DOI:
10.1039/D3RA03624C
(Paper)
RSC Adv., 2023,
13, 26324-26329
A novel tetranuclear Cu(II) complex for DNA-binding and in vitro anticancer activity†
Received
30th May 2023
, Accepted 29th August 2023
First published on 4th September 2023
Abstract
A novel tetranuclear Cu(II) complex (TNC) was successfully synthesized and characterized by X-ray single crystal diffraction. The interaction of the complex with calf thymus DNA (CT-DNA) has been studied by UV-vis absorption titration, fluorescence technology and molecular docking. The results indicated that TNC could bind to the DNA through an intercalative mode. The agarose gel electrophoresis experiment showed that TNC could cleave supercoiled plasmid DNA into linear DNA. The anticancer activity of TNC was tested on four cancer cell lines: MCF7, A549, 4T1 and HepG2. The results indicated that TNC shown significant activity against all of above cell lines.
Introduction
In the last decades, metal complexes have attracted increasing attention due to the potential applications such as catalysis,1–3 luminescence,4–8 magnetic material,9–13 and biological activities14,15 etc. In particularly, the anticancer activity of the metal complexes has received more attention since cisplatin was successfully used to treat various cancers.16,17
Copper is an essential trace element in the human body and plays a critical role in various biological processes. Compared to platinum-based drugs, copper compounds generally exhibit lower systemic toxicity.18 As a result, copper(II) complexes have garnered significant attention as potential anticancer drugs.19–21 Additionally, Cu2+ ions can be reduced to Cu+ ions by glutathione (GSH) overexpressed in the tumor microenvironment. Cu+ ions can then convert hydrogen peroxide (H2O2) overexpressed in the tumor microenvironment into highly toxic reactive oxygen species (ROS) known as hydroxyl radicals (˙OH), thereby killing tumor cells.22–24 This process is known as chemodynamic therapy (CDT). Currently, most of the reagents used for CDT are nanoparticles of metal oxides,25–27 but their application in vivo is limited due to safety concerns. On the other hand, oxamide-based ligands such as N,N′-bis(substituted) oxamide have flourished indeed since they can be used to construct complexes with structural diversity.28–31 In addition to the utilization of an oxamide-based ligand, the incorporation of 1,10-phennanthroline as a secondary ligand was deemed necessary due to the fact that 1,10-phennanthroline derivatives have demonstrated noteworthy biological activity, including but not limited to antibacterial, antifungal, and antiviral properties.32 These complexes have shown promising results in improving the antitumor activity.
Molecular docking is a computer simulation method for drug design through the interaction between receptors and drug molecules. This method is mainly used to study intermolecular interactions (such as ligands and receptors) and predict their binding modes and affinity. In recent years, molecular docking has become an important technology in the field of computer-aided drug research. Many studies on the interaction between complexes and DNA have used molecular docking to predict the interaction mode.33–36
Taking all this into account, in the present study the synthesis, structure, characterization and DNA binding of a tetranuclear copper(II) complex (TNC) were investigated in detail. Molecular docking was also investigated to predict the binding mode. Agarose gel experiments were carried out to further study the ability of TNC to cleave supercoiled plasmid DNA. Moreover, the in vitro anticancer potential of TNC was also evaluated against MCF7, A549, 4T1 and HepG2 cell lines (Scheme 1).
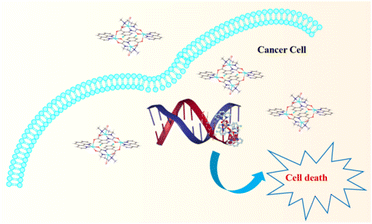 |
| Scheme 1 Schematic illustration of TNC antitumor activity. | |
Results and discussion
Synthesis route
The synthetic routes for TNC are shown in Scheme 1S.† The mononuclear copper ligand (CuL) was synthesized from the reaction of 2-amino-5-fluorobenzoic acid with ethyl chlorooxoacetate in tetrahydrofuran medium to get the intermediate ethyl-N-2-(carboxy-4-fluorophenyl)oxamate. Then the intermediate reacted with 3-(dimethylamino)-1-propylamine in tetrahydrofuran medium to get H3L. The CuL was prepared by the reaction of the H3L with cupric chloride and sodium hydroxide in ethanol and water in 1
:
1 (v/v) ratio. TNC was synthesized by the reaction of CuL and cupric bromide with 1,10-phenanthroline in methanol.
