DOI:
10.1039/D3RA03874B
(Paper)
RSC Adv., 2023,
13, 20909-20915
Synthesis and biological evaluation of sulfamoyl benzamide derivatives as selective inhibitors for h-NTPDases†
Received
9th June 2023
, Accepted 4th July 2023
First published on 11th July 2023
Abstract
The aim of this research work is the synthesis of sulfamoyl-benzamides as a selective inhibitor for h-NTPDases. Sulfonamides are synthesized in aqueous medium from chlorosulfonylbenzoic acid while carboxamides are synthesized using carbodiimide coupling decorated with different biologically relevant substituents such as n-butyl, cyclopropyl, benzylamine, morpholine, and substituted anilines. In addition, sulfonamide-carboxamide derivatives were synthesized having the same substituents on either side. These compounds were screened against h-NTPDase activity, a main family of ectonucleotidases. Among the eight discovered isoforms of the h-NTPDases, four isoforms, h-NTPDase1, -2, -3, and -8, are involved in various physiological and pathological functions, for instance thrombosis, diabetes, inflammation, and cancer. The compound N-(4-bromophenyl)-4-chloro-3-(morpholine-4-carbonyl)benzenesulfonamide (3i) was found to be the most potent inhibitor of h-NTPDase1 with an IC50 value of 2.88 ± 0.13 μM. Similarly, the compounds N-(4-methoxyphenyl)-3-(morpholinosulfonyl)benzamide (3f), 5-(N-benzylsulfamoyl)-2-chloro-N-(4-methoxyphenyl)benzamide (3j) and 2-chloro-N-cyclopropyl-5-(N-cyclopropylsulfamoyl)benzamide (4d) reduced the activity of the h-NTPDases2 with IC50 in sub-micromolar concentrations. Against the h-NTPDase3, 3i was the potent compound with an IC50 concentration of 0.72 ± 0.11 μM. The h-NTPDase8 was selectively blocked by the most potent inhibitor 2-chloro-5-(N-cyclopropylsulfamoyl)benzoic acid (2d) with (IC50 = 0.28 ± 0.07 μM). Moreover, the molecular docking studies of the potent inhibitors showed significant interactions with the amino acids of the respective h-NTPDase homology model proteins.
Introduction
The ectonucleotidases are comprised of four families which include nucleoside triphosphate diphosphohydrolases (h-NTPDases), ecto-nucleotide pyrophosphatases/phosphodiesterases (NPPs), ecto-5′-nucleotidase and alkaline phosphatases (ALPs)1–6 The h-NTPDases belong to a diverse group of eight isozymes ranging from h-NTPDase1 to h-NTPDase8. Each isozyme has a specific capability to catalyse nucleotides. The h-NTPDase1, h-NTPDase3, and h-NTPDase8 have the ability of hydrolysing nucleoside triphosphates as well as nucleoside diphosphates with nearly similar preferences whereas, h-NTPDase2 preferentially catalyses the nucleoside triphosphate as substrate.7 The expression of the h-NTPDase isoforms was identified in almost every human body tissue i.e. h-NTPDase1 is expressed in the subsets of the activated T-cells, monocytes, blood vascular endothelium, and dendritic cells.4 h-NTPDase3 was found in the digestive system (epithelial cells), kidneys, reproductive system, respiratory tract, and islets of Langerhans, whereas, h-NTPDase8 was located in the kidney, liver, and intestine.8–11 Various physiological and pathological functions are associated with the h-NTPDases such as regulation of vascular fluidity, cardiac protection, inflammation of vascular vessels, maintenance of thrombosis, and relaxation of vascular vessels. Some unwanted effects due to the irregularity of the h-NTPDases include thrombosis, inflammation, diabetes, and cancer.8,12,13
Sulfonamides are the most effective chemotherapeutic agents used as anti-hypertensive, anti-bacterial, antiprotozoal, anti-cancer, anti-inflammatory, and anti-diuretic agents.14 They are mostly considered bacteriostatic and exhibit exceptional anti-bacterial activity against both Gram-positive and Gram-negative bacteria.15,16 Hence, sulfonamide moieties are used to treat septicaemia, bacillary dysentery, tonsillitis, and urinary tract infections.17 They can also lower the blood glucose level and are employed as anti-diabetic agents.
