DOI:
10.1039/D3RA03427E
(Paper)
RSC Adv., 2023,
13, 25518-25528
Emulsions synergistic-stabilized by a hydroxyl sulfobetaine surfactant and SiO2 nanoparticles and their potential application for enhanced oil recovery†
Received
23rd May 2023
, Accepted 7th August 2023
First published on 25th August 2023
Abstract
The emulsions formed by conventional surfactants have poor stability in high temperature and high salinity reservoirs, which limits the fluidity control ability of emulsion flooding systems. Hydroxyl sulfobetaine surfactants have excellent emulsifying properties and can maintain good activity under high temperature and high salinity conditions. In this study, an emulsion synergistic-stabilized by hydroxyl sulfobetaine surfactant LHSB and SiO2 nanoparticles was reported for the first time, and the feasibility of its enhanced oil recovery was investigated. The results show that the stability, temperature and salt resistance of the emulsion were significantly improved after adding nanoparticles, which positively affected the exploitation of harsh reservoirs. The synergistic-stabilized mechanism between LHSB and SiO2 nanoparticles was revealed by the measurements of zeta potential, surface tension and contact angle. Moreover, core flooding experiments reflect the emulsion synergistic-stabilized by LHSB and SiO2 nanoparticles can effectively enhance oil recovery by 11.41%. This study provides an emulsion flooding system with excellent performance for enhanced oil recovery in harsh reservoirs.
1. Introduction
Chemical flooding is an important means to enhance oil recovery in oilfield development. In chemical flooding, the surfactant-stabilized emulsions can control the mobility of the oil displacement system to a certain extent, enhance the sweep efficiency, and play an important role in improving oil recovery.1–3 However, with the successive exploitation of reservoirs with high temperature and high salinity, the formation conditions are becoming more and more complex. In high temperature and high salinity environments, the emulsion formed by conventional surfactants cannot maintain good stability, which limits the fluidity control ability of the emulsion system.4–7 In contrast, emulsions synergistic-stabilized by solid nanoparticles and surfactants have unique advantages in solving this problem. The emulsion synergistic-stabilized by nanoparticles and surfactants through electrostatic adsorption or repulsion has excellent stability, which is not achieved by using surfactants alone.8–12 This synergistic effect enables the emulsion to maintain good fluidity control ability under high temperature and high salinity conditions. Therefore, the emulsion stabilized by nanoparticles and surfactants has a good application prospect for enhanced oil recovery in harsh reservoirs.
Hydroxyl sulfobetaine is a zwitterionic surfactant with outstanding emulsifying and foaming properties.13 Compared with conventional surfactants, hydroxyl sulfobetaine surfactants have great temperature and salt resistance, and can maintain good interfacial activity in a wide pH range.14–17 Such excellent performances suggest their potential application in high temperature and high salinity formations. Further, if there is a certain effect between hydroxyl sulfobetaine surfactants and nanoparticles, the synergistically stable emulsions may be suitable for formation environments with higher temperature and salinity, which is of great significance for the exploitation of harsh reservoirs. However, due to the unique internal salt structure, these surfactants do not have the ability to accept or release protons in almost all pH ranges, and generally have a neutral net charge.18,19 Accordingly, there are few reports about hydroxyl sulfobetaine surfactants and nanoparticles synergistically stabilizing emulsion through electrostatic adsorption or repulsion to enhance oil recovery.
Interestingly, Baptists and Cuccovia et al. reported that the presence of opposite charges in zwitterionic surfactants leads to large dipole moments in headgroups, which gives rise to interactions with both cations and anions.20,21 Moreover, in the study of Perttu et al., it was pointed out that zwitterionic sulfobetaine lipids have an anionic sulfonate that extends to the aqueous medium and a cationic amine adjacent to the bilayer, which has the properties of anionic groups to a certain extent.22 In the zeta potential test, the surface potential of sulfobetaine lipids was negative even at high cation concentration. Meanwhile, the study of Ji et al. also showed that there was a certain electrostatic interaction between hydroxyl sulfobetaine and ions.23 These provide support for the synergistic effect of hydroxyl sulfobetaine surfactants and nanoparticles. Regarding the aim of this study, as a surfactant with good activity in high temperature and high salinity reservoirs, the synergistic effect of hydroxyl sulfobetaine and nanoparticles may lead to a further improvement in the temperature and salt resistance of emulsions. Therefore, the aim of this study was to investigate the feasibility of emulsions synergistic-stabilized by hydroxyl sulfobetaine and nanoparticles and their potential application for enhanced oil recovery.
In this paper, a hydroxyl sulfobetaine surfactant (laurelamide propyl hydroxyl sulfobetaine, abbreviated as LHSB) and SiO2 nanoparticles stabilized emulsions were studied, and the oil displacement effect of the emulsions was evaluated. The corresponding molecular structure of this surfactant is shown in Fig. 1. Compared with the emulsion stabilized by LHSB, the emulsion synergistic-stabilized by LHSB and SiO2 nanoparticles has better stability and can also exist stably under high temperature and salinity conditions, which has a positive effect on enhancing oil recovery. This study provides an emulsion flooding system with excellent performance for enhanced oil recovery in harsh reservoirs.
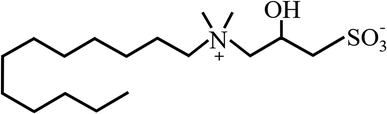 |
| Fig. 1 Chemical structure of the surfactant used. | |
2. Experimental section
2.1. Materials
Laurelamide propyl hydroxyl sulfobetaine was purchased from Linyi Lusen Chemical company with a purity of 35%. SiO2 nanoparticles of 99.9% purity were purchased from Shanghai Xiaoge Nano Material Company, which have a primary particle diameter of 20 nm according to the supplier. The particle size distribution determined by dynamic light scattering using a Zetasizer Nano ZS ZEN3600 instrument is shown in Fig. S1,† yielding an average particle diameter of 418 nm, attributed to the nanoparticle agglomeration in aqueous. Liquid paraffin oil was purchased from Sinopharm Chemical Reagent Co. All other chemicals were analytically pure and purchased from Sinopharm Chemical Reagent Co.
2.2. Preparation and characterization of emulsions
Firstly, 0.5 wt% SiO2 nanoparticles were dispersed in 10 mL LHSB solution with a certain concentration in a 40 mL glass bottle. After ultrasonic dispersion for 2 minutes, LHSB/SiO2 dispersions were obtained. Then 10 mL oil phase (liquid paraffin oil) was added to 10 mL LHSB aqueous solutions or 10 mL LHSB/SiO2 dispersions, and homogenized at 15
000 rpm for 2 minutes to gain emulsions. The particle and surfactant concentrations were expressed as weight percentage (wt%) and moles per liter (mol L−1) relative to the aqueous phase, respectively.