Crystal structure
The triclinic space group P
is responsible for crystal diffraction parameters of TNC. The complex comprises four Cu(II) centers, two Br− anions, two L ligands and two 1,10-phennanthroline ligands. As the structure of TNC is symmetric, only the symmetric unit is discussed. Fig. 1 shows that the coordination environment around the copper atoms is distinct. The Cu1 atom has an N3OBr donor set and adopts a distorted square pyramidal geometry (τ = 0.04) with bond angles in the range of 83.3(2) to 164.8(2)°. τ is defined as, the difference between the maximum and second maximum angles surrounding the central Cu(II) ion, divided by 60. The range of τ is from zero to 1.00, where zero corresponds to a perfect square pyramid and 1.00 is for an ideal trigonal bipyramid. The basal planes of the square pyramids are formed by the O1, N1, N2, and N3 atoms of the oxamide ligands. The range of bond lengths is from 1.936(4) to 2.048(6) Å and bond angle is from 83.3(2) to 164.8(2)° in the square pyramids. The Cu1 ion deviates 0.278 Å from the basal plane toward the apical bromine anion which is located in the axial position with a bond length of 2.7782(12) Å. Due to the Jahn-Teller elongation effect, the bond length is greater than the basal one.37 The Cu2 atoms are in an N2O3 coordination environment, forming a distorted square pyramidal geometry (τ = 0.235). The basal plane is the square pyramid which is defined by O3, O4 from the oxamide ligand and N4, N5 from the 1,10-phennanthroline ligand. The bond angle range is from 83.0(2) to 174.6(2)° and the bond length range is from 1.928(4) to 1.986(5) Å in the basal plane. The Cu2 ion is located above the basal plane toward the apical O21 atom with a distance about 0.211 Å. The oxygen atom (O21; 1 − X, 2 − Y, 1 − Z) lies in the axial position. The structure was stabilized by hydrogen bonds and π–π* stacking interactions. Free water forms intermolecular hydrogen bonds with the oxamide ligand. 3D supramolecular network of TNC was shown in Fig. 1S.†
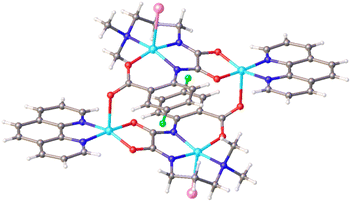 |
| Fig. 1 Crystal structure of TNC. C = gray, N = blue, O = red, Cu = sky blue, Br = pink, F = green, solvent molecules are omitted for clarity. | |
Stability of TNC. It is generally known that the stability of the complex in aqueous media affects its uptake by cells. To investigate the stability of TNC in aqueous media, UV-vis spectroscopy experiments were carried out. The results of the absorption spectra were shown in Fig. 2S.† As shown in Fig. 2S,† after standing for 24 hours, the absorbance of TNC aqueous solution decreased by 5.4%. According to our previous work,38 complexes could enter the cell within 12 hours, so the stability could meet the requirements of cell uptake.
DNA interaction studies.
Electronic absorption. To further investigate the interaction between TNC and DNA, UV-vis spectroscopy experiments were carried out. It is well known that complexes can bind to DNA through noncovalent interactions which include intercalative, electrostatic and groove modes. Generally, when the complexes bind to DNA through the intercalative mode, it could result in the strong stacking interaction between the aromatic chromophore of the complex and the base pairs of DNA.39 The groove mode is interaction between the complex and the DNA bases by hydrogen bond and van der Waals force. The electrostatic mode is the interaction between the two through the Coulomb force.36 The absorption spectra was shown in Fig. 2(a). It was observed from Fig. 2(a), as the CT-DNA added in the solution of TNC, the absorption bands of TNC showed hypochromisms about 28.6% and 16.6% without red shifts at 273 nm and 290 nm, respectively. These results clearly showed that TNC most likely bound to CT-DNA by intercalative mode.40,41 To further study the binding strength between TNC and CT-DNA, Kb was calculated using following equation: |
CDNA/(εa − εf) = CDNA/(εb − εf) + 1/[Kb(εb − εf)]
| (1) |
where CDNA is the CT-DNA concentration, εa, εb and εf are the extinction coefficient of the partially bound TNC, fully bound TNC and free TNC, respectively. The Kb was calculated from the ratio of slope to the intercept from CDNA/(εa − εf) versus CDNA plot.42 As shown in Fig. 2(b), Kb of TNC was calculated to be 7.22 × 103 L M−1 (R2 = 0.99008) suggesting TNC binding to CT-DNA through an intercalative mode.