Sulfamoyl-carboxamide is a continually growing class of derivatives possessing valuable and diverse biological activities. Some recent biological activities are reviewed to demonstrate their significance (Fig. 1). NLRP3 is an emerging target for inflammatory diseases such as neuroinflammation, immune inflammation, and metabolic inflammation. NLRP3 is a nucleotide oligomerization domain (NOD)-like receptor protein 3 which is activated by pathogens and damages the cell signal pathway by making NLRP3 inflammasome, a multimeric protein complex.18 Small molecule sulfonylurea urea such as glyburide (glibenclamide) have been identified as inhibitors for NLRP3 inflammasome.19 Further modification of the glyburide led to sulfamoyl-benzamide derivatives JC-171 with higher selectivity, increase solubility, and importantly, without affecting the glucose level (Fig. 1).20 Mincione et al. have reported sulfamoyl-benzamides as topically acting carbonic anhydrase inhibitors. These were tested against three isozymes of carbonic anhydrases and showed affinity close to clinical drugs both in vitro and in vivo as evidenced by compound I (Fig. 1).21 A series of sulfamoyl-4-oxoquinoline-3-carboxamides were screened for defective ΔF508-cystic fibrosis transmembrane conductance regulator (CFTR) chloride channel. Several of the compounds showed correction for CFTR in sub-micromolar concentration, for instance compound II was more effective in comparison.22 A prominent example is the sulfonylurea-carboxamide based herbicide nicosulfuron, which works at low doses and high selectivity for corn.23 Zhao et al. have reported a series of indole sulfonamide-carboxamide as an excellent inhibitor for wild-type HIV reverse transcriptase through a systematic SAR. The optimization led to compound III which was found to have pyrrolidine sulfonamide at position 3 and bromo at position 5 of the indole structure.24 The 5-chlorosalicylamide scaffold holds biologically interesting properties and the core structure exists in glyburide and JC-171 (vide supra), however, Galal et al. have found their anti-cancer properties. The sulfamoyl-salicylamide derivatives were tested against five types of human cell lines, while compound IV was found to be the best against the breast cancer (MCF-7 and MDA-MB-231) cells (Fig. 1). Furthermore, the tubulin polymerization experiments and flow cytometric assays revealed that compound IV resulted in cell cycle arrest by preventing tubulin polymerization.25
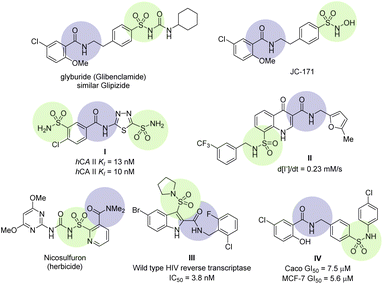 |
| Fig. 1 Selected examples of sulfamoyl-carboxamide containing biologically active compounds. | |
Considering our interest in finding new inhibitors for h-NTPDases,26,27 and owing to the diverse biological profile of sulfamoyl-carboxamide, synthesis of planned derivatives of sulfamoyl-benzamides was carried out to identify their potential against the activity of the h-NTPDase-1, -2, -3, and -8 to develop more effective inhibitors of h-NTPDases for the potential treatment of the pathological conditions associated with the unwanted function of the h-NTPDases.
Results and discussion
Chemistry
The synthesis of the sulfamoyl-benzamide derivatives is outlined in Scheme 1. We synthesize the 2-substituted benzene sulfonamides-carboxamides through a linear approach, starting from chlorosulfonation of benzoic acids, followed by the sulfonamide and finally the carboxamide synthesis. The chlorosulfonation of the electron-deficient benzoic acid has required slight excess of chlorosulfonic acid and elevated temperature.28 The corresponding sulfonyl chloride was treated with different amines including cyclopropylamine, morpholine and p-bromoaniline for the synthesis of sulfamoylbenzoic acids (2a–2e).
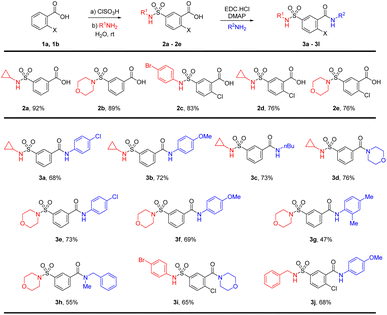 |
| Scheme 1 Linear synthesis of sulfamoyl-benzamide derivatives. | |
The sulfamoyl benzoic acids were subjected to standard carbodiimide coupling conditions using EDC catalytic DMAP in DCM and DMF as co-solvents.29 The amide coupling generally worked well for anilines and primary and secondary aliphatic amines (Scheme 1). The cyclopropyl sulfonamide 2a was treated with p-chloroaniline and p-anisidine for the synthesis of the sulfamoyl-benzamides 3a and 3b in 68% and 72% isolated yields, respectively. There was little effect of the electronic nature of the aniline on the amide formation. Similar yields were found for aliphatic amines such as n-butylamine and morpholine resulting in product 3c and 3d. The morpholinosulfonamide 2b was also reacted with p-chloroaniline and p-anisidine for the synthesis of the benzamides 3e and 3f in 73% and 69% yields, respectively. In addition, 2,4-xylidine was able to furnish product 3g in 47% yield. Further reaction with N-methylbenzylamine resulted in the corresponding amide 3h in 55% yields. The morpholine substituted products 3e–3h have high polarity and if the pH is not properly maintained, the isolation of the product from the aqueous phase may pose a challenge. The p-bromoaniline and benzylamine substituted sulfonamides 2c and 2d were also converted to amides 3i and 3j in good yields (Scheme 1).
