located at the valence band, indicating the p-type metallic ground state, which is consistent with the experiment results. Furthermore, as the replace of Cu by Ni, the Fermi energy move deeper in valence band, namely similar p type metallic ground state. The conduction band is attached to the valence band. The semiconducting behaviour in Ni-doped Bi2YO4Cu2Se2 observed in our experiment may be non-intrinsic. For Bi2YO4Cu2Se2, the bands exhibit complicated character near the Fermi surface. Along the Z–Γ line, the energy dispersion is very weak. The energy dispersion is strong in the layer-parallel directions, which originates from the strong hybridizations between Cu and Se. Similar quasi-two-dimensional properties are found in Bi2YO4Ni2Se2, which is consistent with KNi2Se2.20 There are also two bands crossing the Fermi energy, indicting the multi-band character. Compared the band structure of Bi2YO4Ni2Se2 and KNi2Se2, the structure of the valence band is very similar. Moreover, the thick Bi2YO4 layer make the 2D property more obvious. The ThCr2Si2-type super-conductor KNi2Se2 was discovered with a superconducting transition temperature of 0.8 K. We believe the Bi2YO4Cu2Se2 may be a superconducting material if it can be successful synthesized.
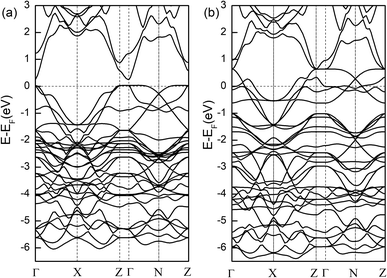 |
| Fig. 5 The band structure of Bi2YO4Cu2Se2 and Bi2YO4Ni2Se2. | |
Experimental
Bi2YO4Cu2−xNixSe2 sample was prepared by reacting a stoichiometric mixture of Bi2O3, Y2O3, Y, Cu, Ni and Se. The raw materials weighed by stoichiometric ratio were mixed. After thoroughly grounding in an agate pestle and mortar, the mixtures were pressed into pellets under 12 MPa. The pellets were then sealed under vacuum (<10−4 Pa) in the silica tubes which had been baked in drybox for 1–2 h at 150 °C. The ampoules were heated to 830 °C and maintained at this temperature for 24 h. Finally, the furnace is shut down and cools to room temperature naturally. The obtained samples were reground, pelletized, and heated for another 24 hours at 830 °C followed by furnace cooling. The X-ray powder diffraction patterns were recorded at room temperature on a PANalytical diffractometer (X'Pert PRO MRD) with Cu Kα radiation (40 kV, 40 mA). Structural refinement of powder samples was carried out by using Rietica software. The electrical resistivity and thermoelectric properties were measured using a Quantum Design PPMS (Physical Properties Measurement System) from 300 to 2 K.
Conclusions
In summary, we have synthesized a series of layered oxyselenides Bi2YO4Cu2−xNixSe2 through solid state reaction. There crystalline structures and physical properties were thoroughly studied. Along with the increase of Ni content, the resistivity increase. It exhibits semiconducting transport behaviour in Bi2YO4Cu1.6Ni0.4Se2, which may be caused by the diffraction of impurity or defects. The crystal structure and band structure of Bi2YO4Ni2Se2 are like those of KNi2Se2. We suspect that Bi2YO4Ni2Se2 should also exhibit superconductivity if it can be successfully synthesized.
Author contributions
Conceptualization, L. X. and J. W.; methodology, L. X.; validation, Z. L., W. K. and C. W.; formal analysis, Y. Z.; investigation, S. T.; resources, S. T.; data curation, L. X.; writing—original draft preparation, S. T.; writing—review and editing, L. X.; all authors have read and agreed to the published version of the manuscript.
Conflicts of interest
There are no conflicts to declare.
Acknowledgements
This work was supported by the Research Start-up Funds of Shandong University of Science and Technology (No. 415058).
Notes and references
- J. Chen, L. Hu, J. X. Deng and X. R. Xing, Chem. Soc. Rev., 2015, 44, 3522 RSC.
- Y. Kamihara, T. Watanabe, M. Hirano and H. Hosono, J. Am. Chem. Soc., 2008, 130, 3296 CrossRef CAS PubMed.
- Y. Mizuguchi, S. Demura, K. Deguchi, Y. Takano, H. Fujihiss, Y. Gotoh, H. Izawa and O. Miura, J. Phys. Soc. Jpn., 2012, 81, 114725 CrossRef.