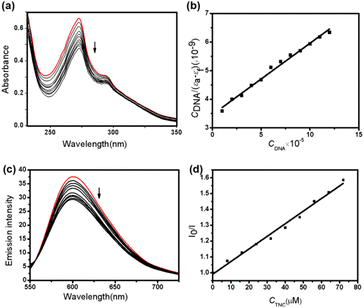 |
| Fig. 2 (a) The absorb spectra for TNC binding with DNA. Arrow shows the absorbance change upon the increase of the TNC concentrations. (b) The linear DNA-binding constant Kb (λ = 273 nm.). (c) Emission spectra for the EB–DNA system. Arrow shows the intensity change upon the increase of the TNC concentrations. (d) The linear Stern–Volmer constant Ksv. | |
Fluorescence spectral studies. Next, fluorescence spectral were carried out to further determinate the binding mode. EB (ethidium bromide) is a kind of nucleic acid intercalating agent and its fluorescence intensity is very weak. Once binding with DNA, the fluorescence intensity of EB will be greatly improved. If the complex replaced EB and intercalated with DNA base, the fluorescence intensity of EB will be weakened. The emission spectra of the EB–DNA was shown in Fig. 2(c). It was observed from Fig. 2(c) that emission quenching at 600 nm due to TNC addition to the EB–DNA mixture, which became more obvious with increasing TNC concentration, suggesting that TNC replace the EB molecules from the DNA binding sites. The results for the quenching of EB–DNA by TNC was in agreement with the following Stern–Volmer equation:43where I0 and I are the fluorescence intensities of the EB–DNA solution and EB–DNA–TNC system, respectively. Ksv is quenching constant, CTNC is the TNC concentration. The quenching plot (see Fig. 2(d)) was obtained by plotting I0/I versus CTNC. In the Fig. 2(d), the Ksv was got from the ratio of the slope to intercept which was calculated to be 8.1 × 103 L M−1 for TNC. This results are consistent with the above UV-vis experiments.
Molecular docking. The binding mode of the lowest free energy pose of TNC and DNA are shown in Fig. 3. As depicted from Fig. 3, the π–π* stacking between phenanthroline ligand of TNC and benzene ring of DC9 residue in DNA double helix and the hydrophobic interaction between TNC and DT8 residue of DNA made the TNC stably bind to DNA through the intercalative mode. The results of molecular docking were consistent with the experimental results of DNA binding.
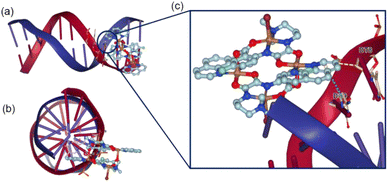 |
| Fig. 3 Binding pose for TNC and CT-DNA. (a) X-axis view (b) Z-axis view (c) binding site. The blue dotted line indicated π–π* stacking and yellow dotted line indicates hydrophobic interaction. | |
DNA cleavage. In order to further investigate the cleavage ability of TNC on plasmid pUC19 DNA (which is a supercoiled DNA), the agarose gel electrophoresis experiment was performed. As shown in Fig. 3S,† with the increase of TNC concentration, the number of supercoiled DNA (Form I) decreased and the amount of linear DNA (Form II) increased, indicating that TNC could cleave supercoiled DNA into linear DNA. These results indicated that excellent DNA cleavage ability would contribute to cytotoxicity against cancer cells.
In vitro anticancer activity. The positive results received from TNC binding to DNA and DNA cleavage inspired us to evaluate its anticancer activity against cancer cell lines MCF7, A549, 4T1 and HepG2 by the MTT assay method. As shown in Fig. 4, the cell viability was found to be about 104% for MCF7, 94% for A549, 84% for 4T1 and 90% for HepG2 at 0.32 μM dose of TNC. When TNC concentration reached to 0.63 μM, the cell viability decreased slightly for all four cell lines. At a dose of 1.25 μM, the cell viability continues to decrease for MCF7 (71%), for A549 (76%), for 4T1 (52%) and for HepG2 (61%). When the dose increased to 5 μM, the cell viability was found to decreased significantly for MCF7 (5%), for A549 (6%), for 4T1 (18%) and for HepG2 (9%). Fig. 4 indicated that TNC shown significant activity against MCF7, A549, 4T1 and HepG2 cell lines. The highly cytotoxic activity of TNC may be duo to TNC binding to DNA. The results of the in vitro anticancer activities further confirmed the interaction between TNC and CT-DNA, which consequently resulted in cell death.
