DOI:
10.1039/D3RA05320B
(Paper)
RSC Adv., 2023,
13, 31720-31727
Semiquantitative and visual detection of ferric ions in real samples using a fluorescent paper-based analytical device constructed with green emitting carbon dots†
Received
5th August 2023
, Accepted 20th October 2023
First published on 30th October 2023
Abstract
A simple and portable paper-based analytical device was developed for visual and semiquantitative detection of ferric ion in real samples using green emitting carbon dots (CDs), which were prepared via microwave method using sodium citrate, urea and sodium hydroxide as raw materials and then loaded on the surface of paper substrate. When Fe3+ exists, the green fluorescence of CDs was quenched and significant color change from green to dark blue were observed, resulting the visual detection of Fe3+ with a minimum distinguishable concentration of 100 μM. By analyzing the intensity changes of green channels of test paper with the help of smartphone, the semiquantitative detection was realized within the range of 100 μM to 1200 μM. The proposed paper-based analytical devices have great application prospects in on site detection of Fe3+ in real samples.
Introduction
As an essential element for the human body, iron plays an important role in maintaining life systems. Iron deficiency may trigger a variety of tissue changes and dysfunctions, e.g., affecting the development and infection resistance of lymphoid tissues, and even worse, lead to iron deficiency anemia and decline in intelligence.1,2 The excessive intake of iron ions will damage important human organs such as heart, liver, and lungs and cause various diseases such as tissue inflammation and cancers.3 Besides that, Fe3+ widely exists in various natural environments such as animals and plants, soil, and river basin water, and in case of high content, it will be enriched in soil and water, which will affect the growth of animals and plants, and may also enter the body through the food chain to endanger human health.4–6 Therefore, the rapid, accurate, and sensitive detection of Fe3+ is still necessary for environmental monitoring and human health protection.
At present, traditional methods for Fe3+ detection with high sensitivity and accuracy, including electrochemical analysis method, atomic absorption spectrometry (AAS), mass spectrometry (MS), liquid chromatography, and inductively coupled plasma mass spectrometry (ICP-MS) have been developed.7–10 However, the application of these methods in metal ion detection is greatly limited because of their expensive detection equipment, complicated detection operation steps, and the need for professional personnel to test. Therefore, the development of simple and fast Fe3+ sensors has become the focus of attention in recent years. Among numerous new detection methods, fluorescence analysis has attracted great attention due to its advantages of simplicity and high sensitivity.11,12
Carbon dots (CDs) have very great potential in the detection field of fluorescent probes by virtue of high fluorescence quantum yield, stable physical and chemical properties, good water dispersibility, green synthesis process, and low costs.13–15 In recent years, based on the quenching effect of metal ions on CDs, a serious of fluorescence sensors have been developed and can be used to detect Fe3+ selectively and sensitively.16–18 However, most of the current Fe3+ fluorescence sensors are aimed at the detection of homogeneous solutions, needing precision instruments such as fluorescence spectrometers and the rapid on site detection of Fe3+ is still a great challenge.
As a new analytical method with paper as the substrate material, paper-based analytical devices (PADs) have attracted extensive attention for its advantages of easily available raw materials, low manufacturing costs, fast detection speed, and simple detection instruments.19,20 Now a series of different optical and electrical detection methods have been developed. Among them, the fluorescence detection method, which integrates the merit of high sensitivity, has been applied in PADs in recent years.21–23 At present, many different types of fluorescent nanoparticles, such as quantum dots,24,25 upconversion nanoparticles,26,27 polymer dots,28 and noble metal nanoclusters,29 have been used to construct paper-based fluorescent sensors and achieved excellent detection results. CDs have also been used as signal markers of paper-based fluorescence sensors to detect metal ions, biological small molecules, proteins and so on.30–32 For the detection of Fe3+, relatively few paper-based fluorescence sensors have been reported,33,34 with the detection performance remaining to be further improved.
Herein, a fluorescent PADs was constructed based on green emitting CDs to realize the detection of Fe3+ in real water samples. CDs were prepared from sodium citrate and urea in sodium hydroxide solution through the microwave method and had bright green emission at 514 nm (Scheme 1a). The fluorescent PADs were fabricated through loading this CDs on the surface of paper substrate using a “drop-coating” method. With the introduction of Fe3+, the fluorescence of CDs was quenched and the PADs showed a color change from green to dark blue. Thus the visual and semiquantitative detection of Fe3+ could be realized by observing the color changes with naked eye and analyzing the intensity changes of green channels with the help of smartphone respectively (Scheme 1b).
