DOI:
10.1039/D3RA04150F
(Paper)
RSC Adv., 2023,
13, 23396-23401
Coordination-directed self-assembly of nano-cages: metal ion-change, ligand-extending, shape-control and transdermal drug delivery†
Received
20th June 2023
, Accepted 19th July 2023
First published on 4th August 2023
Abstract
The combination of different pyridyl ligands and metal ions has proven to be a very reliable strategy for controlling the coordination mode of the heterometallic coordination nano-cages. Adjusting the length of the ligands could result in the selective synthesis of several heterometallic coordination nano-cages, either [8Rh + 2M]-4L, [8Rh + 2M]-5L or [8Rh + 4M]-6L cages, derived from the very same precursors (LH3tzdc) through half-sandwich rhodium self-assembly. Moreover, a series of [8Rh + 4M]-6L cages was chosen to exemplify the preparation. The rigidity of various pyridyl donor ligands caused the vertical nano-cage to be energetically preferred and was able to change the self-assembly process through ligand flexibility to selectively give the inclined nano-cage and cross nano-cage.
1. Introduction
Over the past two decades, coordination-driven self-assembly has developed into an efficient way to construct diverse metal–organic metallacycles and nano-cages with well-defined shapes, sizes and applications.1–5 The structural outcomes of metalla-supramolecular self-assembly processes are largely controlled by factors such as the building blocks, metal ions, and organic ligands.6 By choosing an appropriate ligand, it is possible to influence the size, geometry, and functionality of the resulting metalla-supramolecular assembly. Heterometallic coordination complexes have attracted considerable attention due to their aesthetically pleasing structures and also their potential applications in catalysis, electronic, magnetic, host–guest, drug delivery and other fields.7–10 However, the construction of well-defined metalla-supramolecular coordination complexes, especially stable large structures, presents a challenge because changing the metal ions or an increase in linker length may lead to an increase in structure flexibility and multiple stereochemical configurations. Therefore, the use of different metal ions and ligands to control the assembly of metallosupramolecular coordination complexes is a well-established methodology.
The metal centers can be an extremely important consideration when designing heterometallic assemblies and represent a significant advantage. Supramolecular chemistry based on Cp*M (M = Ir, Rh) and (p-cymene) Ru units has grown rapidly since the first report of the organometallic Cp*Rh fragment being used as a building block for the construction of discrete heterometallic architectures with metal acceptor units and nitrogen donor ligands.11,12 The coordination of pyridyl-based ligands with Cp*Rh or Cp*Ir fragments has proven very useful for constructing a wide array of metallamacrocycles, which has enabled the construction of a variety of metal–organic cages.13–22
In our previous work, structures ranging from a one-dimensional (1D) organometallic chain up to 3D discrete heterometallic nano-cages have been constructed from half-sandwich Cp*Rh units with triazole proligand 1,2,3-triazole-4,5-dicarboxylate (LH3tzdc) and linear N-donor ligands of different lengths.23–33 Scheme 1 shows the design and self-assembly of discrete coordination cages based on three modes. The rigid ligands are a prerequisite for the assembly of four-ligand coordination nano-cages and flexible ligands tend to favor diverse complexes of five-ligand coordination nano-cages. On changing the center metal ions, it is also possible to influence the metal nuclearity, quantity of ligands and the resulting six-ligand coordination mode of the assemblies. Therefore, the strategic adjustment of the length of the pyridyl donor ligands and changing the metal center ions allowed us to control the self-assembly behavior of the systems.34–39 Transdermal drug delivery systems (TDDSs) are an innovative approach for delivering drugs into the blood at a controlled rate via the skin. TDDSs avoid the first-pass effect and improve patient compliance because they are applied topically. Heterometallic cage compounds are expected to be outstanding TDDS materials but to the best of our knowledge, such materials have yet to be reported in the literature. However, the construction of well-defined metalla-supramolecular coordination complexes, especially stable larger structures, presents a challenge because changing the metal ions or increasing the linker length may lead to an increase in the structural flexibility and multiple stereochemical configurations. Therefore, the use of different metal ions and ligands to control the assembly of metallosupramolecular coordination complexes is a well-established methodology (Fig. 1).