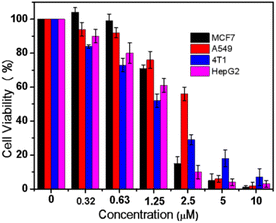 |
| Fig. 4 Cell viability of MCF7, A549, 4T1 and HepG2 cells subjected to TNC. | |
Experimental
Materials
Calf thymus DNA (CT-DNA) and 2-amino-5-fluorobenzoic acid (98%) were bought from Alfa Aesar. Ethyl chlorooxoacetate (98%) and 3-(dimethylamino)-1-propylamine (98%) were purchased from Aladdin, shanghai. MCF-7, A549, 4T1 and HepG2 cells were purchased from Chinese Academy of Sciences Cell Bank. All other chemicals were commercially available and used directly.
Synthesis of the TNC
Synthesis of the ligand (H3L) and mononuclear copper (CuL). The synthetic routes for H3L and CuL are shown in Scheme 1S.† The synthesis method of H3L and CuL refers to our previous work.22
Synthesis of TNC. The solution of cupric bromide (0.0223 g, 0.1 mmol) in methanol (5 mL) was added into the solution of CuL (0.0395 g, 0.1 mmol) in methanol solution (10 mL) and the reaction mixture was continued to be stirred for 0.5 h, then a methanol solution (5 mL) of 1,10-phenanthroline (0.01982 g, 0.1 mmol) was added into the mixture and continued to be stirred for 5 h at 60 °C. The precipitate in the reaction mixture was removed to obtain a green solution, which was slowly evaporated at 20 °C, and green crystals were obtained after 7 days. Yield: 78%; Anal. calcd for C27H29BrCu2FN5O6 (%): C, 43.50; H, 3.92; N, 9.39. Found (%): C, 43.48; H, 3.90; N, 9.41; IR (KBr pellet, ν/cm−1): 1627 [νas (COO) + ν(C
O)]; 1446 [ν (C
N)]; UV-vis: λmax (nm) [εmax (M−1 cm−1)]: 273(66204); 290(31314).
Crystal structure determination and refinement. The X-ray diffraction technology was used to determinate the crystal structure. Single crystal diffraction experiment was executed on Apex Smart CCD diffractometer (Bruker) with Mo-Kα radiation (λ = 0.71073 Å) at 298 K. The structure of TNC was solved and refined using software SHELXS-97 by the direct method. The refinement method is full-matrix least squares based on F2. Crystal and refinement data are listed in Table S1.† The bond angles and selected bond lengths are summarized in Table 1. CCDC number for TNC is 1888702.†
Table 1 Selected bond length and bond angle for TNCa
Bond |
Dist. |
Bond |
Dist. |
1 − X, 2 − Y, 1 − Z. |
Cu1–O1 |
1.936(4) |
Cu2–O4 |
1.928(4) |
Cu1–N2 |
1.972(5) |
Cu2–O3 |
1.950(4) |
Cu1–N1 |
1.992(5) |
Cu2–N4 |
1.986(5) |
Cu1–N3 |
2.048(6) |
Cu2–N5 |
1.986(5) |
Cu1–Br1 |
2.7782(12) |
Cu2–O21 |
2.244(5) |
Angle |
(°) |
Angle |
(°) |
O1–Cu1–N2 |
162.4(2) |
O4–Cu2–O3 |
85.13(17) |
O1–Cu1–N1 |
90.03(18) |
O4–Cu2–N4 |
94.7(2) |
N2–Cu1–N1 |
83.3(2) |
O3–Cu2–N4 |
174.6(2) |
O1–Cu1–N3 |
87.0(2) |
O4–Cu2–N5 |
160.5(2) |
N2–Cu1–N3 |
95.1(2) |
O3–Cu2–N5 |
95.43(19) |
N1–Cu1–N3 |
164.8(2) |
N4–Cu2–N5 |
83.0(2) |
O1–Cu1–Br1 |
101.64(15) |
O4–Cu2–O21 |
106.01(18) |
N2–Cu1–Br1 |
95.51(18) |
O3–Cu2–O21 |
92.06(16) |
N1–Cu1–Br1 |
99.65(16) |
N4–Cu2–O21 |
93.17(18) |
N3–Cu1–Br1 |
95.58(19) |
N5–Cu2–O21 |
93.50(18) |
Stability of TNC
Dissolved a certain amount of TNC in DMSO to obtain 10 mM stock solution. Took 20 μL stock solution and diluted it with water. The absorbance of the diluted solution was measured using a ultraviolet visible (UV-vis) spectrophotometer from 240–400 nm.