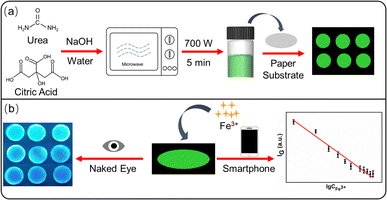 |
| Scheme 1 Schematic illustration of the preparation of paper-based analytical devices on the basis of green emitting carbon dots (a) and the semiquantitative and visual detection of Fe3+ (b). | |
Materials and methods
Materials
Sodium citrate, urea, FeCl3, sodium hydroxide (NaOH) and other inorganic salts were purchased from Sinopharm Chemical Reagent Co., Ltd. (Shanghai, China) and used as received. Dialysis membrane (MWCO = 1000) was bought from Spectrumlabs (USA). Whatman No. 1 filter paper was purchased from Whatman (UK) and used to prepare paper-based analytical devices. The ultrapure water used in this work was produced with water purification system (Milli-Q, Millipore).
Apparatus
TEM characterization of CDs was performed under a Tecnai G2 F 20 transmission electron microscope, the FT-IR spectrum was tested via a Nicolet 5 FT-IR spectrometer (Thermo Fisher, USA), and the zeta potential was measured using a Zetasizer Nano ZS/Mastersizer 3000E laser particle sizer (Malvern Instruments Co., Ltd., UK). In addition, the valence state of surface elements on CDs was determined using a K-ALPHA 0.5 eV X-ray photoelectron spectrometer (Thermo Scientific, USA). The fluorescence spectra, dynamic measurements, and fluorescence lifetimes were measured through an FLS1000 (Edinburgh Instruments, UK) steady-state transient fluorescence spectrometer. The ultraviolet-visible (UV-vis) spectra of materials was acquired using a Shimadzu UV mini-1240 UV-vis spectrometer (Shimadzu Corporation, Japan). The CDs were prepared in a P70D20TL-D4 microwave oven (Galanz, China).
Preparation of green emitting CDs
The preparation of green emitting CDs was appropriately improved on the basis of the reported microwave method.35,36 In brief, 2 g of urea and 1 g of citric acid were added in 20 mL of ultrapure water. Then 0.8 g of NaOH was introduced and stirred for 5 min. The mixed solution was heated for 5 min under the microwave condition of 700 W. After cooling to room temperature, a proper amount of ultrapure water was added for dilution, and the obtained solution was centrifuged at 8000 rpm for 3 min to remove suspended impurities. The supernatant was put into a dialysis membrane (MWCO = 1000) and purified in ultrapure water for 48 h. Finally, the concentration was adjust to 1 mg mL−1.
Detection of Fe3+ in solution
Fe3+ standard solution with gradient concentration was prepared by the stepwise dilution method. 30 μL of CDs solution (1 mg mL−1) and different amounts of Fe3+ standard solution were added into a serious of 2 mL centrifuge tubes and diluted to 600 μL with ultrapure water immediately. The fluorescence spectra were collected subsequently. To investigate the selectivity of CDs toward Fe3+, Fe3+ and other interfering ions with the same concentration were added, and the fluorescence spectra were measured following the same steps.
Detection of Fe3+ on paper-based analytical devices
The circular test papers (6 mm diameter) were prepared using an office puncher. To prepare paper-based analytical devices for the detection of Fe3+, 6 μL of 0.05 mg per mL CDs was added on the surface of paper and then the paper was placed at room temperature for drying. For Fe3+ detection, 6 μL of Fe3+ solution was added on the test paper and let it dry at room temperature. The fluorescence images of the test paper was taken with a smartphone under the irradiation of a 365 nm ultraviolet lamp.
Fe3+ detection in actual samples
The tap water sample used in this work was taken from the laboratory of our research group and filtered with filter membrane (0.22 μm) in advance to remove solid impurities. Then, Fe3+ was detected with the impurities-removed water as the analysis medium according to the same steps as above.