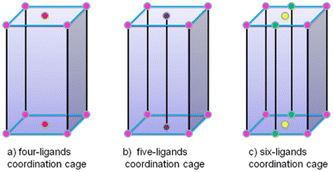 |
| Scheme 1 The three modes of self-assembly of heterometallic coordination cages observed in this work, with different metal centers and ligands (pink for Rh, red for Zn, Ni, or Na; purple for Zn or Ni; yellow for Na or K, green for Cu). | |
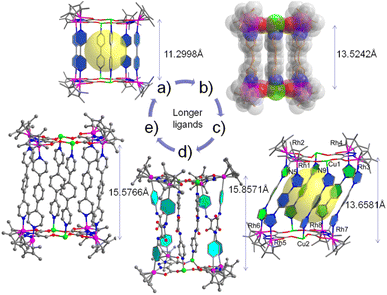 |
| Fig. 1 (a)–(e) Single-crystal structures of 1–5 (gray for C, blue for N, red for O, green for Cu, pink for Rh, orange for Na, yellow for S, fluorescent green for F; Na some hydrogen atoms and solvent molecules or anions are omitted for clarity). | |
2. Results and discussion
As shown in Scheme 2, a variety of supramolecular coordination assemblies, from a 1D chain structure to 2D (M = Cu) polygons and 3D (M = Cu, Na) nano-cages, have been constructed by using metal ions to tune their cage sizes for potential applications.40–51 The sodium or potassium salt of LH3tzdc was added to a solution of [Cp*RhCl2]2 in MeOH. The reaction mixture was stirred with AgOTf for 12 h, and then sixfold pyridyl donor ligands (L1 to L5) with the metal salt Cu(NO3)2·3H2O were added to the mixture, and five kinds of [8Rh + 4Cu]-6L cages were obtained respectively. In such an assembly process, it is crucial to select appropriate metal ions that can be linked by the ligands. Cu–O linkages are more flexible and have versatile coordination behavior and favorable hard–soft combination. A variety of heteroleptic complexs 1–5 nano-cages were generated from the combination of sixfold pyridyl donor ligands. In Fig. 2a, the vertical nano-cage of complex 1 consists of eight Cp*Rh fragments at the corners, and the two pairs of [4Rh + 2Cu] units are bridged with sixfold L1. Each [4Rh + 2Cu] unit is formed by two Cu(II) ions and four Cp*Rh units, which are chelated by two LHtzdc units. By utilizing LHtzdc-based units that coordinate with Cu(II) ions as building blocks, we have synthesized the nano-cage complex 2 in Fig. 2b, displaying a Rh1A⋯Rh1B distance of 13.5242(4) Å, Na1A⋯Na1B distance of 13.3227(7) Å and a Cu1A⋯Cu1B distance of 13.4794(4) Å. The nano-cage of complex 1 is quite similar to that of complex 2 and has a cuboid cavity with dimensions of 6.19 × 11.21 × 10.48 Å according to Rh⋯Rh non-bonding distances, which is much larger than that of complex 9 6.20 × 13.52 × 10.40 Å. Complexes 3 (Fig. 2c) and 4 (Fig. 2d) are inclined cuboid nano-cages with six L3 or L4 units in a symmetrical arrangement. In complex 3, each copper ion is coordinated to four oxygen atoms from the carboxylate groups of symmetry-related LHtzdc with Cu1A–Cu1B distances of 6.3602(2) Å.