DNA interaction studies
UV-vis absorption studies. To determinate the mode of action between DNA and TNC, UV-vis absorption experiments were carried out. During the experiment, the concentration of TNC was kept constant at 10 μM in tris–HCl/NaCl buffer with pH = 7.2 (5 mM tris–HCl/50 mM NaCl), while the CT-DNA was added in batches. The solutions of TNC and CT-DNA were incubated together for 4–5 min before testing.
Fluorescence quenching study. To further determinate the mode of action between TNC and CT-DNA, the ethidium bromide (EB) fluorescence quenching experiments were performed. In these experiments, stock solution of the EB and CT-DNA system with [EB] = 1 μM and [CT-DNA] = 8.8 × 10−4 M in Tris buffer was incubated for 4 hours in the dark before using. TNC was added into the system and incubated for 10 min at 20 °C before measurements. Fluorescence intensities of the EB and CT-DNA system were recorded from 530 nm to 700 nm with the excitation 520 nm.
Molecular docking. As a computer simulation, molecular docking is considered to be the most reliable tool to predict the binding mode of DNA and complexes. Use the crystal data of TNC to draw a 3D molecular structure, and then the 3D molecular structure was hydrogenated, charged, and minimized in the MMFF94 force field. The crystal structure of CT-DNA (PDB no. 1bna) was downloaded from the ProteinData Bank and used for molecular docking after the ligand water in the crystal structure was removed. GHECOM algorithm was used to identify DNA binding sites and box size. The varying poses were calculated by using flexible molecular docking mode. The lowest free energy pose was selected to analyze the interaction between DNA and TNC.
DNA cleavage. Supercoiled DNA (pUC19) was purchased from thermo scientific. 20 ng mL−1 supercoiled pUC19 DNA was incubated with the different concentration TNC for 12 h. Then loading buffer was added into above mixture. Gel electrophoresis experiment was carried out with 1.5% (tris–acetate EDTA (TAE) buffer) agarose gel at 150 V 30 min in TAE buffer. The agarose gel was stained 20 min with Ethidium bromide (1 μg mL−1), then analyzed with the ChemiScope 6200 gel imaging system.
In vitro cytotoxicity assay. To assess the cytotoxicity of TNC, cell viability experiments were performed on breast adenocarcinoma (MCF7), hepatocellular carcinoma (HepG2), human small cell lung cancer (A549) and mouse breast cancer (4T1) cells lines. In this assay, MCF7, A549, HepG2 and 4T1 cells were seeded with the density of 5000 cells per well in 96-well plate and cultured for 24 h in a 5% CO2 incubator at 37 °C. Next, the old medium was discarded and then the fresh medium containing different concentrations of TNC was added to each well. After 48 h, 20 μL 3-(4,5-dimethylthiazol-2-yl)-2,5-diphenyltetrazolium bromide (MTT) (5 mg mL−1) were added in each well and continued to culture for another 4 h. During this period, the alive cell could reduce the yellow MTT to the purple formazan crystals under the action of mitochondrial dehydrogenase. Then the medium was discarded carefully and 200 μL dimethyl sulfoxide (DMSO) was added into each well to dissolve the purple formazan crystals. The absorbance of each well was measured at 490 nm on a Molecular Devices SpectraMax i3x plate reader. The MTT assays were performed in triplicates.
Conclusions
A novel tetranuclear Cu(II) complex (TNC) bridged by N-(2-carboxy-4-fluorophenyl) – N′-[3-(dimethylamino)propyl]oxamide had been successfully synthesized and well characterized. The interaction of TNC with CT-DNA had been studied using UV-vis absorption, fluorescence technology and molecular docking. The results revealed that TNC could bind to CT-DNA through the intercalative mode. Agarose gel experiment was carried out to further study the ability of TNC to cleave supercoiled plasmid DNA. The results showed that TNC could cleave supercoiled plasmid DNA into linear DNA. In addition, TNC showed excellent cytotoxic activity against MCF7, A549, 4T1 and HepG2 cancer cell lines. The results clearly illustrated that TNC have potential practical applications.