To introduce further diversity, a one-pot bis-sulfonamide-carboxamide synthesis resulted in parallel substitutions on either side of the aryl core. To this end, the chlorosulfonation product was telescoped and treated with excess amines for the synthesis of sulfonamide and carboxamide simultaneously for product 4a–4e in good yields. The bis-amidation was carried out with p-chloroaniline, morpholine, pyrrolidine, cyclopropylamine, and benzylamine (Scheme 2).
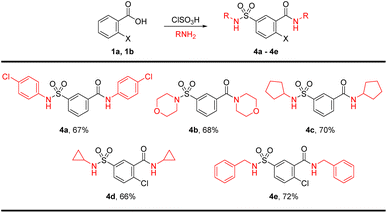 |
| Scheme 2 Direct synthesis of sulfamoyl-benzamide derivatives. | |
Biological study
Enzyme inhibition assay. The synthesized sulfamoyl derivatives were tested for their enzyme inhibitory potential against the four isoforms of the h-NTPDases by using the reported method with minor modifications.30 The obtained results are presented in Table 1.
Table 1 Presentation of the % inhibition ± S.D or IC50 ± SEM (μM) values at 100 μM compound concentration
Codes |
h-NTPDase1 IC50 ± SEM (μM) or % inhibition ± S.Da |
h-NTPDase2 IC50 ± SEM (μM) or % inhibition ± S.Da |
h-NTPDase3 IC50 ± SEM (μM) or % inhibition ± S.Da |
h-NTPDase8 IC50 ± SEM (μM) or % inhibition ± S.Da |
The results are presented as an average of the assays conducted in triplicate. IC50 values are determined for the compounds exhibiting over 50% inhibition of enzyme activity; SEM = standard error mean; SD = standard deviation. |
2a |
25 ± 3.2% |
10 ± 1.2% |
1.32 ± 0.06 |
24 ± 2.5% |
2b |
15 ± 2.3% |
8 ± 0.7% |
35 ± 1.5% |
9 ± 3.3% |
2c |
3 ± 1.2% |
22 ± 1.2% |
37 ± 2.1% |
25 ± 2.4% |
2d |
3 ± 1.2% |
19 ± 0.9% |
41 ± 1.4% |
0.28 ± 0.07 |
2e |
12 ± 3.4% |
15 ± 1.4% |
46 ± 1.3% |
36 ± 2.3% |
3a |
5 ± 1.1% |
36 ± 1.7% |
1.33 ± 0.05 |
1.78 ± 0.08 |
3b |
34 ± 2.3% |
46.91 ± 2.31 |
46.16 ± 2.41 |
41.48 ± 2.7 |
3c |
42 ± 1.4% |
2.88 ± 0.08 |
1.49 ± 0.51 |
46 ± 2.1% |
3d |
28 ± 2.2% |
44 ± 2.3% |
41 ± 1.3% |
35 ± 2.4% |
3e |
17.49 ± 1.23 |
15 ± 1.2% |
9 ± 3.2% |
24 ± 3.1% |
3f |
>100 |
0.27 ± 0.08 |
6.71 ± 0.04 |
29 ± 4.5% |
3g |
26 ± 3.1% |
31 ± 3.2% |
3.21 ± 0.09 |
39 ± 3.2% |
3h |
16 ± 2.3% |
32 ± 1.5% |
2.54 ± 0.05 |
38 ± 1.5% |
3i |
2.88 ± 0.13 |
43 ± 1.4% |
0.72 ± 0.11 |
32 ± 3.5% |
3j |
37 ± 1.3% |
0.29 ± 0.07 |
3.16 ± 0.12 |
36 ± 1.7% |
4a |
9 ± 2.3% |
35 ± 4.6% |
22 ± 2.1% |
9 ± 2.1% |
4b |
19 ± 2.2% |
25 ± 3.7% |
37 ± 3.4% |
31.79 ± 2.51 |
4c |
17 ± 4.1% |
46.91 ± 2.07 |
9 ± 1.2% |
35 ± 1.2% |
4d |
36 ± 2.4% |
0.13 ± 0.01 |
0.20 ± 0.07 |
2.46 ± 0.09 |
4e |
42 ± 1.3% |
2.24 ± 0.03 |
12 ± 2.4% |
3 ± 1.2% |
Suramin |
16.1 ± 1.08 |
24.1 ± 0.15 |
4.30 ± 0.84 |
101.1 ± 2.34 |
Structure–activity relationship. In order to clearly understand the structure–activity relationship (SAR) of the various synthesized benzene sulfonamide-carboxamide derivatives influencing the activity of the h-NTPDases isoforms i.e., h-NTPDases1, h-NTPDases2, h-NTPDases3, and h-NTPDases8, the derivatives may be divided into three groups depending upon the difference in the structures. Group A: sulfamoylbenzoic acids (2a–2e); Group B: sulfamoyl-benzamides (3a–3j) and Group C: bis-sulfonamide-carboxamide (4a–4e).