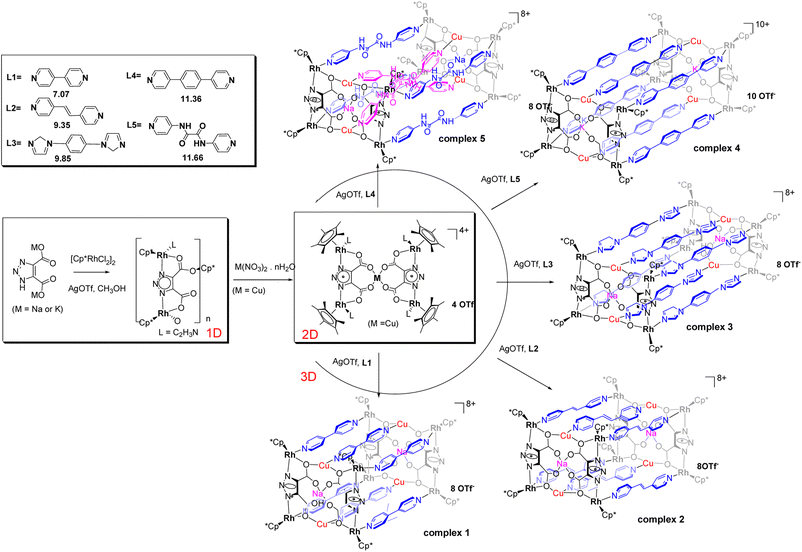 |
| Scheme 2 The synthetic routes to 1D chain structures, 2D polygons and 3D nano-cages. | |
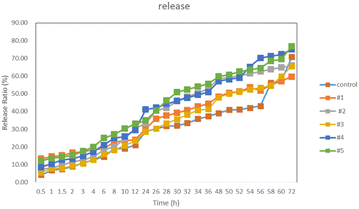 |
| Fig. 2 In vitro drug release of Febuxostat tablets with complexes. | |
Fig. 2e shows a cross nano-cage, with four ligands forming the side walls and two ligands coordinating to the four Cu(II) ions. Inside the structure, each Cu(II) ion is distinctly coordinated to two L5 ligands and two oxygen atoms from two LHtzdc. Interestingly, the two L5 ligands resemble a cross occurring in the interior of the structure, and the crossed coordination motif resembles a “helicate”. Complex 5 shows Cu1A⋯Cu1B distances of 15.6461(6) Å, with an inclination angle (Rh4–Rh1–Cu1) of 65.363(1) °. Outside of the nano-cage, four L5 ligands are coordinated to eight Cp*Rh units, similarly generating a right-handed helical mode. Despite many attempts, no incorporation of guest molecules in the cavities of the structures was observed (except for solvent molecules).
In Fig. 2, the release of Febuxostat tablets from the complexes was investigated using a classic transdermal drug release study with a two-chamber diffusion cell. Except for complex 2, the other four complexes can lead to a significant increase in drug release as compared with the control, which indicates that the interaction between Febuxostat tablets and the complexes for 24 hours is beneficial for drug release. These four complexes play a role in the sustained release of drugs to different degrees, which can effectively reduce the number of times that the drugs need to be taken, reduce the toxicity and side effects of drugs and reduce the total amount of drugs used. Complexes 4 and 5 have better effects; 4 is a linear-type nano-cage with a larger inner cavity and 5 is a screw-type nano-cage. Although the structures of the two compounds are different, the effects are not much different, which shows that all the nano-cage compounds can play a good role in drug delivery.
An in vitro skin penetration assay is one way to predict drug penetration in vivo and has been validated by in vitro correlation studies. The amount of skin penetration in complex 3 was 280 μg cm−2; the results from the caged drug-loading were the best when the side arms of the caged molecules were longer and there was no cross-coordination in the caged molecules (Fig. 3). The experimental results showed that the drug-loading performance of the compound can be improved by extending the side arm of the molecular cage with divalent copper ions as the center. However, a longer side arm was not better for complex 4 because the side arm of complex 4 is flexible enough to cross-coordinate, and the internal space of the molecular cage is small; the drug-loading effect of complex 5 with the longest side arm is not ideal because the drug-carrying molecules will specifically choose the size of nano-cage and diameter.
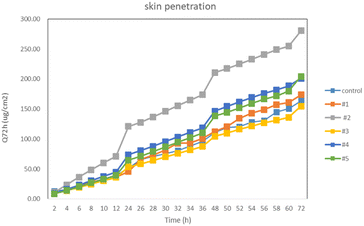 |
| Fig. 3 In vitro skin penetration of Febuxostat tablets released from the complexes. | |
The biocompatibility of the complexes with skin was assessed by a non-invasive in vivo skin erythema test. As shown in Fig. 4, in the group of 10% SDS, the ΔEI increased significantly, indicating that the skin reacted normally to irritants. The application of the complexes suspended in water did not increase ΔEI, indicating high biocompatibility with skin.