Conflicts of interest
There are no conflicts to declare.
Acknowledgements
We thank Guangzhou Yinfo Information Technology Co., Ltd. for providing a friendly and versatile web server (https://cloud.yinfotek.com) to aid the molecular docking studies.
Notes and references
- S. Xia, L. Gan, K. Wang, Z. Li and D. Ma, J. Am. Chem. Soc., 2016, 138, 13493–13496 CrossRef CAS PubMed.
- P. Zhang, X. Hou, L. Liu, J. L. Mi and M. Dong, J. Phys. Chem. C, 2015, 119(50), 28028–28037 CrossRef CAS.
- Y. S. Kang, Y. Lu, K. Chen, Y. Zhao, P. Wang and W. Y. Sun, Coordin. Chem. Rev., 2019, 378, 262–280 CrossRef CAS.
- L. You, B. Zhao, H. Liu, S. Wang, G. Xiong, Y. He, F. Ding, J. J. Joos, P. F. Smet and Y. Sun, CrystEngComm, 2018, 20, 615–623 RSC.
- K. M. Ayers, N. D. Schley and G. Ung, Chem. Commun., 2019, 55(58), 8446–8449 RSC.
- A. B. Scharf, S. L. Zheng and T. A. Betley, Dalton Trans., 2021, 50(19), 6418–6422 RSC.
- E. E. Braker, N. F. M. Mukthar and N. D. Schley, ChemPhotoChem, 2021, 5(10), 902–905 CrossRef CAS.
- Y. Mi, M. Yang, X. Kuang and C. Lu, Chin. J. Struct. Chem., 2022, 41(2), 2202079–2202084 CAS.
- D. Shi, W. Wang, S. Wang and Z. Liu, J. Mol. Struct., 2021, 12229(5), 129807 CrossRef.
- H. W. Wei, Q. F. Yang, X. Y. Lai, X. Z. Wang, T. L. Yang, Q. Hou and X. Y. Liu, CrystEngComm, 2018, 20, 962–968 RSC.
- K. Dankhoff and B. Weber, CrystEngComm, 2018, 20, 818–828 RSC.
- D. Natke, A. Preiss, S. Klimke, T. Shiga, R. Boca, M. Ohba, H. Oshio and F. Renz, Eur. J. Inorg. Chem., 2021, 2021(15), 1498–1504 CrossRef CAS.
- B. Dojer, A. Golobič, N. Babič, Z. Jagličić and M. Kristl, J. Mol. Struct., 2022, 1265, 133393 CrossRef CAS.
- C. Schattschneider, S. D. Kettenmann, S. Hinojosa, J. Heinrich and N. Kulak, Coordin. Chem. Rev., 2019, 385, 191–207 CrossRef CAS.
- M. Sohrabi, M. Saeedi, B. Larijani and M. Mahdavi, Eur. J. Med. Chem., 2021, 216, 113308 CrossRef CAS PubMed.
- X. Tai, H. Guo and Q. Guo, Chin. J. Struct. Chem., 2018, 37(7), 1052–1056 CAS.
- S. Cao, X. Tai and C. Xin, Chin. J. Struct. Chem., 2021, 40(3), 324–328 CAS.
- D. C. Shobha, B. Thulasiram, A. R. Rao and N. Penumaka, J. Fluoresc., 2018, 28, 1195–1205 CrossRef PubMed.
- S. H. S. Saleem, M. Sankarganesh, J. D. Raja, P. R. A. Jose, A. Sakthivel, T. C. Jeyakumar and R. N. Asha, J. Saudi Chem. Soc., 2021, 25, 101225 CrossRef CAS.
- P. Jain, C. Das, S. Roychoudhury, H. Majumder and S. Das, ChemistrySelect, 2016, 1(21), 6623–6631 CrossRef.
- S. Abdelrahman, M. Alghrably, M. Campagna, C. A. E. Hauser, M. Jaremko and J. I. Lachowicz, Molecules, 2021, 26, 4730 CrossRef CAS PubMed.
- S. Cao, X. Li, Y. Gao, F. Li, K. Li, X. Cao, Y. Dai, L. Mao, S. Wang and X. Tai, Dalton Trans., 2020, 49, 11851–11858 RSC.