- F. Weber, S. Rosenkranz, J. P. Castellan, R. Osborn, R. Hott, R. Heid, K. P. Bohnen, T. Egami, A. H. Said and D. Reznik, Phys. Rev. Lett., 2011, 107, 107403 CrossRef CAS PubMed.
- L. P. Gor’kov, Phys. Rev. B: Condens. Matter Mater. Phys., 2012, 85, 165142 CrossRef.
- X. Xi, Z. Wang, W. Zhao, J. H. Park, K. T. Law, H. Berger, L. Forró, J. Shan and K. F. Mak, Nat. Phys., 2016, 12, 139 Search PubMed.
- Y. Yang, S. Fang, V. Fatemi, J. Ruhman, E. Navarro-Moratalla, K. Watanabe, T. Taniguchi, E. Kaxiras and P. Jarillo-Herrero, Phys. Rev. B, 2018, 98, 035203 CrossRef CAS.
- E. Navarro-Moratalla, J. O. Island, S. Mañas-Valero, E. PinillaCienfuegos, A. Castellanos-Gomez, J. Quereda, G. RubioBollinger, L. Chirolli, J. A. Silva-Guillén, N. Agraït, G. A. Steele, F. Guinea, H. S. J. van der Zant and E. Coronado, Nat. Commun., 2016, 7, 11043 CrossRef CAS PubMed.
- S. C. de la Barrera, M. R. Sinko, D. P. Gopalan, N. Sivadas, K. L. Seyler, K. Watanabe, T. Taniguchi, A. W. Tsen, X. Xu, D. Xiao and B. M. Hunt, Nat. Commun., 2018, 9, 1427 CrossRef PubMed.
- S. Murakami, N. Nagaosa and S.-C. Zhang, Spin-Hall insulator, Phys. Rev. Lett., 2004, 93, 156804 CrossRef PubMed.
- Y. Kamihara, T. Watanabe, M. Hirano and H. Hosono, J. Am. Chem. Soc., 2008, 130, 3296 CrossRef CAS PubMed.
- Y. Kamihara, H. Hiramatsu, M. Hirano, R. Kawamura, H. Yanagi, T. Kamiya and H. Hosono, J. Am. Chem. Soc., 2006, 128, 10012 CrossRef CAS PubMed.
- M.-L. Liu, L.-B. Wu, F.-Q. Huang, L.-D. Chen and I.-W. Chen, J. Appl. Phys., 2007, 102, 116108 CrossRef.
- D. O. Scanlon and G. W. Watson, Chem. Mater., 2009, 21, 5435 CrossRef CAS.
- O. Tiedje, E. E. Krasovskii, W. Schattke, P. Stoll, C. Nather and W. Bensch, Phys. Rev. B: Condens. Matter Mater. Phys., 2003, 67, 134105 CrossRef.
- J. S. O. Evans, E. B. Brogden, A. L. Thompson and R. L. Cordiner, Chem. Commun., 2002, 912 RSC.
- S. G. Tan, D. F. Shao, W. J. Lu, B. Yuan, Y. Liu, J. Yang, W. H. Song, H. Lei and Y. P. Sun, Phys. Rev. B: Condens. Matter Mater. Phys., 2014, 90, 085144 CrossRef CAS.
- Y. Yang, J. Han, Z. Zhou, M. Zou, Y. Xu, Y. Zheng, C.-W. Nan and Y.-H. Lin, Adv. Funct. Mater., 2022, 32, 2113164 CrossRef CAS.
- R. N. James, L. Anna, V. S. Andreas, L. Wu, J. J. Wen, J. Tao, Y. M. Zhu, B. T. Zlatko, N. P. Armitage and M. M. Tyrel, Phys. Rev. B: Condens. Matter Mater. Phys., 2012, 86, 054512 CrossRef.
- F. Lu, J. Z. Zhao and W.-H. Wang, J. Phys.: Condens. Matter, 2012, 24, 495501 CrossRef PubMed.
- O. Tiedje, E. E. Krasovskii, W. Schattke, P. Stoll, C. Näther and W. Bensch, Phys. Rev. B: Condens. Matter Mater. Phys., 2003, 67, 134105 CrossRef.
- R. N. James and M. M. Tyrel, J. Am. Chem. Soc., 2012, 134, 7750 CrossRef.
- F. Ahmed, N. Tsujii and T. Mori, J. Mater. Chem. A, 2017, 5, 7545 RSC.
|
This journal is © The Royal Society of Chemistry 2023 |
Click here to see how this site uses Cookies. View our privacy policy here.