The method for determining the type of emulsion was as follows: the type of emulsion was determined by drop test. The prepared emulsion was dropped into water. If the emulsion droplets can spread rapidly, it is an O/W emulsion; if it cannot spread, it is a W/O emulsion.
The stability of emulsions was expressed by the volume fraction of the water phase, the oil phase and the size of the emulsion droplets. The appearance photos of the emulsion and the microscopic photos of the emulsion droplets at different standing times were recorded by digital camera and optical microscope.
2.3. Measurements
2.3.1 Zeta potential of particles in water. 0.5 wt% SiO2 nanoparticles were dispersed in 10 mL LHSB solution with a certain concentration in a 40 mL glass bottle. After ultrasonic dispersion for 2 minutes, LHSB/SiO2 dispersion was obtained. After the dispersions were placed for 24 h, the zeta potential of the SiO2 nanoparticles was then measured with a Zetasizer Nano ZS ZEN3600 instrument (Malvern Company). Measured 3 times at 25 °C, take the average.
2.3.2 Surface tension. The air–water surface tensions of surfactant solutions and dispersions were measured by QBZY series automatic surface tension meter at 25 °C. Each measurement was performed at least three times and the average was recorded.
2.3.3 Contact angle. The wettability of SiO2 nanoparticles modified by different concentrations of LHSB was measured by contact angle experiment. ① Preparation of test samples: the glass slides were washed with acetone, deionized water and absolute ethanol in turn for 10 min under the action of water bath ultrasound. After cleaning, the glass slides were dried in the air for backup use. The LHSB/SiO2 dispersion was uniformly sprayed onto the treated glass slides using a spray gun with a nozzle diameter of 0.5 mm. After spraying, it was placed in the oven to dry, and the modified particle surface was obtained. ② Measurement of contact angle: keep the surface of the test sample flat and dry, and use the JY-82B Kruss DSA contact angle test instrument to measure the contact angle. The test liquid was deionized water, and about 5 μL of water droplets hanging in the needle naturally fell to the surface of the test sample. Each sample was measured for 3 times and averaged.
2.4. Core flooding tests
In order to evaluate the effect of emulsion enhanced oil recovery under reservoir conditions, core flooding tests were carried out. First, the dry core was vacuumized for 6 h, and then saturated with water and oil. After aging at 70 °C for 24 h, water injection was started. When the water cut was higher than 98%, emulsion flooding would be carried out (0.5 PV). Finally, water flooding was carried out again until the water cut was greater than 98%. The temperature of the whole displacement process was 70 °C.
3. Results and discussion
3.1. Emulsions stabilized solely with LHSB
Firstly, the surface tension of surfactant LHSB was measured, as shown in Fig. S2.† It can be seen that the critical micelle concentration (cmc) of LHSB and the surface tension at cmc were about 1.8 × 10−3 mol L−1 and 36.8 mN m−1, respectively. Then the stability of LHSB stabilized emulsions was investigated. A series of LHSB surfactant solutions with different concentrations were selected, and liquid paraffin oil was used as the oil phase to prepare O/W emulsions stabilized by LHSB (Fig. 2). As shown in Fig. 2a, demulsification occurred in the emulsion with LHSB concentration less than 0.3 mmol L−1 after 24 h of preparation. As the standing time was extended to 3 days, only the emulsions with LHSB concentrations of 0.6 mmol L−1 and 1 mmol L−1 remained stable (Fig. 2b). From the microscopic image shown in Fig. 2c, it can be seen that the diameter of the emulsion droplets decreased with increasing surfactant concentration. This is because LHSB molecules can be adsorbed on the oil–water interface to form an interface film, which has a protective effect, so that the emulsion droplets are not easy to coalesce when they collide with each other. With the increase of surfactant concentration, the adsorption amount of LHSB molecules at the oil–water interface raised, which meant that the strength of the interface film enhanced, and the difficulty of emulsion droplets coalescence increased, resulting in smaller diameters. In addition, for ionic surfactants, the increase of concentration led to more charges being adsorbed at the interface film. The increase of electrostatic repulsion between the droplet–droplet inhibited coalescence of the emulsion droplets, which also led to a decrease in the droplet diameters. It is accepted that the emulsion droplets were more uniform and smaller, indicating emulsions were more stable.10,11 Among them, the droplet diameters stabilized by 0.6 mmol L−1 and 1 mmol L−1 LHSB were much smaller than that of 0.3 mmol L−1, but the difference between 0.6 mmol L−1 and 1 mmol L−1 was not obvious. It can be concluded that when the concentration of LHSB was low, emulsions could not be formed in the system. When the LHSB concentration increased to 0.6 mmol L−1, stable emulsions could be obtained, and the average droplet diameter was about 66.15 μm. The particle size distribution of emulsion stabilized by 0.6 mmol L−1 LHSB is shown in Fig. S3.†