Sulfamoylbenzoic acids 2a–2e (Group A). The sulfamoyl group substituted derivatives 2a–2e tabulated the complex potential to inhibit the activity of either of the h-NTPDases isoforms depending upon the nature of the substituted groups. The study of the SAR of the derivatives 2a and 2b revealed that N-cyclopropyl ring substitution with the sulfonyl group (2a) was more favourable for the inhibition of the h-NTPDases3 activity (IC50 = 1.32 ± 0.06 μM) as compared to the morpholine ring substituted compound 2b with the sulfonyl group which was devoid of any considerable inhibitory potential against any of the h-NTPDases isoenzyme. However, the selectivity of compound 2a was moved to h-NTPDases8 in case of the presence of chlorine atom at position 2 of the benzene ring in compound 2d. On the other hand, a comparison of the activity of the 2b and chlorine substituted 2e does not enhance the activity of the compound i.e., lower than 50%, suggesting that the attachment with the sulfonyl group is of much importance.
Sulfamoyl-benzamides 3a–3j (Group B). The SAR study of the sulfamoyl-benzamide derivatives differs concerning attachments with the amide group and continuously the cyclopropyl sulfonamide group yielded reliable results. The compound 3a with chlorophenyl attachment with the amide group significantly reduced the activity of the isoforms h-NTPDases3 and h-NTPDases8 with IC50 values of 1.33 ± 0.05 μM and 1.78 ± 0.08 μM, respectively. However, this activity was reduced to IC50 values of 46.16 ± 2.41 μM and 41.48 ± 2.7 μM against the same enzymes for the compound 3b possessing the methoxyphenyl attachment with the amide group suggesting that the presence of electron withdrawing atom (chlorine) increased the resonance stability of the molecule in 3a which was much effective for the inhibitory activity as compared to the electron donating methoxy group in compound 3b. More interestingly, compound 3c regained the inhibitory potential against the h-NTPDases2, h-NTPDases3, and h-NTPDases8 to the considerable IC50 values of 2.88 ± 0.08 μM, 1.49 ± 0.51 μM, and 5.34 ± 0.73 μM, respectively. The increase in the inhibitory potential may be attributed to the presence of the n-butyl linkage which enhanced the binding of the 3c in the hydrophobic region of the enzyme's active site. On the contrary, the presence of the heterocyclic morpholine ring abolished the activity against all four isoforms of h-NTPDases.The observation of the SAR of the compounds 3e–3h with morpholine ring attached to the sulfonyl group and variation with respect to the amide linkage, the compound 3e was found although least but selective inhibitor of the h-NTPDase1 which blocked the activity presenting the IC50 = 17.49 ± 1.23 μM, whereas the molecule 3f more specifically inhibited the activity of the isoform with IC50 values equals 0.27 ± 0.08 μM. The two compounds 3g and 3h selectively reduced the activity of the h-NTPDase3 to almost equal extent i.e., 3.21 ± 0.09 μM and 2.54 ± 0.05 μM. The effect of the compounds 3g and 3h may be attributed to the presence of the methyl and methylene groups which contributed to the sigma bond hyperconjugation bonding effect to interact with the amino acids of the h-NTPDase3 catalytic site. The presence of the two halogen atoms chlorine and bromine in the structure 3i (IC50 = 2.88 ± 0.13 μM) led the compounds towards the most potent and single active molecule against the isoform h-NTPDase1. Comparison of the SAR of the compounds 3e and 3i against the h-NTPDase1 revealed that the presence of the two halogen atoms on the chemically almost similar structures enhanced the activity of compound 3i to a greater extent. The compound 3j preferentially blocked the activity of the h-NTPDase2 demonstrating the IC50 concentration of 0.29 ± 0.07 μM in accordance with the 3f which reduced the h-NTPDase2 activity to the same level. The h-NTPDase2 inhibitory effect of the 3j might be attributed to the presence of the methoxy group and the additional potency of the 3j to inhibit the h-NTPDase3 could be due to the presence of the methylene group like the structures 3g and 3h.