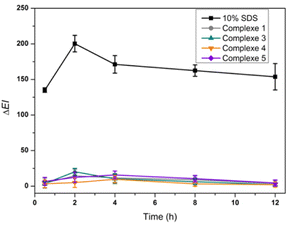 |
| Fig. 4 Biocompatibility of the complexes. | |
3. Experimental
3.1 Synthesis and characterization of complexes 1–5
3.1.1 Synthesis of complexes 1–5. 1,2,3-triazole-4,5-dicarboxylic acid (LHtzdc) (157 mg, 0.1 mmol) and NaOH (12.0 mg, 0.3 mmol) or KOH (17.1 mg, 0.3 mmol) were stirred strongly in 10 mL of MeOH at room temperature for 12 h, affording a slight yellow creamy slurry of the sodium salt. The solvent was removed by filtration and the remaining solid was washed with Et2O (20 mL), followed by the addition of the solution of [Cp*RhCl2]2 (61.8 mg, 0.1 mmol), and sodium salt (20.1 mg, 0.1 mmol in 10 mL of MeOH). The metal salt Cu(NO3)2·3H2O (12.1 mg, 0.05 mmol) was then added to the solution. AgOTf (51.2 mg, 0.2 mmol) was stirred in the dark at room temperature for 12 h, and then filtered to remove insoluble materials. After that, L1 (23.4 mg, 0.15 mmol), L2 (27.3 mg, 0.15 mmol), L3 (31.5 mg, 0.15 mmol), L4 (33.8 mg, 0.15 mmol), or L5 (36.3 mg, 0.15 mmol) was added to the mixture. After 12 h of stirring, a yellow-green mixture resulted, and MeOH was evaporated under a vacuum. Crystals of complexes 1–5 that were suitable for an X-ray diffraction study were obtained by the slow diffusion of diethyl ether into a mixture solution in MeOH and CH3CN (1
:
1).
3.1.2 Characterization of complex 1. Green solid, yield: 89.4%, 114.2 mg. Anal. calcd for C182H310Cu4F18N32Na2O109Rh8S6: C, 34.43; H, 4.92; N, 7.06; found: C, 34.66; H, 4.56; N, 7.28; IR (KBr disk): 3649, 3629, 3481, 2923, 1611, 1577, 1529, 1491, 1384, 1274, 1168, 1073, 1032, 937, 820, 785, 640, 576, 518, 454 cm−1.
3.1.3 Characterization of complex 2. Green solid, yield: 88.5%, 127.2 mg. Anal. calcd for C194H244Cu4F30N28Na2O82Rh8S10: C, 37.02; H, 3.91; N, 6.23; found: C, 37.31; H, 3.70; N, 6.02; IR (KBr disk): 3447, 1612, 1430, 1384, 1280, 1258, 1224, 1160, 1068, 1031, 1168, 836, 784, 638, 573, 557, 517, 473 cm−1.
3.1.4 Characterization of complex 3. Green solid, yield: 87.1%, 128.9 mg. Anal. calcd for C192H246Cu4F24N41NaO72Rh8S8: C, 37.85; H, 4.07; N, 9.42; found: C, 37.48; H, 4.24; N, 9.25; IR (KBr disk): 3482, 3132, 2920, 1577, 1552, 1530, 1405, 1354, 1384, 1275, 1224, 1161, 1069, 1030, 937, 782, 652, 638, 613, 454 cm−1.
3.1.5 Characterization of complex 4. Green solid, yield: 85.9%, 130.1 mg. Anal. calcd for C234H270.4Cu4F30K2N24O67.2Rh8S10: C, 42.97; H, 4.17; N, 5.14; found: C, 42.64; H, 4.31; N, 5.39; IR (KBr disk): 3511, 2927, 1659, 1611, 1521, 1501, 1430, 1386, 1344, 1257, 1158, 1100, 1065, 1031, 843, 787, 638, 573, 559, 517, 472, cm−1.