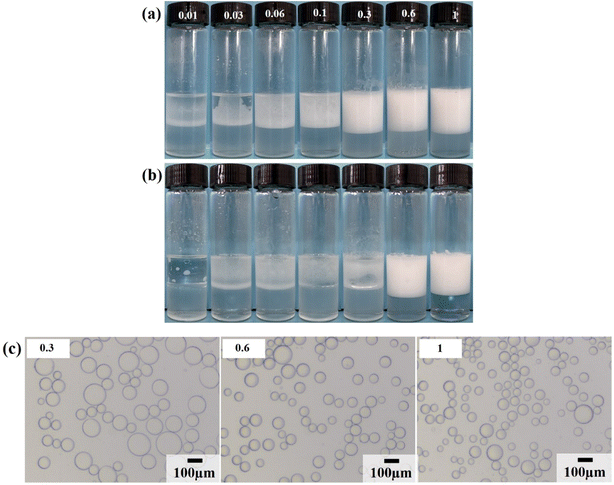 |
| Fig. 2 Digital photos and micrographs of emulsions stabilized by LHSB solely. (a) Taken 24 h, and (b) taken 3 days after preparation. The concentration of LHSB from left to right: 0.01, 0.03, 0.06, 0.1, 0.3, 0.6 and 1 mmol L−1. (c) Selected micrographs of emulsions in (a). | |
3.2. LHSB and SiO2 nanoparticles synergistic-stabilized emulsions
Due to the strong hydrophilicity of SiO2 nanoparticles, emulsions could not be formed in the system when they were used as emulsifiers independently (see the first photo in Fig. 3a). This is as reported in most studies.10,11,24,25 Based on the above results, it can be seen that the LHSB alone could not stabilize the emulsion well at low concentrations (less than 0.6 mmol L−1). However, when both LHSB and SiO2 nanoparticles were used as emulsifiers, stable O/W emulsions can be formed at a surfactant concentration of 0.3 mmol L−1. As shown in Fig. 3a, after adding 0.5 wt% SiO2 nanoparticles to the surfactant aqueous solution, the stability of the emulsion was significantly better than that of the emulsion stabilized by LHSB solely. Even at low concentrations of LHSB (0.01–0.1 mmol L−1), the emulsions after 3 days of homogenization were not completely demulsified, and only part of the oil phase was released. When the concentration of LHSB was 0.3 mmol L−1, stable emulsions could be obtained. Meanwhile, there was little change in the appearance of the emulsions after 3 days of preparation (Fig. 3b), which could not be achieved using LHSB alone. The micrographs of the emulsions synergistic-stabilized by 0.5 wt% SiO2 nanoparticles and LHSB were shown in Fig. 3c. It can be seen that as the surfactant concentration increased from 0.3 mmol L−1 to 1 mmol L−1, the average droplet diameter of the emulsion also decreased from 48.40 μm to 29.12 μm. Compared with the emulsion stabilized by LHSB, the droplet size of the emulsion containing SiO2 nanoparticles was significantly smaller, indicating that the emulsion was more stable. This is mainly attributed to the synergistic effect between SiO2 nanoparticles and LHSB which increased the stability of the emulsion. Moreover, there may be some SiO2 nanoparticles in the aqueous phase in the emulsions. The electrical repulsion between particles can increase the inter-droplets spacing and thus hinder coalescence of the emulsion droplets.12 It can be seen from Fig. S4† that the emulsions still did not occur demulsification after 1 month of preparation, but the droplet diameters increased slightly, which reflected that the emulsions prepared by LHSB and SiO2 nanoparticles had outstanding stability.
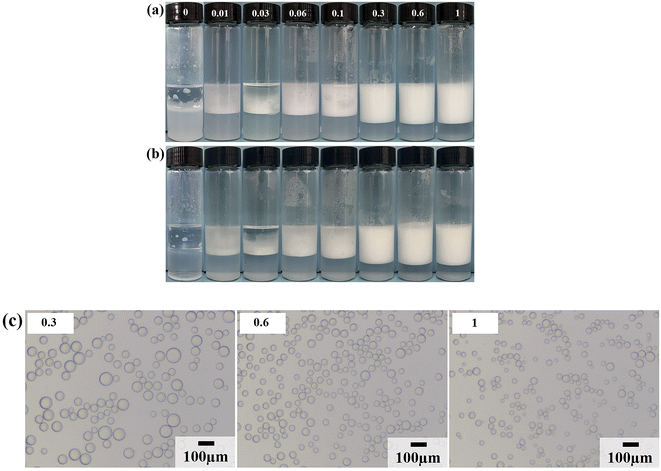 |
| Fig. 3 Digital photos and micrographs of emulsions synergistic-stabilized by LHSB and SiO2 nanoparticles (0.5 wt%). (a) Taken 24 h, and (b) taken 3 days after preparation. The concentration of LHSB from left to right: 0.01, 0.03, 0.06, 0.1, 0.3, 0.6 and 1 mmol L−1. (c) Selected micrographs of emulsions in (a). | |
The effects of the dosage of SiO2 nanoparticles on the stability of the emulsions were investigated, as shown in Fig. 4, where the concentration of LHSB was 0.3 mmol L−1. As shown in Fig. 4a, the emulsions with different concentrations of nanoparticles did not break after 24 h of preparation, and there was no obvious difference in water separation rate. With the increase of SiO2 nanoparticle concentration, the water phase gradually became turbid, especially when the concentration was 0.7 wt% and 1.0 wt%. When the particle concentration is too high, a large number of nanoparticles will be dispersed in the continuous phase, and then enter the aqueous phase with the creaming.26 It can be seen from Fig. 4b that the dosage of SiO2 nanoparticles had obvious effects on the droplet size. With the increase of nanoparticles concentration from 0.1 wt% to 1.0 wt%, the average size of emulsion droplets gradually decreased from 91.59 μm to 36.76 μm. When the concentration of nanoparticles increased to 0.5 wt%, the droplet diameters were less affected by particle concentrations. It indicates that most of the added nanoparticles can cooperate with surfactants to stabilize the emulsion at this concentration. These nanoparticles may act on the oil–water interface or the continuous phase, but no matter what mechanism, this synergy has the maximum effect. When the particle concentration continues to rise, the synergistic effect between the added particles and surfactants gradually weakens, which leads to a small change in the droplet diameters.27 Considering the practical application in engineering, when the concentration of LHSB is 0.3 mmol L−1, the appropriate concentration of SiO2 nanoparticles is 0.5 wt%.