Result and discussion
Characterization of CDs
The morphology of green emitting CDs were characterized through TEM image. As shown in Fig. 1a, the prepared CDs were nearly spherical particles with an average particle size of 2.3 nm (Fig. 1b) and showed well dispersivity in water, which was benefit by the rich functional groups on the surface of CDs. The hydrodynamic diameter of the CDs was measured by dynamic light scattering measurements and the results demonstrated that CDs were uniformly distributed in the range of 37.8–68.0 nm (Fig. S1†). The difference compared to TEM result may be caused by the aggregation of CDs when it was dispersed in water for a long time. As depicted in Fig. S2a,† the zeta potential of CDs was −6.4 mV, indicating that the functional groups with negative charges have a slight advantage. To have a clearer understanding of surface functional groups, the FT-IR spectrum was subsequently measured. As shown in Fig. S2b,† the peak at 3393 cm−1 and 2940 cm−1 was ascribed to O–H and C–H stretching vibration, the peaks at 1567 cm−1 were attributed to C
O stretching vibration, the broad peak between 1465 cm−1 and 1392 cm−1 was assigned to COO− symmetric stretching and C–N stretching vibration, and the peaks at 1103 cm−1 and 874 cm−1 were cause by C–O and N–H bonds, indicating the existence of various functional groups such as carboxyl and amino groups on the surface of CDs. The elemental composition and valence state of CDs were further evaluated by XPS measurements. The four main peaks at 1071.1, 531.1, 399.0, and 285.1 eV in the full-scanning XPS spectrum (Fig. 2a) were the characteristic peaks of Na 1s, O 1s, N 1s, and C 1s, with 6.92%, 27.79%, 0.84%, and 64.45% of each element, respectively. Fig. 2b is the high-resolution XPS spectrum of C 1s, which was fitted to three peaks and assigned to C–C/C
C (284.8 eV), C–N/C–O (286.3 eV), and –COOH (288.1 eV), respectively. For O 1s (Fig. 2c), the three characteristic peaks at 531.0, 532.8, and 535.5 eV were attributed to C
O, O–H, and C–O, respectively. The two peaks at 399.2 eV and 397.7 eV in the high-resolution energy spectrum of N 1s (Fig. 2d) indicated the presence of C–N and N–H bonds. Combining with the N–H bond and C–N bond strength in FTIR spectrum, we speculated that the low nitrogen content was perhaps due to the fact that urea may be mainly used to form the core structure of CDs during the microwave synthesis process at lower power, while with a lower surface content on CDs. Overall, the above FT-IR and XPS results are consistent, indicating the existence of water-soluble functional groups such as carboxyl and amino groups, which exhibited high affinity with Fe3+, ensuring the selective fluorescence response of this green emitting CDs to Fe3+.
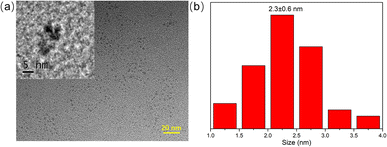 |
| Fig. 1 (a) TEM image of CDs (inset: the HRTEM image of CDs). (b) Size distribution of CDs. | |
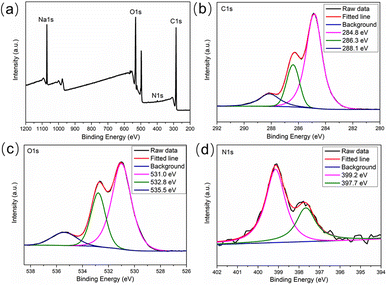 |
| Fig. 2 (a) XPS spectra of CDs and high resolution peaks of (b) C 1s, (c) O 1s and (d) N 1s. | |
Subsequently, UV-vis spectrum and fluorescence spectra were used to characterize the optical properties of CDs. As can be observed in the UV-vis spectrum in Fig. 3a, this green emitting CDs had a characteristic absorption peak at 490 nm. The maximum excitation wavelength of CDs was located at 490 nm (Fig. 3b), which matched well with the characteristic absorption peak. Excited under this wavelength, the maximum emission wavelength of CDs was 514 nm, which was bright green (inset in Fig. 3b). Furthermore, the excitation wavelength independence test of CDs was carried out. As shown in Fig. 3c, the position of the emission peak did not change in the excitation wavelength range of 365–480 nm, indicating that CDs had independent excitation luminescence properties. The photoluminescent quantum yield (QY) of this green emitting CDs was measured and calculated to be 9.3% using of fluorescein in 0.1 M NaOH solution as a reference dye, which exhibits a quantum yield of 95% under 496 nm excitation. Meanwhile, the fluorescence stability of CDs was also measured. As depicted in Fig. 3d, the fluorescence intensity of CDs remained basically unchanged (the reduction degree was less than 3%) under the irradiation of a xenon lamp for 30 min, indicating the good fluorescence stability of the prepared CDs.