3.1.6 Characterization of complex 5. Green solid, yield: 88.7%, 135.5 mg. Anal. calcd for C182H232Cu4F24N44Na2O82Rh8S8: C, 35.35; H, 3.78; N, 9.97; found: C, 35.71; H, 3.48; N, 9.68; IR (KBr disk): 3447, 1712, 1615, 1582, 1508, 1384, 1333, 1281, 1225, 1212, 1160, 1064, 1030, 837, 788, 639, 574, 556, 517 cm−1.
3.2 In vitro drug release study
Drug-in-adhesive patch with 5% materials and 4% ketoprofen (KP) was prepared using solvent evaporation method. A drug release study was conducted with modified Franz diffusion cells at 32 °C. The patch was pasted on a cellulose membrane and then fixed onto the receptor cell with phosphate buffer solution (PBS, pH 7.4) of 4.5 mL. Samples of 2.0 mL were collected at 0.5, 1, 1.5, 2, 3, 4, 6, 8, 10, 12, 24, 26, 28, 30, 32, 34, 36, 48, 50, 52, 54, 56, 58, 60 and 72 h, and then replaced with the same volume of fresh PBS. The drug concentration was determined by Hitachi HPLC (Tokyo, Japan), which consisted of a pump L-2130, auto sampler L-2200 and UV-detector L-2420 and Waters C18 reversed-phase column (Waters, America) (200 × 4.6 mm, 5 μm). The mobile phase of KP was a mixture of methanol, water and acetic acid solution (60
:
40
:
0.2, v/v), and the pH was adjusted to 6.8 with triethylamine. The column temperature was 40 °C and the flow rate was 1 mL min−1. The wavelength of the detector was set at 260 nm.
A simplified Higuchi equation was used to describe the drug release behavior as follows:
|
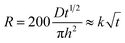 | (1) |
where
D and
h are the diffusion rate and diffusion length, respectively. The release percent
R was plotted against

and
k is the release rate.
3.3 In vitro skin penetration study
Male Wistar rats (180–220 g, 68 weeks old) were supplied by Liaoning Changsheng (Liaoning, China). All the procedures were performed in accordance with the NIH guidelines for the Care and Use of Laboratory Animals and were approved by the Animal Ethics Committee of Shenyang Medical College. Full-thickness skin was prepared as follows. The hair of the abdomen skin was shaved after the rat was anesthetized with urethane (20%, w/v, 6 mL kg−1, i.p.). Abdomen skin was excised after the rat was sacrificed. The adhering subcutaneous tissues were removed carefully.
The patch was pasted on the stratum corneum and then assembled onto the modified Franz cells. Methods of sample collection and drug concentration determination were the same as for the drug release study, except that sample collection was conducted at 2, 4, 6, 8, 10, 12, 24, 26, 28, 30, 32, 34, 36, 48, 50, 52, 54, 56, 58, 60, 72 h. The cumulative drug skin penetration amount was determined by drug concentration and the volume of the sampling point.
|
 | (2) |
where
Q is the cumulative skin permeation amount,
Ci is the concentration in the receiver compartment at time
i and
A is the effective diffusion area (1.5 cm
2). The cumulative amount permeated from the unit area
versus time
i was plotted.
3.4 In vivo skin erythema study
The experimental method is as follows: Mexameter® (MX 16, Courage & Khazaka Co., Germany) was used to evaluate the biocompatibility of the complexes by measuring the erythema index (EI) of the rabbit skin. Here, a 10% (w/v) aqueous solution of sodium dodecyl sulfate (SDS) was used as the positive control. The dorsal skin of the rabbits was shaved and divided into 5 separate sections (2.5 cm × 2.5 cm). Before the application of 500 μL of PBS, the complexes (10% in PBS), or 10% SDS to their separate sections, the initial EI values were measured as EI0. After 8 h, the solutions were removed and the areas were gently cleaned with cotton wool swabs. The EIt was measured and ΔEI was calculated (ΔE = EIt − EI0). The experiment was performed in quadruplicate.