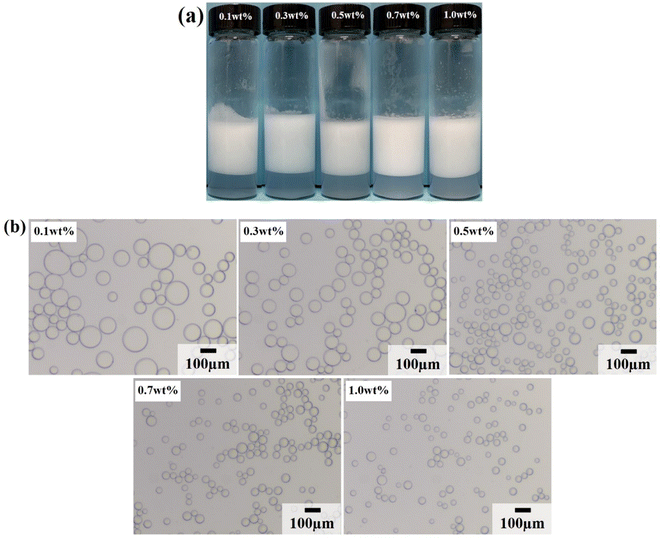 |
| Fig. 4 Digital photos (a) and micrographs (b) of emulsions synergistic-stabilized by LHSB (0.3 mmol L−1) and SiO2 nanoparticles taken 24 h after preparation. The concentration of SiO2 nanoparticles from left to right: 0.1, 0.3, 0.5, 0.7, and 1.0 wt%. | |
3.3. The effects of temperature and salinity on emulsions
3.3.1 Temperature. Temperature is an important factor affecting the stability of emulsion, because most reservoirs have high temperature characteristics.28 The stability of emulsions at different heating temperatures was investigated, as shown in Fig. 5. With the increase of temperature, the two emulsions showed different demulsification. Firstly, for the emulsion stabilized by LHSB solely, the increase of temperature had a great influence on its stability. The demulsification speed of emulsion accelerated obviously with the increase of temperature. After heating for 120 min, about 10% of oil in the emulsion was released at 35 °C, and then raised to 50% at 45 °C. When the heating temperature further raised to 55 °C and 65 °C, the emulsion completely broke after 60 min, and the time required for complete phase separation shortened with the increase of temperature. It can be seen that the emulsion stabilized by LHSB independently was sensitive to temperature changes. On the one hand, the collision probability between droplet–droplet caused by Brownian motion increased at high temperatures, which led to the coalescence of emulsion droplets; on the other hand, with the rise in temperature, the solubility of surfactant LHSB in the continuous phase increased, and the LHSB molecules adsorbed at the oil–water interface reduced, which led to the decrease of interfacial film strength and the deterioration of emulsion stability.29 In contrast, the demulsification speed of the emulsion stabilized by LHSB and SiO2 nanoparticles was significantly slower. When the temperature rose to 35 °C and 45 °C, the emulsion did not appear demulsification after heating for 120 min, and no oil was released. When the temperature rose to 55 °C and 65 °C, only 5% and 10% of oil in the emulsion was released, respectively. The emulsion was relatively insensitive to temperature changes. It is attributed to the synergistic effect between the SiO2 nanoparticles and LHSB, whether electrostatic adsorption or repulsion, which increased the strength of the oil–water interface film at high temperatures. Compared with LHSB stabilized emulsion, the emulsion prepared by LHSB and SiO2 nanoparticles exhibited better temperature resistance, which indicated that it was more suitable for high temperature reservoirs.
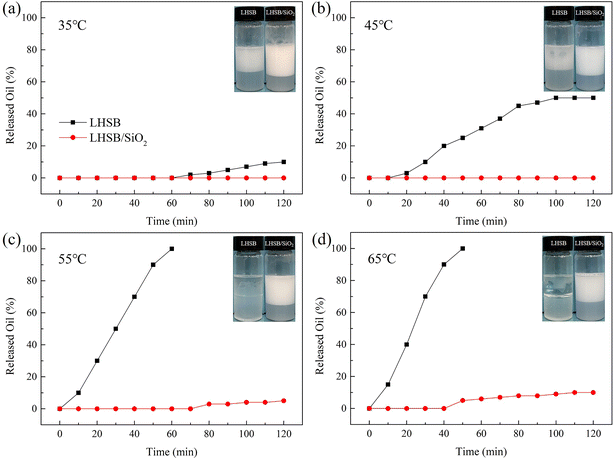 |
| Fig. 5 Time dependence of released oil for emulsions at different temperatures: (a) 35, (b) 45, (c) 55 and (d) 65 °C (0.3 mmol L−1 LHSB, 0.5 wt% SiO2 nanoparticles). | |
3.3.2 Salinity. In fact, the water in the reservoirs has high salinity and conventional emulsions are difficult to maintain stability under high salinity conditions.30,31 In the formation, the ion components of high salinity water are mainly sodium ions and chloride ions. Therefore, the stability of the emulsion under different salinity conditions was further investigated by preparing aqueous solutions with different concentrations of NaCl (Fig. 6). As shown in Fig. 6a, when the NaCl concentration in the system was 0.01 mol L−1, the emulsion could not be formed by LHSB solely. When SiO2 nanoparticles were added to the system, even at a NaCl concentration of 0.5 mol L−1, the emulsion after one day of preparation only showed a slight demulsification, as shown in Fig. 6b. This indicates that the addition of SiO2 nanoparticles greatly improved the salt resistance of the emulsion. The reason is that NaCl can promote the adsorption of SiO2 nanoparticles at the oil–water interface. With appropriate addition of NaCl, the potential on the surface of SiO2 nanoparticles decreased, the repulsion between particles weakened, and the particles exhibited a micro-flocculation state, which greatly promoted the adsorption of particles at the oil–water interface.26,32 At this point, the stability of the emulsion was determined by the attached SiO2 nanoparticles and surfactant adsorbed at the oil–water interface. In addition, it is found in Fig. 6b that the aqueous phase was clear and transparent, which indicated that the SiO2 nanoparticles gradually entered the emulsion layer from the aqueous phase. Generally, the stability of emulsions decreases with the increase of NaCl concentration.10,24 As shown in Fig. 6c, the droplet diameters increased with increasing NaCl concentration, indicating that the stability of the emulsion was weakened. It is worth noting that when the NaCl concentration was 0.1 mol L−1, the average droplet diameter was about 50.03 μm, which was similar to that without NaCl (48.40 μm). This means that when NaCl concentration was less than 0.1 mol L−1, the emulsion was almost unaffected by NaCl and still maintained good stability. As the NaCl concentration further rose to 0.3 mol L−1 and 0.5 mol L−1, the droplet size gradually increased, and the average droplet diameters were 79.32 μm and 219.13 μm, respectively. Correspondingly, in Fig. 6b, the water separation rate of emulsions containing 0.3 mol L−1 and 0.5 mol L−1 NaCl was higher than that of other emulsions, further indicating that the stability of emulsions was reduced. On the one hand, when the salt concentration was high, the diffused double layer of the hydrophilic group of LHSB would be excessively compressed, resulting in a low activity and a tendency to diffuse into the dispersed phase. Subsequently, the adsorption of LHSB molecules at the oil–water interface decreased, resulting in the coalescence and stratification of the emulsion, and thus the water separation rate increased.33 On the other hand, the addition of excessive NaCl led to the decrease of interfacial adsorption of SiO2 nanoparticles, because the particles flocculation was aggravated greatly, which facilitated desorption of the particles at the interface.32
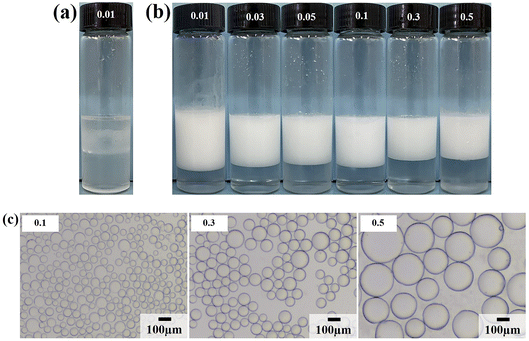 |
| Fig. 6 Effects of naCl on emulsion stability. (a) Digital photo of emulsion stabilized by 0.3 mmol L−1 LHSB taken 2 h after preparation. (b) Digital photos of emulsions synergistic-stabilized by 0.3 mmol L−1 LHSB and 0.5 wt% SiO2 nanoparticles were taken 24 h after preparation. (c) Selected micrographs of emulsions in (b). | |
In a word, the emulsion synergistic-stabilized by LHSB and SiO2 nanoparticles exhibits superior temperature and salinity tolerances, which is expected to be applied in unconventional reservoirs with high temperature and high salinity.