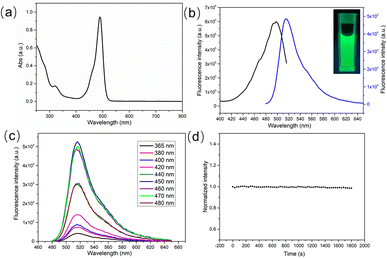 |
| Fig. 3 (a) UV-vis absorption spectrum of CDs. (b) Excitation and emission spectra of CDs. (c) Emission spectra of CDs under different excitation wavelengths. (d) The fluorescence intensity of CDs at 514 nm under continuous irradiation for 30 min. | |
In addition, the luminescent performance of CDs is also affected by the solution environment. Therefore, the influence of pH value on the fluorescence intensity was further studied. As shown in Fig. S3a,† the pH value of the solution did not influence the position of the emission peak but would generate significant effects on the fluorescence intensity. When the pH value grew from 3 to 10, the fluorescence intensity was firstly enhanced and then declined. And the fluorescence intensity reached the maximum value under neutral conditions (Fig. S3b†). Interestingly, CDs also showed satisfying fluorescence emission behavior in pure water.
Feasibility of Fe3+ detection
The specific recognition of signaling molecule to target is the key to construct high-performance sensor. The abundant groups on the surface of CDs indicated that CDs were applicable to fluorescence recognition of Fe3+. In order to explore the feasibility of Fe3+ detection, the selectivity and anti-interference of this green emitting CDs on Fe3+ were first studied. It could be known from Fig. 4 that when Fe3+ with concentration of 200 μM was added, the fluorescence of CDs was significantly quenched. To evaluate the selectivity of CDs towards Fe3+, meanwhile, the influences of other over ten types of interfering metal ions, including Na+, K+, Ca2+, Mg2+, Ba2+, Cu2+, Pb2+, Hg2+, Ni3+, Cr3+, and Fe2+, on the fluorescence signal of CDs were measured. The experimental results revealed that except Fe3+, other interfering cations exerted very minor influences on the fluorescence of CDs (red histogram in Fig. 4), indicating the exclusive fluorescence response to Fe3+. To explore the interference immunity of CDs, the anti-interference experiment of Fe3+ detection was performed under the co-existence with other metal ions. From the blue histogram in Fig. 4, it could be observed that with the presence of 200 μM interfering metal ions, the fluorescence of CDs would still be significantly quenched after the addition of Fe3+. Compared with the sample only added with Fe3+, the fluorescence quenching degree of CDs was basically the same under the co-existence of interfering ions, indicating that the influence of co-existing interfering ions on the detection could be ignored. The influence of ion strength on the fluorescence intensity of CDs is relatively small, and even if the concentration of disruptors reached more than twice that of Fe3+, it can be clearly distinguished (Fig. S4a†). Moreover, the anion interference test were carried out and this green emitting CDs show excellent selectivity and anti-interference ability toward anions such as Cl−, NO3−, and SO42− (Fig. S4b†). The above results indicate that CDs can selectively recognize Fe3+ and are expected to be used in the determination of Fe3+ in practical samples. The excellent selectivity of this green emitting CDs towards Fe3+ is due to their rich functional groups on the surface, such as hydroxyl, carboxyl and amino groups, which was confirmed by FTIR spectra in Fig. S2b.† These functional groups show high affinity to Fe3+ and can form a complex with the Fe3+, thus ensuring the selectivity of this CDs-based sensor.
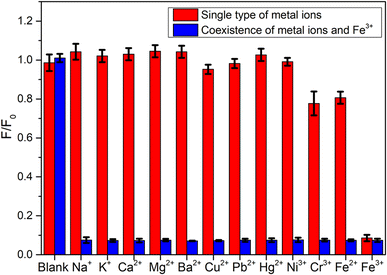 |
| Fig. 4 The relative fluorescence intensity of CDs with the addition of single type of metal ions (Fe3+ and different kind of disruptors) and the coexistence of metal ion disruptors and Fe3+. All of the concentrations of metal ions were 200 μM. | |
Condition optimization
Since Fe3+ could selectively quench the fluorescence of CDs, Fe3+ could be detected based on the CDs fluorescence quenching method. To achieve optimal analytical performance, the experimental conditions were optimized. Considering that the fluorescence quenching degree of Fe3+ on CDs may vary under different pH conditions, this condition was optimized firstly. As shown in Fig. S5a,† Fe3+ exhibited the highest quenching degree for the fluorescence of CDs in the neutral environment pH 6 and pH 7. It is noteworthy that in pure water, Fe3+ also showed very excellent quenching performance for the fluorescence of CDs. For the convenience of operation, the subsequent analysis and detection process was carried out in pure water. Subsequently, the influence of the reaction time between CDs and Fe3+ on the fluorescence intensity was studied. As can be seen from Fig. S5b,† the fluorescence of CDs was quenched substantially as soon as Fe3+ was added and then kept basically unchanged with the extension of the reaction time. To obtain stable fluorescence signals, the reaction time was selected as 5 min.