4. Conclusions
A large number of nano-cages and metallacycles with well-designed N-donor ligands and the LHtzdc ligand have been constructed using a range of central metal atoms. By choosing the template with copper ion as the central metal, we can obtain six-sided compounds, and five kinds of [8 + 4] core semi-sandwich rhodium molecular cages by adjusting the length and rigidity and flexibility. Among these, 1,2,3-triazole-4,5-dicarboxylic acid is located on the upper and lower planes of the cage, and the upper two oxygens chelate with the central metal, and the eight vertices coordinate with the rhodium metal, forming a hexahedral structure in which the side arms of each cage choose linear ligands with different lengths. Because of their different levels of rigidity and flexibility, the cage configuration changes in the form of inclination and cross-coordination. In this way, because the coordination mode of Cu–O bridge makes the compounds have more flexible and changeable coordination behavior and good hardness, various cages with complex structures (various pyridine-based ligands with 6 times the amount of side arms) are formed. The metalla-supramolecular assemblies prepared include [8Rh + 4M]-6L heterometallic nano-cages (complexes 1–5). This study demonstrates how the choice of corner metal centers and ligands in the construction of heterometallic supramolecular complexes can influence the geometry and composition of the resulting assemblies. Furthermore, the use of ligands of different lengths leads to nano-cages with different cavity sizes, which may lead to their application in transdermal drug delivery. We are currently exploring other methods of functionalization of these multicomponent nano-cages, and the use of coordination-driven self-assembly to prepare new micro-reactors.
Conflicts of interest
There are no conflicts to declare.
Acknowledgements
This work was supported by National natural science foundation (82003639), 2019 Scientific Research Fund of Shenyang Medical College (20201004, 20191023), Shenyang Medical College of Science and Technology fund project (20186071), 2018 Provincial Key Research Program Guidance Plan (2018225021), Science Prounicatom Of China (21804093).
References
- J. E. Beves, B. A. Blight, C. J. Campbell, D. A. Leigh and R. T. McBurney, Angew. Chem., Int. Ed., 2011, 50, 9260–9327 CrossRef CAS PubMed.
- D. Fujita, Y. Ueda, S. Sato, N. mizuno, T. Kumasaka and M. Fujita, Nature, 2016, 540, 563–566 CrossRef CAS PubMed.
- Q. F. Sun, J. Iwasa, D. Ogawa, Y. Ishido, S. Sato, T. Ozeki, Y. Sei, K. Yamaguchi and M. Fujita, Science, 2010, 328, 1144–1147 CrossRef CAS PubMed.
- M. Li, D. Li, M. O’ Keeffe and O. M. Yaghi, Chem. Rev., 2014, 114, 1343–1370 CrossRef CAS PubMed.
- L. L. Dang, T. T. Li, T. T. Zhang, Y. Zhao, T. Chen, X. Gao, L. F. Ma and G. X. Jin, Chem. Sci., 2022, 13, 5130–5140 RSC.
- S. M. Jansze, G. Cecot, M. D. Wise, K. O. Zhurov, T. K. Ronson, A. M. Castilla, A. Finelli, P. Pattison, E. Solari, R. Scopelliti, G. E. Zelinski, A. V. Vologzhanina, Y. Z. Voloshin, J. R. Nitschke and K. Severin, J. Am. Chem. Soc., 2016, 138, 2046–2054 CrossRef CAS PubMed.
- Y. Y. Zhang, Y. J. Lin and G. X. Jin, Chem. Commun., 2014, 50, 2327–2329 RSC.
- Y. Y. Zhang, L. Zhang, Y. J. Lin and G. X. Jin, Chem.–Eur. J., 2015, 21, 14893–14900 CrossRef CAS PubMed.
- Y. F. Han, W. G. Jia, W. B. Yu and G. X. Jin, Chem. Soc. Rev., 2009, 38, 3419–3434 RSC.
- Y. Lu, D. Liu, Y. J. Lin, Z. H. Li, F. E. Hahn and G. X. Jin, J. Am. Chem. Soc., 2021, 143, 12404–12411 CrossRef CAS PubMed.
- H. N. Zhang, W. B. Yu, Y. J. Lin and G. X. Jin, Angew. Chem., Int. Ed., 2021, 60, 15466–15471 CrossRef CAS PubMed.