3.4. Synergistic-stabilized mechanism
Most studies have shown that the interaction between surfactants and nanoparticles and the activity of surfactants at the oil–water interface are mainly controlled by electrostatic adsorption or repulsion.9–12,34 In order to reveal the synergistic-stabilized mechanism between LHSB and SiO2 nanoparticles, the zeta potential, surface tension and contact angle were monitored. It is known that the measured zeta potential of SiO2 nanoparticles dispersed in deionized water was −29.9 mV (Fig. S5†). As shown in Fig. 7, the zeta potential of SiO2 nanoparticles dispersed in LHSB aqueous solutions rose slightly with the increase of LHSB concentration. When the concentration of LHSB was 0.03 mmol L−1, the zeta potential was −29.7 mV, which was similar to that without LHSB. However, when the concentration of LHSB increased to 1 mmol L−1, the zeta potential was −25.4 mV. This indicates that there was a weak adsorption between LHSB and SiO2 nanoparticles at relatively high concentrations. When surfactants are adsorbed on the surface of the nanoparticles, the electrostatic repulsion between the particles is weakened, and the absolute value of the zeta potential decreases.35,36 The reason is that there are only three carbon atoms between the positive and negative charges in the LHSB head group, which cannot form a ring structure to achieve mutual neutralization.37 Therefore, LHSB molecules can adsorb negatively charged SiO2 nanoparticles to a certain extent through the positively charged quaternary ammonium group in the head group. On the whole, this adsorption was very weak, almost only when the concentration of LHSB was high. It is attributed to the zwitterionic structure of LHSB molecules in aqueous solution. The electrostatic attraction between quaternary ammonium groups and SiO2 nanoparticles was significantly weakened due to the presence of negatively charged sulfonic acid groups, resulting in a weak adsorption of LHSB on the surface of SiO2 nanoparticles. Moreover, it can be seen from Fig. 7 that the zeta potential difference of SiO2 nanoparticles was only 2.6 mV before and after adding 0.3 mmol L−1 LHSB. Obviously, the adsorption amount of 0.3 mmol L−1 LHSB on the surface of SiO2 nanoparticles was too small, and it was difficult to form a tight monolayer on the surface of the particles to significantly improve the surface activity of the particles. Combined with the above experimental phenomena, it is considered that the weak adsorption between LHSB and SiO2 nanoparticles was not the main factor for synergistic stabilization of emulsion. Therefore, we speculated that LHSB and SiO2 nanoparticles may cooperate to stabilize the emulsion through electrostatic repulsion.
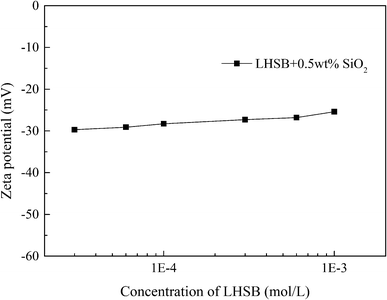 |
| Fig. 7 Zeta potential of 0.5 wt% SiO2 nanoparticles as a function of LHSB concentration. | |
In order to verify this conjecture, the change of the surface tension of the system with the concentration of LHSB was investigated, as shown in Fig. 8. Compared with LHSB aqueous solution, the surface tension of the system was obviously reduced after adding 0.5 wt% SiO2 nanoparticles. It indicates that there was a repulsion effect between LHSB and SiO2 nanoparticles. Under the effect of electrostatic repulsion, the rate of surfactant migration to the interface increased and thus the surface tension decreased.38 With the increase of LHSB concentration, the difference of surface tension between LHSB/SiO2 dispersion and LHSB aqueous solution reduced gradually. When the concentration was higher than 3 mmol L−1, the difference was almost non-existent. The reason is that there were both electrostatic repulsions and adsorption between LHSB and SiO2 nanoparticles. When the concentration of LHSB was high, the LHSB molecules adsorbed on the surface of SiO2 nanoparticles increased, which weakened the repulsion effect between the particles and the surfactant, and thus reducing the difference of surface tension. The change curve of the surface tension of the supernatant in Fig. 8 also proves this view. When LHSB/SiO2 dispersions with different LHSB concentrations were standing for 48 h, the surface tension of the supernatant was measured. The results show that with the increase of LHSB concentration, the surface tension of the supernatant was more than that of LHSB aqueous solution to a certain extent, which was evident at high concentrations such as 3 mmol L−1. The reason is that some LHSB molecules were adsorbed on the surface of SiO2 nanoparticles and then settle to the bottom of the dispersion, so that the LHSB molecules in the supernatant decreased and the surface tension increased. Moreover, it can also be seen from Fig. 8 that the adsorption effect was obvious only when the concentration of LHSB was relatively high, which was consistent with the zeta potential measurement results. In consequence, LHSB molecules and SiO2 nanoparticles can synergistically stabilize the emulsion at low concentrations mainly through electrostatic repulsion.