Fe3+ detection in aqueous solutions
Under the above optimized conditions, green emitting CDs were applied to Fe3+ detection. As shown in Fig. 5a, with the concentration of Fe3+ increased from 0 μM to 400 μM, the fluorescence intensity of CDs gradually decreased. Under the low concentration of Fe3+ (<60 μM), the fluorescence quenching degree of CDs was relative low. When the concentration of Fe3+ was over 60 μM, the fluorescence quenching efficiency was significantly improved and reached the maximum under the Fe3+ concentration of 200 μM. As the concentration of Fe3+ continued to increase the fluorescence quenching efficiency was basically maintained (Fig. 5b). A good linear relationship was observed in the range of 60–200 μM (inset of Fig. 5b). The linear curve equation was (F0 − F)/F0 = 0.0069[Fe3+] − 0.4609, and the correlation coefficient was R = 0.9965. According to 3δ/K (δ is the standard deviation of blank samples for 9 times and K is the slope of the linear equation), the LOD of Fe3+ was about 9.6 μM.
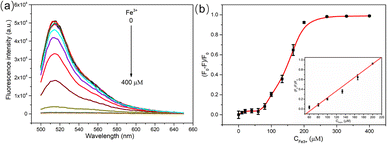 |
| Fig. 5 (a) Fluorescence spectra of CDs (0.05 mg mL−1) with the addition of different amounts of Fe3+ (0, 10, 20, 40, 60, 80, 100, 133, 167, 200, 270, 330, 400 μM). (b) The quenching efficiency versus the concentration of Fe3+ (inset: the linear relationship between the quenching efficiency and Fe3+ concentration within the range of 60–200 μM). | |
Quenching mechanism study
The fluorescence quenching modes of CDs include dynamic quenching, static quenching, fluorescence resonance energy transfer (FRET), and internal filtration effect (IFE).37–39 Static quenching is trigged by the change of surface group structure, which leads to the decrease of fluorescence intensity, without any obvious change of fluorescence lifetime. For dynamic quenching, the fluorophores in solution collide with the quencher, which results in energy loss and the reduction of fluorescence intensity, which is most significantly characterized by the obviously shortened fluorescence lifetime of fluorophores. When there is spectral overlap between the absorption spectrum of the quencher and the excitation/emission spectrum of the fluorophore, fluorescence quenching may also be caused by IFE or FRET. The difference between them is that in the FRET process, the fluorescence lifetime of fluorophores is significantly shortened, while IFE does not affect the lifetime.