Bis-sulfonamide-carboxamide 4a–4e (Group C). The attachment of the chlorophenyl scaffolds on both sides of the molecules as in compound 4a, abolished the activity of the 4a against any of the h-NTPDases isoforms which can be argued that the presence of the chlorophenyl ring on the single side of the molecule was effective. The almost reduced potency was noted in compound 4b possessing morpholine ring and compound 4c with cyclopentyl ring on both sides of the compound. However, a completely opposite result was obtained in case of cyclopropyl on both sides of the molecule 4d, which retrieved the activity for the h-NTPDases2, h-NTPDases3 and h-NTPDases8 to an IC50 value of 0.13 ± 0.01 μM, 0.20 ± 0.07 μM, and 2.46 ± 0.09 μM, tabulating the most potent inhibitor of the h-NTPDases2, and h-NTPDases3 among the synthesized compounds suggesting that the small cyclic rings are effective for the activity of the compounds.
Molecular docking study
For the purpose to predict the plausible mode of interaction of selected inhibitors of each isoform i.e., h-NTPDase1, h-NTPDase2, h-NTPDase3, and h-NTPDase8, the molecular docking study was carried out by using the homology models. Followed by the docking of the compounds with respective proteins, the most reliable pose which showed a significant binding affinity with the amino acid residues was selected to visualize.
Molecular docking studies at h-NTPDase1. The molecular docking studies of the most active inhibitor 3i against the h-NTPDase1 revealed the interactions with several amino acid residues Trp450, Asp54, Tyr63, Phe360, Ile182, and Ser361 in accordance with the reported literature.31 These interactions were comprised of van der Waals forces, hydrogen bonding, π-alkyl, and π–π stacked forces. Apparently, the residues Trp450, Asp54, and Tyr63 were connected with the morpholine ring of inhibitor 3i through hydrogen bonding. A single π–π stacked interaction was observed between amino acid Phe360 and π-electrons of the phenyl ring of the bromo-phenyl scaffold. Moreover, the bromine atom was connected with the Ile182 and Phe360 via alkyl and π-alkyl interactions, respectively as depicted in Fig. 2a.
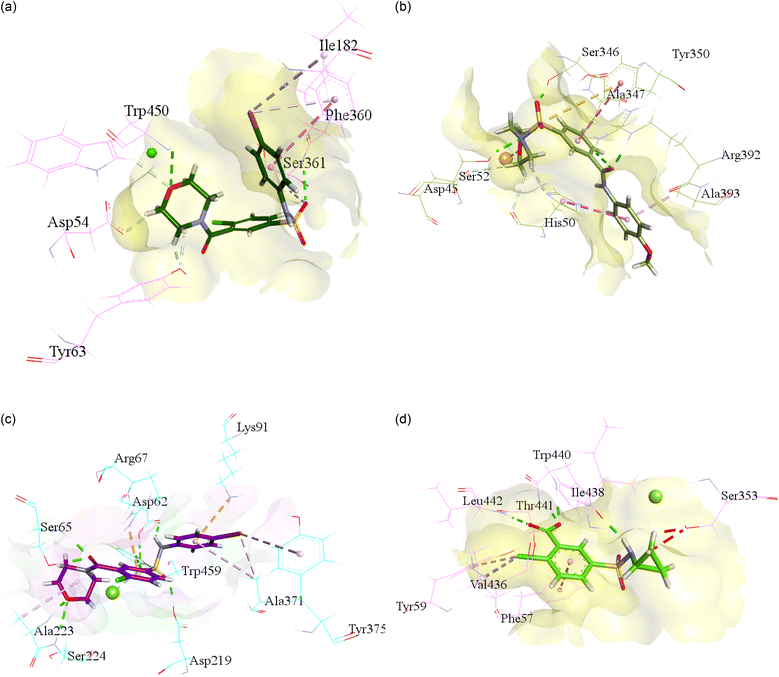 |
| Fig. 2 Illustration of molecular docking of the most potent NTPDases inhibitor against modelled h-NTPdase1, h-NTPdase2, h-NTPdase3, and h-NTPdase8. (a) Illustration of the plausible mode of interaction of compounds 3i within the h-NTPDase1 catalytic site. (b) Illustration of the plausible mode of interaction of compounds 3f within the h-NTPDase2 catalytic site. (c) Illustration of the plausible mode of interaction of compounds 3i within the h-NTPDase3 catalytic site. (d) Illustration of the plausible mode of interaction of compounds 3i within the h-NTPDase8 catalytic site. | |
Molecular docking studies at h-NTPDase2. In the case of the molecular docking of the most potent inhibitor of h-NTPDase2 shown that molecule 3f was surrounded by an array of multiple bonds (Fig. 2b). The previously reported amino acids such as: His50, Tyr350, Ser346, Arg245, Arg392, Ala393, and Ala347 were found in interactions with the molecule 3f.32 The H-bonding were observed among the His50 – morpholine ring, Asp45 – morpholine ring, Ser52 – morpholine ring, Ser346 – oxygen atom of sulfonyl group, and between the residue Arg392 and oxygen atom of acetamide moiety. The sulfur atom of the sulfonyl group was found in linkage with the residue Tyr350. The phenyl rings of the inhibitor 3f were surrounded by the residues His50 and Tyr350 through the π–π interaction, and by the residues Ala393 and Ala347 via the π-alkyl linkage. Moreover, the oxygen atom of the morpholine ring formed metal chelation with the calcium ion of the protein.