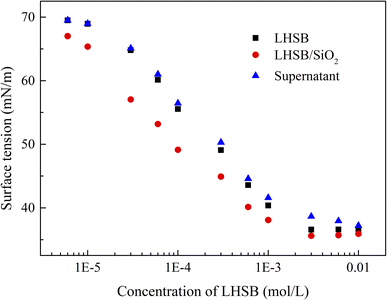 |
| Fig. 8 Surface tension of LHSB aqueous solutions (■), LHSB/SiO2 dispersions (●) and the supernatant of LHSB/SiO2 dispersions after standing for 48 h (▲), as a function of LHSB concentration. | |
In order to further determine that the weak adsorption between LHSB and SiO2 nanoparticles is not the dominant factor in stabilizing the emulsion, the change in the wettability of SiO2 nanoparticles modified with LHSB was investigated. The modified particle surface was obtained on the glass slide by spraying method, and then the water contact angle of the surface at different LHSB concentrations was measured, as shown in Fig. 9. The results show that there was almost no difference in the contact angle at different LHSB concentration, especially when the concentration was less than cmc. This indicates that the presence of LHSB hardly changed the hydrophobicity of SiO2 nanoparticles. Therefore, there was no obvious adsorption behavior between LHSB and SiO2 nanoparticles at low LHSB concentrations, which was consistent with the results of zeta potential and surface tension measurements.
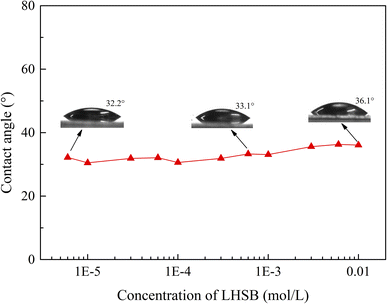 |
| Fig. 9 The water contact angle of the particle surface modified by different concentrations of LHSB at room temperature. | |
Based on the above results, the mechanism of LHSB cooperating with SiO2 nanoparticles to stabilize the emulsion can be revealed. Firstly, there is a large dipole moment in the head group of LHSB molecule, which makes it exhibit a certain electronegativity as a whole, thus repelling each other with SiO2 nanoparticles.20,21 Under the effect of electrostatic repulsion, the migration rate of LHSB molecules to the oil–water interface is increased, and the adsorption of LHSB at the interface is promoted, which makes the oil–water interfacial tension significantly reduced, and the emulsion droplets are electronegative. Secondly, when similarly charged SiO2 nanoparticles are added, they disperse evenly in the aqueous phase in the emulsions, each being surrounded by an electrical double layer. The electrical repulsions between the droplet–droplet, particle–droplet, and particle–particle inhibit flocculation and coalescence of the emulsion droplets. Furthermore, double-layer repulsion between particles and between particles and charged droplets leads to a thick aqueous lamellae, which increases the inter-droplets spacing and thus reduces the van der Waals attraction between the droplets.39 Finally, there is a weak adsorption between some LHSB molecules and SiO2 nanoparticles, which can also enhances the stability of the emulsion to some extent. Fig. 10 shows the synergistic-stabilized mechanism.
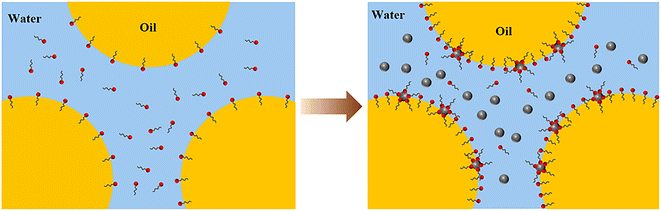 |
| Fig. 10 The synergistic-stabilized mechanism of emulsion by LHSB and SiO2 nanoparticles. | |
3.5. Core flooding studies
In order to explore the potential of the emulsions in enhanced oil recovery, core displacement experiments were carried out. The basic parameters of artificial core and oil displacement results are shown in Table 1, and the dynamic flooding curve is shown in Fig. 11. The temperature of the whole experimental process was 70 °C, and the injected volume of the emulsions in the chemical flooding stage was 0.5 PV. After injecting emulsions stabilized by LHSB, the effect of chemical flooding to enhance oil recovery was not well, only 4.79%. The reason is that the LHSB stabilized emulsions could not remain stable under high temperatures, and the demulsification occurred during the displacement process. After demulsification, surfactant solution alone could not sweep to the large remaining oil area due to the high mobility ratio, and then a serious fingering phenomenon occurred during the flooding process. This means that the sweep efficiency of the oil displacement was low, which led to a limited increment in the oil recovery rate. When injected with LHSB/SiO2 stabilized emulsions, the oil recovery was significantly increased by 11.41%, which should be ascribed to the outstanding stability of the emulsions at high temperatures. As shown in Fig. 11, the injection pressure rose dramatically in the emulsion flooding stage, which was attributed to the fact that the O/W emulsions blocked the high permeability channel through the Jamin effect and thus increased the flow resistance. Accordingly, the continuously injected emulsion turned to the unswept area, improving the sweep efficiency and thus enhancing oil recovery. In addition, the prepared emulsions can be dissolved in heavy oil and reduce the viscosity of heavy oil, which is conducive to enhance oil recovery.
Table 1 Summary of chemical flooding in core flooding tests
Permeability/(10−3 μm2) |
Initial oil saturation/(%) |
Emulsion flooding system |
Oil recovery/(%) |
Water flooding |
Chemical flooding |
Final recovery |
602 |
79.53 |
LHSB |
46.51 |
4.79 |
54.87 |
557 |
77.25 |
LHSB/SiO2 |
47.03 |
11.41 |
61.74 |
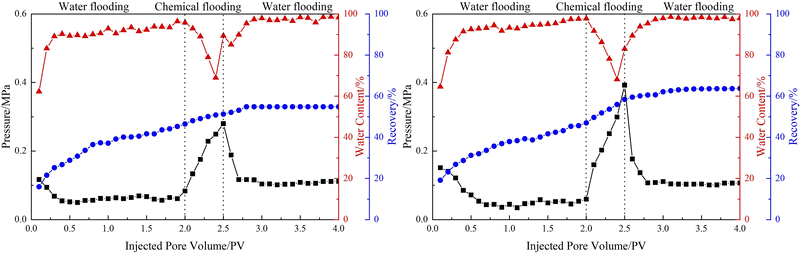 |
| Fig. 11 The results of emulsion flooding: (left) LHSB stabilized emulsions, (right) LHSB/SiO2 stabilized emulsions. | |
4. Conclusion
In summary, we studied an emulsion stabilized by hydroxyl sulfobetaine surfactant LHSB and negatively charged SiO2 nanoparticles. The surfactant LHSB has excellent emulsifying properties and can maintain good activity under high temperature and high salinity conditions. However, compared with the emulsion stabilized by LHSB independently, the emulsion synergistic-stabilized by LHSB and SiO2 nanoparticles had better stability and temperature and salt resistance. By exploring the synergistic-stabilized mechanism, it was found that the electrostatic repulsion between LHSB molecules and SiO2 nanoparticles and between SiO2 nanoparticles was crucial to the stability of the emulsion. In addition, in the core flooding test, the emulsion flooding system stabilized by LHSB and SiO2 nanoparticles effectively improved the displacement efficiency and sweep efficiency, and enhanced oil recovery by 11.41%. This study proved the feasibility of hydroxyl sulfobetaine surfactant in collaboration with nanoparticles to stabilize the emulsion, and provided a promising emulsion flooding system for enhanced oil recovery in harsh reservoirs.