- S. Cao, F. Li, Q. Xu, M. Yao, S. Wang, Y. Zhou, X. Cui, R. Man, K. Li and X. Tai, J. Saudi Chem. Soc., 2021, 25, 101372 CrossRef CAS.
- B. Ma, S. Wang, F. Liu, S. Zhang, J. Duan, Z. Li, Y. Kong, Y. Sang, H. Liu, W. Bu and L. Li, J. Am. Chem. Soc., 2019, 141, 849–857 CrossRef CAS PubMed.
- S. Wang, L. Yang, H. Cho, S. Chueng and K. Lee, Biomaterials, 2019, 224, 119498 CrossRef CAS PubMed.
- P. Yang, J. Tao, F. Chen, Y. Chen, J. He, K. Shen, P. Zhao and Y. Li, Small, 2021, 11(7), 202005865 Search PubMed.
- N. Zhang, G. Shu, L. Shen, J. Ding, E. Qiao, S. Fang, J. Song, Y. Yang, Z. Zhao, C. Lu, J. Tu, M. Xu, Y. Du, M. Chen and J. Ji, Nano Res., 2022, 15(6), 5262–5272 CrossRef CAS.
- B. Ramakrishna, D. Divya, P. V. Monisha and B. Manimaran, Eur. J. Inorg. Chem., 2015, 36, 5839–5846 CrossRef.
- M. M. Omar, H. F. A. El-Halim and E. A. M. Khalil, Appl. Organomet. Chem., 2017, 31, e3724 CrossRef.
- S. Weheabby, M. A. Abdulmalic, M. Atzori, R. Sessoli, A. Aliabadi and T. Rüffer, Dalton Trans., 2018, 47(45), 16164–16181 RSC.
- S. P. Gavrish, Y. D. Lampeka, M. V. Babak and V. B. Arion, Inorg. Chem., 2018, 57(3), 1288–1297 CrossRef CAS PubMed.
- M. r Kuma, S. U. Parsekar, N. Duraipandy, M. S. Kiran and A. P. Koley, Inorg. Chim. Acta, 2019, 484, 219–226 CrossRef.
- N. Arshad, N. Abbas, F. Perveen, B. Mirza, A. M. Almuhaini and S. Alkahtani, J. Saudi Chem. Soc., 2021, 25, 101323 CrossRef CAS.
- S. A. Al-Harbi, J. Saudi Chem. Soc., 2022, 26, 101528 CrossRef CAS.
- A. Hossan, A. F. Alrefaei, H. A. Katouah, A. Bayazeed, B. H. Asghar, F. Shaaban and N. M. El-Metwaly, J. Saudi Chem. Soc., 2023, 27(2), 101599 CrossRef CAS.
- A. Ziannaa, G. D. Geromichalosa, A. Pekoub, A. G. Hatzidimitrioua, E. Coutouli-Argyropoulouc, M. Lalia-Kantouria, A. A. Pantazakib and G. Psomasa, J. Inorg. Biochem., 2019, 199, 110792 CrossRef PubMed.
- P. Sudipta, C. Koushik, G. Surajit, R. Kunal, J. Barnali and K. Saugata, J. Mol. Struct., 2018, 1152, 96–100 CrossRef.
- S. Cao, J. Fan, W. Sun, F. Li, K. Li, X. Tai and X. Peng, Chem. Commun., 2019, 55, 12956–12959 RSC.
- F. Shan, H. Song, X. Gao, B. Li and X. Ma, Chin. J. Struct. Chem., 2022, 41(2), 2202057–2202063 CAS.
- Y. Kou, M. Li and X. Ren, Spectrochim. Acta, Part A, 2018, 205, 435–441 CrossRef CAS PubMed.
- S. Radisavljević, L. Bratsos, A. Scheurer, J. Korzekwa, R. Masnikosa, A. Tot, N. Gligorijević, S. Radulović and A. R. Simović, Dalton Trans., 2018, 47, 13696 RSC.
- A. M. Pyle, J. P. Rehmann, R. Meshoyrer, C. V. Kumar, N. J. Turro and J. K. Barton, J. Am. Chem. Soc., 1989, 111, 3051–3058 CrossRef CAS.
- D. R. Mcmillan and K. M. Mcnett, Chem. Rev., 1998, 3, 1201–1220 CrossRef PubMed.
|
This journal is © The Royal Society of Chemistry 2023 |
Click here to see how this site uses Cookies. View our privacy policy here.