- S. T. Guo, P. F. Cui, X. R. Liu and G. X. Jin, J. Am. Chem. Soc., 2022, 144, 5130–5140 Search PubMed.
- P. F. Cui, X. R. Liu, Y. J. Lin, Z. H. Li and G. X. Jin, J. Am. Chem. Soc., 2022, 144, 6558–6565 CrossRef CAS PubMed.
- B. Kilbas, S. Mirtschin, T. Riis-Johannessen, R. Scopelliti and K. Severin, Inorg. Chem., 2012, 51, 5795–5804 CrossRef CAS PubMed.
- Y. H. Song, N. Singh, J. Jaehoon, H. Kim, E. H. Kim, H. K. Cheong, Y. Kim and K. W. Chi, Angew. Chem., Int. Ed., 2015, 54, 1–6 CrossRef.
- H. W. Lee, P. Elumalai, N. Singh, H. Kim, S. U. Lee and K. W. Chi, J. Am. Chem. Soc., 2015, 137, 4674–4677 CrossRef CAS PubMed.
- F. Schmitt, J. Freudenreich, N. P. E. Barry, L. Juillerat-Jeanneret, G. Süss-Fink and B. Therrien, J. Am. Chem. Soc., 2012, 134, 754–757 CrossRef CAS PubMed.
- J. J. Li, L. H. Guo, Z. Z. Tian, S. M. Zhang, Z. S. Xu, Y. L. Han, R. X. Li, Y. Li and Z. Liu, Inorg.
Chem., 2018, 57, 13552–13563 CrossRef CAS PubMed.
- H. B. Yang, K. Ghosh, Y. Zhao, B. H. Northrop, M. M. Lyndon, D. C. Muddiman, H. S. White and P. J. Stang, J. Am. Chem. Soc., 2008, 130, 839–841 CrossRef CAS PubMed.
- Y. Sakata, S. Hiraoka and M. Shionoya, Chem.–Eur. J., 2010, 16, 3318–3325 CrossRef CAS PubMed.
- H. B. Wu and Q. M. Wang, Angew. Chem., Int. Ed., 2009, 48, 7343–7345 CrossRef CAS PubMed.
- D. Simond, S. E. Clifford, A. F. Vieira, C. Besnardb and A. F. Williams, RSC Adv., 2014, 4, 16686–16693 RSC.
- X. C. Liu and L. Lin, Inorg. Chem. Commun., 2021, 129, 108660–108665 CrossRef CAS.
- F. E. Hahn, M. Offermann, C. S. Isfort, T. Pape and R. Fröhlich, Angew. Chem., Int. Ed., 2008, 47, 6794–6797 CrossRef CAS PubMed.
- J. J Liu, Y. J. Lin and G. X. Jin, Organometallics, 2014, 33, 1283–1290 CrossRef.
- Y. Y. Zhang, Y. J. Lin and G. X. Jin, Chem. Commun., 2014, 50, 2327–2329 RSC.
- M. Schmittel, B. He and P. Mal, Org. Lett., 2008, 10, 2513–2516 CrossRef CAS PubMed.
- M. Fujita, K. Biradha, M. Fujita, S. Sakamoto and K. Yamaguchi, Angew. Chem., Int. Ed., 2001, 40, 1718–1721 CrossRef PubMed.
- A. K. W. Chan, W. H. Lam, Y. Tanaka, K. M. C. Wong and V. W. W. Yam, Proc. Natl. Acad. Sci. U. S. A., 2015, 112, 690–695 CrossRef CAS PubMed.
- L. Zhang, L. Lin, D. Liu, L. Jian, Z. H. Li and G. X. Jin, J. Am. Chem. Soc., 2017, 139, 1653–1660 CrossRef CAS PubMed.
- X. C. Liu and Li. Lin, Inorg. Chem. Commun., 2021, 129, 108660–108666 CrossRef CAS.
- H. R. Zhang, L. H. Guo, Z. Z. Tian, M. Tian, S. M. Zhang, Z. S. Xu, P. W. Gong, X. F. Zheng, J. Zhao and Z. Liu, Chem. Commun., 2018, 54, 4421–4424 RSC.