Conflicts of interest
The authors declare that they have no known competing financial interests or personal relationships that could have appeared to influence the work reported in this paper.
Acknowledgements
The authors are grateful for funding from the China Postdoctoral Science Foundation (No. 2022M721396), the Natural Science Foundation of Jiangsu Province (No. BK20220622), the Basic Science (Natural Science) Research Projects of Colleges and Universities in Jiangsu Province (No. 22KJB440001), the major project of universities affiliated to Jiangsu Province Basic Science (Natural Science) Research (No. 23KJA440001), the Sci & Tech Program of Changzhou (No. CE20225065), the Postgraduate Research & Practice Innovation Program of Jiangsu Province (No. SJCX23_1564), and the Industry-University-Research Collaboration Program supported by Science and Technology Department of Jiangsu Province (BY2022963).
References
- M. S. Karambeigi, R. Abbassi, E. Roayaei and M. A. Emadi, Emulsion flooding for enhanced oil recovery: interactive optimization of phase behavior, microvisual and core-flood experiments, J. Ind. Eng. Chem., 2015, 29, 382–391, DOI:10.1016/j.jiec.2015.04.019.
- T. Maozhang, S. Xinmin, M. Desheng, W. Qiang, Z. Qun, W. Luo, Z. H. Zhou and C. Zhao, Phase transitions in emulsions formed by aqueous emulsifier and its action on improving mobility in oil recovery, J. Dispersion Sci. Technol., 2016, 37(5), 706–714, DOI:10.1080/01932691.2015.1057836.
- H. Pei, J. Shan, X. Cao, G. Zhang and P. Jiang, Research Progresses on Nanoparticle-stabilized Emulsions for Enhanced Oil recovery, Mater. Rep., 2021, 35(13), 13227–13231, DOI:10.11896/cldb.20050023.
- L. Shi, X. Duan, J. Hou, T. Cheng, H. Yu and H. Xu, Thermal Stability and Salt Resistant Emulsion Flooding System, J. of Petrochem. Univ., 2014, 27(02), 59–64, DOI:10.3969/j.issn.1006-396X.2014.02.013.
- B. Li, C. Feng, F. Yang, G. Cu and L. Han, Influencing factors of crude oil emulsion stability, Chem. Eng., 2013, 27(11), 41–43, DOI:10.16247/j.cnki.23-1171/tq.2013.11.016.
- K. Wang, P. Liu, B. Wang, C. Wang, P. Liu, J. Zhao, J. Chen and J. Zhang, Experimental Study and Numerical Simulation of W/O Emulsion in Developing Heavy Oil Reservoirs, Appl. Sci., 2022, 12(22), 11867, DOI:10.3390/app122211867.
- Y. Zhou, D. Yin, W. Chen, B. Liu and X. Zhang, A comprehensive review of emulsion and its field application for enhanced oil recovery, Energy Sci. Eng., 2019, 7(4), 1046–1058, DOI:10.1002/ese3.354.
- B. P. Binks, J. A. Rodrigues and W. J. Frith, Synergistic interaction in emulsions stabilized by a mixture of silica nanoparticles and cationic surfactant, Langmuir, 2007, 23(7), 3626–3636, DOI:10.1021/la0634600.
- M. Xu, J. Jiang, X. Pei, B. Song, Z. Cui and B. P. Binks, Novel Oil-in-Water Emulsions Stabilised by Ionic Surfactant and Similarly Charged Nanoparticles at Very Low Concentrations, Angew. Chem., Int. Ed., 2018, 130(26), 7864–7868, DOI:10.1002/ange.201802266.
- K. Liu, J. Jiang, Z. Cui and B. P. Binks, pH-responsive Pickering emulsions stabilized by silica nanoparticles in combination with a conventional zwitterionic surfactant, Langmuir, 2017, 33(9), 2296–2305, DOI:10.1021/acs.langmuir.6b04459.
- Y. Zhu, J. Jiang, K. Liu, Z. Cui and B. P. Binks, Switchable Pickering emulsions stabilized by silica nanoparticles hydrophobized in situ with a conventional cationic surfactant, Langmuir, 2015, 31(11), 3301–3307, DOI:10.1021/acs.langmuir.5b00295.
- M. Lv, Q. Meng, W. Si, M. Hao, R. Han, Y. Lai, J. Jiang and Z. Cui, CO2-switchable oil-in-dispersion emulsions stabilized by tertiary amine surfactant and alumina particles, Colloids Surf., A, 2022, 641, 128541, DOI:10.1016/j.colsurfa.2022.128541.
- M. Zhou, Z. Zhang, L. Hou, X. Nie, L. Zhou, J. Zhao and X. Guo, Synthesis and performance of a series of dual hydroxyl sulfobetaine surfactants, J. Dispersion Sci. Technol., 2018, 39(1), 116–121, DOI:10.1080/01932691.2017.1300908.
- F. Zhang, D. Ma, Q. Wang, Y. Zhu, and W. Luo, A novel hydroxylpropyl sulfobetaine surfactant for high temperature and high salinity reservoirs, International Petroleum Technology Conference, OnePetro, 2013, DOI:10.2523/IPTC-17022-MS.
- S. Guo, H. Wang, J. Shi, B. Pan and Y. Cheng, Synthesis and properties of a novel alkyl-hydroxyl-sulfobetaine zwitterionic surfactant for enhanced oil recovery, J. Pet. Explor. Prod. Technol., 2015, 5, 321–326, DOI:10.1007/s13202-014-0141-y.
- C. Shi, S. Song, H. Ren, Q. Zen and X. Tang, Synthesis of didodecylmethyl hydroxyl sulfobetaine and its evaluation for alkali-free flooding, J. Pet. Explor. Prod. Technol., 2015, 5(2), 219–224, DOI:10.1007/s13202-014-0148-4.
- L. Shi, Synthesis and Properties of a Novel of Amphoteric Surfactants Sulfobetaines. Master thesis, Northeast Petroleum University, China, 2012 Search PubMed.