Molecular docking studies at h-NTPDase3. The molecular docking study of the active inhibitor 3i with the isoform h-NTPDase3, was performed by using the homology model of protein h-NTPDase3. A visualization of the binding pattern of molecule 3i declared a complex sequence of interactions with the amino acid residues as highlighted in the reported study (Fig. 2c).29 These interactions include hydrogen bonding, π-cation, π-sulfur, alkyl, and π-alkyl linkages. The hydrogen bonding was found among Ala223, Ser224, and morpholine scaffold; Ser65 and the oxygen atom of the amide group; Asp219, Trp459 and oxygen atoms of the sulfonyl group; Trp459 and the oxygen atom of the sulfonyl group; Asp62 and the oxygen atom of sulfonyl group; Asp62 and hydrogen atom of –NH moiety. Both aromatic rings were connected via π electrons to the important amino acids Arg67 and Lys91 through π-cation linkage. Similarly, the sulfur atom of inhibitor 3i was found to be connected with the π electrons of the phenyl ring of the residue Trp459. Moreover, metal chelation was observed between the magnesium metal ion and the oxygen atom of the sulfamoyl group of compounds 3i.
Molecular docking studies at h-NTPDase8. The docking conformation of the inhibitor 2d and the place of binding interactions within or around the h-NTPDase8 active site is shown in Fig. 2d. The binding site of the protein h-NTPDase8 is comprised of the important amino acid residues such as Phe57, Tyr59, Tyr178, Gly179, Gly435, Ile438, Val436, Thr441, and Leu442 comprising.26,29 The oxygen and hydrogen atoms of the carboxylic group were involved in the H-bond formation with the residues Trp440, Thr441, and Leu442, moreover, the hydrogen atom attached with the nitrogen atom of the sulfamoyl group was also connected via H-bond interaction with the amino acid Ile438. A π–π stacked connection was found between the aromatic ring and the Phe57, a π-alkyl interaction was observed among the chlorine atom of the chlorophenyl ring and the Tyr59, and an alkyl bridge was located between the chlorine atom and the residue Val436. Most importantly, an unfavourable bump was present between the cyclopropyl ring and the residues Ser353 which demonstrate the high binding affinity of the molecule 2d with the active site residues.
Conclusions
In conclusion, the sulfamoylbenzoic acids (2a–2e), sulfamoyl-benzamides (3a–3j), and bis-sulfonamide-carboxamide (4a–4e) were synthesized and tested for their effect on the activity of the h-NTPDase1, h-NTPDase2, h-NTPDase3, and h-NTPDase8. As a result, many potent and selective inhibitors of each isozyme were found which blocked the activity from micromolar to sub-micromolar concentrations. The compound 3i (IC50 = 2.88 ± 0.13) was obtained as most potent inhibitor of h-NTPDases1; compounds 3f (IC50 = 0.27 ± 0.08 μM), 3j (IC50 = 0.29 ± 0.07 μM) and 4d (IC50 = 0.13 ± 0.01 μM) preferably reduced the function of the h-NTPDase2. The potent inhibitor of h-NTPDase1 (3i) was also very active against the activity of the h-NTPDase3 with IC50 concentrations equal to 0.72 ± 0.11 μM and the compound 2d selectively blocked the activity of the h-NTPDase8 presenting the IC50 value of 0.28 ± 0.07 μM. Moreover, the molecular docking interactions of the most potent compounds with the amino acid residues of the h-NTPDases proteins justified the in vitro results. In short, the molecules screened as potent and selective inhibitors of the h-NTPDases isozymes may be further evaluated as drug candidates against the diseases associated with the aberrant activity of these enzymes.
Experimental
Synthesis of the compounds
The detailed procedure for the synthesis of the sulfamoyl-benzamides and their analytical data is given in the ESI† file.