- Z. Q. Lei, X. Han, S. E. Border, J. Gallucci, R. Z. Pavlovic and J. D. Badjić, J. Am. Chem. Soc., 2018, 140, 11091–11100 CrossRef PubMed.
- S. R. Peurifoy, J. C. Russell, T. J. Sisto, Y. Yang, X. Roy and C. Nuckolls, J. Am. Chem. Soc., 2018, 140, 10960–10964 CrossRef CAS PubMed.
- T. B. Gao, J. J. Zhang, R. Q. Yan, D. K. Cao, D. Jiang and D. Ye, Inorg. Chem., 2018, 57, 4310–4316 CrossRef CAS PubMed.
- Y. F. Zhang, B. Gui, R. F. Chen, G. P. Hu, Y. Meng, D. Q. Yuan, M. Zeller and C. Wang, Inorg. Chem., 2018, 57, 2288–2295 CrossRef CAS PubMed.
- B. Kilbas, S. Mirtschin, T. Riis-Johannessen, R. Scopelliti and K. Severin, Inorg. Chem., 2012, 51, 5795–5804 CrossRef CAS PubMed.
- S. Hiraoka, Y. Hisanaga, M. Shiro and M. Shionoya, Angew. Chem., Int. Ed., 2010, 49, 1669–1673 CrossRef CAS PubMed.
- X. C. Sun, Z. Z. Cong, S. Y. Wu, Y. Zhang, M. C. Zhu, X. J. Bai, S. Shanmugaraju and P. S. Mukherjee, J. Mater. Chem. C, 2022, 10, 15516–15523 RSC.
- H. N. Kim, W. X. Ren, J. S. Kim and J. Yoon, Chem. Soc. Rev., 2012, 41, 3210–3244 RSC.
- Y. Lu, Y. X. Deng, Y. J. Lin, Y. F. Han, L. H. Weng, Z. H. Li and G. X. Jin, Chem, 2017, 3, 110–121 CAS.
- D. Tian, Y. Li, R. Y. Chen, Z. Chang, G. Y. Wang and X. H. Bu, J. Mater. Chem. A, 2014, 2, 1465–1470 RSC.
- H. N. Zhang, W. B. Yu, Y. J. Lin and G. X. Jin, Angew. Chem., 2021, 133, 15594–15599 CrossRef.
- N. Jiang, G. Li, W. Che, D. Zhu, Z. Su and M. R. Bryce, J. Mater. Chem. C, 2018, 6, 11287–11291 RSC.
- M. Mastalerz, Angew. Chem., Int. Ed., 2010, 49, 5042–5053 CrossRef CAS PubMed.
- C. Viravaux, O. Oms, A. Dolbecq, E. Nassar, L. Busson, C. Mellot-Draznieks, R. Dessapt, H. Serier-Brault and P. Mialane, J. Mater. Chem. C, 2021, 9, 8323–8328 RSC.
- S. R. Liu, H. T. Chen, H. X. Lv, Q. P. Qin, L. M. Fan and X. T. Zhang, Mater. Today Chem., 2022, 24, 100984–100994 CrossRef CAS.
- H. T. Chen, S. R. Liu, H. X. Lv, Q. P. Qin and X. T. Zhang, ACS Appl. Mater. Interfaces, 2022, 14(16), 18589–18599 CrossRef CAS PubMed.
- T. Zhang, H. T. Chen, S. R. Liu, H. X. Lv, X. T. Zhang and Q. L. Li, ACS Catal., 2021, 11, 14916–14925 CrossRef CAS.
- S. R. Liu, H. G. Chen and X. T. Zhang, ACS Catal., 2022, 12(16), 10373–10383 CrossRef CAS.
- H. Chen, T. Zhang, S. Liu, H. Lu, L. Fan and X. Zhang, Inorg. Chem., 2022, 61(30), 11949–11958 CrossRef CAS PubMed.
|
This journal is © The Royal Society of Chemistry 2023 |
Click here to see how this site uses Cookies. View our privacy policy here.