Given the above differences, the fluorescence quenching mechanism was analyzed through UV-vis spectra and fluorescence lifetime measurements. Fe3+ had a characteristic absorption peak in the range of 250–400 nm, but no absorption in the range of 400–800 nm (Fig. 6a), indicating no spectral overlap between CDs and Fe3+, so FRET and IFE could be excluded. Given that static quenching forms non-fluorescent ground state compounds and the absorption spectrum of fluorophores will change, while the absorption spectrum does not change during dynamic quenching, as this process only affects the excited state of the fluorescent group.37 Therefore, the UV-vis spectra before and after the addition of Fe3+ were compared carefully (Fig. 6a). The results showed that after Fe3+ was added, the absorption spectrum of CDs–Fe3+ experienced shape change. The absorbance of the CDs–Fe3+ was not a summation of CDs and Fe3+ absorbance values, and the absorbance within 250–400 nm was significantly higher than the sum result, indicating that CDs and Fe3+ formed new non-fluorescent ground state complexes.4,16,17 To further ascertain this speculation, zeta potential and FT-IR spectra of CDs before and after the introduction of Fe3+ were measured. As shown in Fig. S6a,† the zeta potential of CDs changed from −10.8 mV to 23.0 mV after been interacted with Fe3+, suggesting that Fe3+ and N–CDs surface groups might be coordinated owing to intermolecular electrostatic interactions. Compared to the FTIR spectrum of CDs before the addition of Fe3+, that after adding Fe3+ showed obvious changes in the intensity and slight shifts in the position of 3385 cm−1, 1427 cm−1 and 878 cm−1 (Fig. S6b†), which were assigned to O–H, C
O, and N–H groups respectively. This observation might be attributed to the presence of polar functional groups, including hydroxyl, amine and carboxylic on the surface of CDs, which show strong affinity toward Fe3+ and form CDs–Fe3+ non-fluorescent complexes.40 Subsequently, the fluorescence lifetime of CDs before and after the introduction of Fe3+ was measured. Fig. 6b shows the fluorescence attenuation curves before and after adding Fe3+ into CDs, and almost no differences were observed. The calculated average fluorescence lifetime was respectively 4.72 and 4.71 ns (Table S1†), manifesting that the fluorescence life of CDs was not affected by Fe3+. The above experimental results showed that Fe3+ could quench the fluorescence of CDs via static fluorescence quenching.
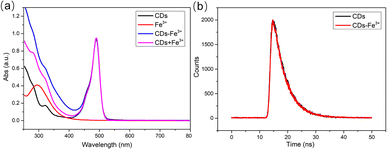 |
| Fig. 6 (a) UV-vis absorption spectra of CDs, Fe3+, CDs–Fe3+, and CDs + Fe3+. (b) Fluorescence lifetime of CDs and CDs–Fe3+. | |
Fe3+ detection on paper-based analytical devices
The circular filter paper was first prepared with the help of office puncher, and the paper-based analytical device for Fe3+ detection was prepared by loading appropriate amount of CDs on the surface of paper. Under the irradiation of 365 nm ultraviolet lamp, this CDs-based PADs emitted bright green fluorescence (Fig. 7a). With the increase of Fe3+ concentration, the green fluorescence of CDs at 514 nm was gradually quenched, causing the color of the test paper to darken, gradually changing from bright green to dark blue. Based on this, visualization detection of Fe3+ can be carried out, and the naked eye distinguishable concentration reached 100 μM. For quantitative analysis, the intensity of green channel of the fluorescent image was read (expressed by IG) via color recognition picker APP. As depicted in Fig. 7b, the IG value was gradually reduced as the Fe3+ concentration increased and a good linear correlation between the IG value and the logarithm of Fe3+ concentration was observed in the range of 100 μM to 1200 μM (Fig. 7c), so the quantitative analysis of Fe3+ could be conducted. Compared to other paper-based fluorescence sensors reported for Fe3+ detection, this CDs-based PADs exhibited an ultrawide linear range (more than 2 orders of magnitude), providing assurance for the detection of Fe3+ in actual samples (Table 1).
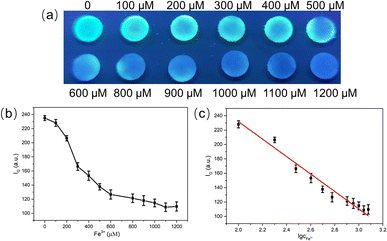 |
| Fig. 7 (a) The images of PADs under 365 nm UV lamp with the introduction of different concentrations of Fe3+. (b) The intensity of green channel of the fluorescent images versus the concentration of Fe3+. (c) The linear relationship between the intensity of green channel and the logarithm of Fe3+ concentration within the range of 100–1200 μM. | |
Table 1 Comparison of different paper-based fluorescence sensors reported for Fe3+ detection
Probe |
Linear range (μM) |
LOD (μM) |
Actual sample testing |
Ref. |
Nitrogen-doped carbon quantum dots |
5 × 10−3 to 1 × 10−1 |
9.4 × 10−4 |
None |
41 |
CdTe@SiO2 QDs with rhodamine derivative |
1–30 |
0.5 |
None |
42 |
Rhodamine based probe RhBUEA |
0–80 |
1.4 |
Tap water |
33 |
Green emitting CDs |
100–1200 |
100 |
Tap water |
This work |
To investigate the selectivity of the constructed PADs to Fe3+, different kinds of metal ions (500 μM) were introduced. It could be seen from Fig. S7† that the color of the PADs remained green after adding other ions, while the PADs added with Fe3+ turned blue obviously, indicating that this PADs had good selectivity to Fe3+ and could distinguish it from other interfering metal ions.