Biological section
Enzyme inhibition assay h-NTPDases. The sulfamoyl derivatives were tested for their enzyme inhibitory potential against the four isoforms of the h-NTPDases by using the previously reported method with minor modifications.30 The malachite green assay was performed in an assay buffer which was composed of the components such as 5 mM of calcium chloride, and 50 mM of tris-hydrochloric acid maintained to a pH of 7.4 at room temperature. The compounds were examined in triplicate at the concentration of 100 μM for each well. The incubation time of 10 min was given to the assay plate containing the assay buffer, 100 μM of the compound, 12 ng of h-NTPDase1, or 37 ng of h-NTPDase2, or 43 ng of h-NTPDase3, and 63 ng of h-NTPDase8. After taking the reading with the assistance of microplate reader Omega FLUOstar microplate reader (BMG Labtech, Germany) at 630 nm, the substrate (100 μM) was added to initiate the reaction and incubated for 15 min at a temperature of 37 °C. After 15 min incubation, malachite green reagent (15 μL) was added to each well and reading was noted up to 6 min. The % enzyme inhibition results were calculated and the inhibitors which showed above 50% reduction of the respective enzyme were subjected to the serial ascending concentrations and half of the maximal compound concentration (IC50) was determined by using PRISM 5.0 (GraphPad, San Diego, California, USA).
Molecular docking study. Due to the unavailability of the crystallographic structures of the h-NTPDases, the already developed homology models were used for molecular docking study.33 The structures of the compounds were drawn by using ChemDraw software and energy was minimized with the help of MOE (Molecular Operating Environment). The compounds were saved in Mol2 formats for docking studies in the active pocket of the enzyme.34 The preparation of proteins, selection of the active pocket, and other parameters were selected by using the software LeadIT (BioSolveIT GmbH, Germany) and molecular docking study was carried out.35 The 50 poses for each ligand were visualized and the ligand showing the highest binding affinity and low interaction energy were selected with the help of Discovery Studio Visualizer DS.36
Conflicts of interest
We have no conflicts of interest to disclose.
Acknowledgements
AH is grateful to the Higher Education Commission (HEC) Pakistan for NRPU research grant 20-3885/R&D/HEC/14. JI acknowledges the financial support provided by the HEC via NRPU project No. 20-15846/NRPU/R&D/HEC/2021.
Notes and references
- I. Grković, D. Drakulić, J. Martinović and N. Mitrović, Curr. Neuropharmacol., 2019, 17, 84–98 CrossRef PubMed.
- S.-Y. Lee and C. E. Müller, MedChemComm, 2017, 8, 823–840 RSC.
- S.-Y. Lee, S. Sarkar, S. Bhattarai, V. Namasivayam, S. De Jonghe, H. Stephan, P. Herdewijn, A. El-Tayeb and C. E. Müller, Front. Pharmacol., 2017, 8, 54 Search PubMed.
- D. D. Nabinger, S. Altenhofen and C. D. Bonan, Prog. Neuro-Psychopharmacol. Biol. Psychiatry, 2020, 98, 109770 CrossRef CAS PubMed.
- M. Tozzi and I. Novak, Front. Pharmacol., 2017, 8, 878 CrossRef PubMed.
- H. Ahmad, S. Ullah, F. Rahman, A. Saeed, J. Pelletier, J. Sévigny, A. Hassan and J. Iqbal, Eur. J. Med. Chem., 2020, 208, 112759 CrossRef CAS PubMed.
- K. Hayat, S. Afzal, A. Saeed, A. Murtaza, S. Ur Rahman, K. M. Khan, A. Saeed, S. Zaib, J. Lecka, J. Sévigny, J. Iqbal and A. Hassan, Bioorg. Chem., 2019, 87, 218–226 CrossRef CAS PubMed.
- A. J. Marcus, M. J. Broekman, J. H. F. Drosopoulos, K. E. Olson, N. Islam, D. J. Pinsky and R. Levi, Semin. Thromb. Hemostasis, 2005, 31, 234–246 CrossRef CAS PubMed.
- G. Kauffenstein, C. R. Fürstenau, P. D'Orléans-Juste and J. Sévigny, Br. J. Pharmacol., 2010, 159, 576–585 CrossRef CAS PubMed.
- S. C. Robson, J. Sévigny and H. Zimmermann, Purinergic Signalling, 2006, 2, 409–430 CrossRef CAS PubMed.
- F. Kukulski, S. A. Lévesque and J. Sévigny, in Advances in Pharmacology, ed. K. A. Jacobson and J. Linden, Academic Press, 2011, vol. 61, pp. 263–299 Search PubMed.
- C. E. Müller, J. Iqbal, Y. Baqi, H. Zimmermann, A. Röllich and H. Stephan, Bioorg. Med. Chem. Lett., 2006, 16, 5943–5947 CrossRef PubMed.
- S. Afzal, M. al-Rashida, A. Hameed, J. Pelletier, J. Sévigny and J. Iqbal, Front. Pharmacol., 2020, 11, 585876 CrossRef CAS PubMed.