- Y. Fang and Y. Xia, Amphoteric Surfactants (I) Introduction to Amphoteric Surfactants, China Surfactant Deterg. Cosmet., 2000,(03), 53–55, DOI:10.13218/j.cnki.csdc.2000.03.017.
- Y. Fang and Y. Xia, Amphoteric Surfactants (II) Surface Activities and Colloidal Properties of Amphoteric Surfactants, China Surfactant Deterg. Cosmet., 2000,(04), 50–53, DOI:10.13218/j.cnki.csdc.2000.04.017.
- M. S. Baptista and M. J. Politi, Dipole oriented anion binding and exchange in zwitterionic micelles, J. Phys. Chem., 1991, 95(15), 5936–5942, DOI:10.1021/j100168a042.
- I. M. Cuccovia, L. S. Romsted and H. Chaimovich, Determination of halide concentrations at the interface of zwitterionic micelles by chemical trapping: influence of the orientation of the dipole and the nature of the cation, J. Colloid Interface Sci., 1999, 220(1), 96–102, DOI:10.1006/jcis.1999.6511.
- E. K. Perttu and F. C. Szoka, Zwitterionic sulfobetaine lipids that form vesicles with salt-dependent thermotropic properties, Chem. Commun., 2011, 47(47), 12613–12615, 10.1039/C1CC15804J.
- X. Ji, M. Tian, D. Ma, Y. Zhu, Z. H. Zhou, Q. Zhang and Y. Wang, Effects of calcium ions on the solubility and rheological behavior of a C22-tailed hydroxyl sulfobetaine surfactant in aqueous solution, Langmuir, 2018, 34(1), 291–301, DOI:10.1021/acs.langmuir.7b03614.
- S. Lu, D. Yang, M. Wang, M. Yan, Y. Qian, D. Zheng and X. Qiu, Pickering emulsions synergistic-stabilized by amphoteric lignin and SiO2 nanoparticles: stability and pH-responsive mechanism, Colloids Surf., A, 2020, 585, 124158, DOI:10.1016/j.colsurfa.2019.124158.
- K. Liu, Q. Lin, Z. Cui, X. Pei and J. Jiang, pH-Responsive Pickering Emulsions Stabilized by Silica Nanoparticles in Combination with N-Dodecyl-β-aminopropionate, Chem. J. Chin. Univ., 2017, 38(01), 85–93, DOI:10.1021/acs.langmuir.6b04459.
- X. Shi, X. Xing, Y. Lei, Y. Zhang and X. Ge, Research Progress on the Stability of Pickering Emulsion, Spec. Petrochem., 2020, 37(01), 65–71, DOI:10.3969/j.issn.1003-9384.2020.01.014.
- H. Chen, X. Zhang, Z. Xu and J. Zhao, Study on the Preparation Conditions of Olive Oil in Water Pickering Emulsions Stabilized by Attapulgite Particles and Formation Mechanism, Non-Met. Mines, 2013, 36(03), 13–17, DOI:10.3969/j.issn.1000-8098.2013.03.007.
- L. Yu, S. Li, L. P. Stubbs and H. C. Lau, Characterization of clay-stabilized, oil-in-water Pickering emulsion for potential conformance control in high-salinity, high-temperature reservoirs, Appl. Clay Sci., 2021, 213, 106246, DOI:10.1016/j.clay.2021.106246.
- X. Gong, Y. Wu and X. Li, Analysis of factors affecting stability of water-in-oil heavy oil emulsion, Appl. Chem. Ind., 2023, 52(04), 1218–1224, DOI:10.16581/j.cnki.issn1671-3206.20230217.014.
- W. Pu, M. He, X. Yang, J. Xie, Y. Chu and R. Liu, Selection of emulsification system for high temperature and high salt reservoir and evaluation of reservoir adaptability, J. Dispersion Sci. Technol., 2021, 1–10, DOI:10.1080/01932691.2021.1985514.
- F. Goodarzi and S. Zendehboudi, A comprehensive review on emulsions and emulsion stability in chemical and energy industries, Can. J. Chem. Eng., 2019, 97(1), 281–309, DOI:10.1002/cjce.23336.
- Q. Lan, F. Yang, S. Zhang, S. Liu, J. Xu and D. Sun, Synergistic effect of silica nanoparticle and cetyltrimethyl ammonium bromide on the stabilization of O/W emulsions, Colloids Surf., A, 2007, 302(1–3), 126–135, DOI:10.1016/j.colsurfa.2007.02.010.
- L. Xia, G. Cao and S. Lu, Influence of Inorganic Salts on Demulsification of Emulsions, Pet. Process. Sect., 2003, 19(04), 94–97 CAS.
- H. Jia, W. Huang, Y. Han, Q. Wang, S. Wang, J. Dai, Z. Tian, D. Wang, H. Yan and K. Lv, Systematic investigation on the interaction between SiO2 nanoparticles with different surface affinity and various surfactants, J. Mol. Liq., 2020, 304, 112777, DOI:10.1016/j.molliq.2020.112777.
- A. Pandey, H. Kesarwani, C. Tewari, A. Saxena, S. Sharma and N. G. Sahoo, Waste plastic derived reduced graphene oxide as a potential additive for the surfactant polymer flooding: A sustainable solution, J. Environ. Chem. Eng., 2023, 11(3), 109661 CrossRef CAS.
- S. Vats, F. Khan, D. Prajapati, A. Pandey, S. Sharma and A. Saxena, Synthesis and Evaluation of Mesoporous Silica Nanoparticle and its Application in Chemical Enhanced oil Recovery, ChemistrySelect, 2023, 8(12), e202204206 CrossRef CAS.
- K. Liu, A study on self-assembly of silica nanoparticles at fluid interface and its pH-responsiveness, Master thesis, Jiangnan University, China, 2017 Search PubMed.
- Q. Wang, Emulsification mechanism and emulsion stability related with nano-silica, Master thesis, Jiangnan University, China, 2021 Search PubMed.
- M. Xu, L. Xu, Q. Lin, X. Pei, J. Jiang, H. Zhu, Z. Cui and B. P. Binks, Switchable oil-in-water emulsions stabilized by like-charged surfactants and particles at very low concentrations, Langmuir, 2019, 35(11), 4058–4067, DOI:10.1021/acs.langmuir.8b04159.
|
This journal is © The Royal Society of Chemistry 2023 |
Click here to see how this site uses Cookies. View our privacy policy here.