Fe3+ detection in actual samples
To further investigate the practicability of this strategy, the constructed PADs were used to detect Fe3+ in tap water. As can be observed from Fig. 8a, when tap water was directly added into the PADs, no obvious color change could be observed. To realize Fe3+ detection in tap water using PADs, Fe3+ with different concentrations (200, 400, 800 μM) was manually introduced into tap water. When the concentration of Fe3+ reached 200 μM, the color change of the PADs could be distinguished by naked eyes, and its color was gradually deepened with the increase of the Fe3+ concentration. Furthermore, the recovery rate experiment were carried out by extracting the green channel intensity of images through smartphones. As shown in Table S2,† acceptable recovery rates ranging from 97.1% to 105.3% with RSDs less than 5% were achieved, indicating the reliability of this PADs in practical applications. In tap water samples, as depicted in Fig. 8b, this PADs could distinguish Fe3+ with the concentration of 200 μM from other metal ions. Considering the possible co-existence of multiple metal ions in actual water samples, a mixed ionic solution was prepared to simulate the actual water sample. It could be observed from Fig. 8c that when Fe3+ was contained in the mixed solution, the color of the PADs experienced significant changes, which is clearly different from the situation where Fe3+ does not exist. Above as, the constructed CDs-based PADs has very great feasibility and application potential in the on-site detection of Fe3+ in actual water samples.
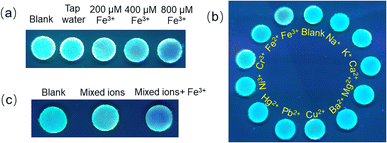 |
| Fig. 8 (a) The images of PADs under 365 nm UV lamp with the addition of different concentrations of Fe3+ (0, 200, 400, 800 μM) in tap water. (b) The images of PADs with the introduction of 200 μM Fe3+ and different kinds of interfering ions in tap water. (c) The images of PADs with the addition of mixed metal ions and their coexistence with Fe3+. The concentration of Fe3+ and other interfering ions are all 500 μM. | |
Conclusion
A green emitting CDs modified paper-based analytical devices integrated with smartphone platform was developed for visual and semiquantitative detection of Fe3+. CDs, which prepared through a facile and high-efficiency microwave method, had intense green light emission at 514 nm and showed specific signal response to Fe3+. Dropped Fe3+ on fluorescent test paper would cause fluorescence quenching of CDs, resulting in the color changing from green to dark blue. The visual and semiquantitative detection of Fe3+ could be realized according to the color changes of the PADs under UV light with a linear range of 100 μM to 1200 μM and a naked eye distinguishable concentration of 100 μM. The CDs-based PADs with smartphone application can render a simple, visual detection of Fe3+ in real water samples. The preparation method of CDs proposed in this study is simple and fast and characterized by environment-friendliness of raw materials, high luminescent intensity, fast response and selectivity to Fe3+, and strong interference immunity, providing a guarantee for the excellent detection performance of PADs. Furthermore, the established fluorescent PADs, which are simple and portable, can realize Fe3+ detection in water samples, showing considerable feasibility and application potential in the fast field detection of Fe3+.
Author contributions
Mengyuan He: conceptualization, funding acquisition, methodology, project administration, investigation, writing – review & editing. Yu Xiao: validation, investigation, data curation, data analysis and discussion. Yuanhang Wei: investigation, data curation, and data analysis and discussion. Bo Zheng: funding acquisition, project administration, writing – original draft.
Conflicts of interest
There are no conflicts to declare.
Acknowledgements
This work was supported by the National Natural Science Foundation of China (No. 21804117), the Key Scientific and Technological Project of Henan Province (232102310151), the Youth Fund Project of Xinyang Normal University (2022-QN-039), and the Nanhu Scholars Program for Young Scholars of Xinyang Normal University.
Notes and references
- M. Y. Hsu, E. Mina, A. Roetto and P. E. Porporato, Cells, 2020, 9, 2591 CrossRef CAS PubMed.
- L. Guo, T. Tang, L. Hu, M. Yang and X. Chen, Sens. Actuators, B, 2017, 241, 773–778 CrossRef CAS.
- X. Zhou, G. Zhao, X. Tan, X. Qian, T. Zhang, J. Gui, L. Yang and X. Xie, Microchim. Acta, 2019, 186, 67 CrossRef PubMed.