- Y. Lavanya, Int. J. Pharm. Sci. Invent., 2017, 6, 1–3 Search PubMed.
- S. Seino, Diabetologia, 2012, 55, 2096–2108 CrossRef CAS PubMed.
- Y. Ö. Genç and Y. Reşit Bekdemir, Ann. Clin. Microbiol. Antimicrob., 2008, 7, 1–6 CrossRef PubMed.
- M. H. Mengelers and Janssen, J. Vet. Pharmacol. Ther., 1997, 20, 276–283 CrossRef CAS PubMed.
- X. Zhang, A. Xu, J. Lv, Q. Zhang, Y. Ran, C. Wei and J. Wu, Eur. J. Med. Chem., 2020, 185, 111822 CrossRef CAS PubMed.
- M. Lamkanfi, J. L. Mueller, A. C. Vitari, S. Misaghi, A. Fedorova, K. Deshayes, W. P. Lee, H. M. Hoffman and V. M. Dixit, J. Cell Biol., 2009, 187, 61–70 CrossRef CAS PubMed.
- C. Guo, J. W. Fulp, Y. Jiang, X. Li, J. E. Chojnacki, J. Wu, X.-Y. Wang and S. Zhang, ACS Chem. Neurosci., 2017, 8, 2194–2201 CrossRef CAS PubMed.
- F. Mincione, M. Starnotti, L. Menabuoni, A. Scozzafava, A. Casini and C. T. Supuran, Bioorg. Med. Chem. Lett., 2001, 11, 1787–1791 CrossRef CAS PubMed.
- Y. F. Suen, L. Robins, B. Yang, A. S. Verkman, M. H. Nantz and M. J. Kurth, Bioorg. Med. Chem. Lett., 2006, 16, 537–540 CrossRef CAS PubMed.
- K. R. Darren, W. M. David and R. S. Jonathan, Weed Technol., 1994, 8, 630–634 CrossRef.
- Z. Zhao, S. E. Wolkenberg, M. Lu, V. Munshi, G. Moyer, M. Feng, A. V. Carella, L. T. Ecto, L. J. Gabryelski, M.-T. Lai, S. G. Prasad, Y. Yan, G. B. McGaughey, M. D. Miller, C. W. Lindsley, G. D. Hartman, J. P. Vacca and T. M. Williams, Bioorg. Med. Chem. Lett., 2008, 18, 554–559 CrossRef CAS PubMed.
- A. M. F. Galal, M. M. Soltan, E. R. Ahmed and A. G. Hanna, MedChemComm, 2018, 9, 1511–1528 RSC.
- G. Zaman, S. Ullah, M. Uzair, S. Batool, H. Ahmad, F. Ullah, J. Pelletier, J. Sévigny, J. Iqbal and A. Hassan, ChemMedChem, 2023, e202300165 CrossRef PubMed.
- Z. Begum, S. Ullah, M. Akram, M. Uzair, F. Ullah, Ahsanullah, J. Pelletier, J. Sévigny, J. Iqbal and A. Hassan, Bioorg. Chem., 2022, 129, 106196 CrossRef CAS PubMed.
- J. P. Bassin, R. J. Cremlyn and F. J. Swinbourne, Phosphorus, Sulfur Silicon Relat. Elem., 1991, 56, 245–275 CrossRef CAS.
- A. Murtaza, S. Afzal, G. Zaman, A. Saeed, J. Pelletier, J. Sévigny, J. Iqbal and A. Hassan, Bioorg. Chem., 2021, 115, 105240 CrossRef CAS PubMed.
- S. A. Lévesque, É. G. Lavoie, J. Lecka, F. Bigonnesse and J. Sévigny, Br. J. Pharmacol., 2007, 152, 141–150 CrossRef PubMed.
- M. Zebisch, M. Krauss, P. Schäfer and N. Sträter, J. Mol. Biol., 2012, 415, 288–306 CrossRef CAS PubMed.
- M. Zebisch and N. Sträter, Proc. Natl. Acad. Sci., 2008, 105, 6882–6887 CrossRef CAS PubMed.
- J. Iqbal and S. J. A. Shah, Sci. Rep., 2018, 8, 2581 CrossRef PubMed.
- MOE (Molecular Operating Environment) Version 2019.0201, Chemical Computing Group (CCG), 2019 Search PubMed.
- LeadIT version 2.3.2, BioSolveIT GmbH, Sankt Augustin, Germany, 2017 Search PubMed.
- Dassault Systemes BIOVIA, Discovery Studio Modeling Environment, Release 2017, Dassault Systemes, San Diego, 2016 Search PubMed.
|
This journal is © The Royal Society of Chemistry 2023 |
Click here to see how this site uses Cookies. View our privacy policy here.