- Y. Zhou, G. Chen, C. Ma, J. Gu, T. Yang, L. Li, H. Gao, Y. Xiong, Y. Wu, C. Zhu, H. Wu, W. Yin, A. Hu, X. Qiu, W. Guan and W. Zhang, Spectrochim. Acta, Part A, 2023, 293, 122414 CrossRef CAS PubMed.
- R. Atchudan, T. N. J. I. Edison, K. R. Aseer, S. Perumal, N. Karthik and Y. R. Lee, Biosens. Bioelectron., 2018, 99, 303–311 CrossRef CAS PubMed.
- S. G. Shi, Y. X. Zhang, Y. Y. Li and J. X. Chi, Au@MnO Core-shell Nanomaterials for Tumor Diagnosis and Therapy, J. Xinyang Norm. Univ., Nat. Sci. Ed., 2021, 34(4), 624–629 Search PubMed.
- Y. Liu, W. Duan, W. Song, J. Liu, C. Ren, J. Wu, D. Liu and H. Chen, ACS Appl. Mater. Interfaces, 2017, 9, 12663–12672 CrossRef CAS PubMed.
- J. Nandre, S. Patil, V. Patil, F. Yu, L. Chen, S. Sahoo, T. Prior, C. Redshaw, P. Mahulikar and U. Patil, Biosens. Bioelectron., 2014, 61, 612–617 CrossRef CAS PubMed.
- L. S. Huang and K. C. Lin, Spectrochim. Acta, Part B, 2001, 56, 123–128 CrossRef.
- D. V. Biller and K. W. Bruland, Mar. Chem., 2012, 130–131, 12–20 CrossRef CAS.
- H. B. Wang, A. L. Mao, H. D. Zhang and H. Y. Bai, DNA-Au Nanoclusters-Based Novel Fluorescent Method for Sensitive Detection of Trypsin, J. Xinyang Norm. Univ., Nat. Sci. Ed., 2021, 34(1), 115–118 Search PubMed.
- H. B. Wang, B. B. Tao, N. N. Wu, A. L. Mao and H. D. Zhang, DNA-Au Nanoclusters Inner Filter Effect Induced Fluorescence Quenching for Sensitive Detection of 4-Nitrophenol, J. Xinyang Norm. Univ., Nat. Sci. Ed., 2022, 35(3), 464–468 Search PubMed.
- C. Ji, Y. Zhou, R. M. Leblanc and Z. Peng, ACS Sens., 2020, 5, 2724–2741 CrossRef CAS PubMed.
- Y. Wang, K. Jiang, J. Zhu, L. Zhang and H. Lin, Chem. Commun., 2015, 51, 12748–12751 RSC.
- M. He, B. Zheng, N. Shang, Y. Xiao, Y. Wei and X. Hu, Microchem. J., 2023, 190, 108593 CrossRef CAS.
- M. Picard, S. Thakur, M. Misra and A. K. Mohanty, RSC Adv., 2019, 9, 8628–8637 RSC.
- M. Amiri, A. M. H. Shabani, S. Dadfarnia, N. Shokoufi, B. Hajipour-Verdom and S. Sadjadi, Microchim. Acta, 2020, 187, 562 CrossRef CAS PubMed.
- Y. Liang, L. Xu, K. Tang, Y. Guan, T. Wang, H. Wang and W. W. Yu, Dyes Pigm., 2020, 178, 108358 CrossRef CAS.
- M. He, N. Shang, Q. Zhu and J. Xu, Anal. Chim. Acta, 2021, 1143, 93–100 CrossRef CAS PubMed.
- M. Wu, Q. Lai, Q. Ju, L. Li, H.-D. Yu and W. Huang, Biosens. Bioelectron., 2018, 102, 256–266 CrossRef CAS PubMed.
- F. Wang, W. Li, J. Wang, J. Ren and X. Qu, Chem. Commun., 2015, 51, 11630–11633 RSC.
- Z. Luo, T. Lv, K. Zhu, Y. Li, L. Wang, J. J. Gooding, G. Liu and B. Liu, Angew. Chem., Int. Ed., 2020, 59, 3131–3136 CrossRef CAS PubMed.
- L. A. Pradela-Filho, W. B. Veloso, I. V. S. Arantes, J. L. M. Gongoni, D. M. de Farias, D. A. G. Araujo and T. R. L. C. Paixão, Microchim. Acta, 2023, 190, 179 CrossRef CAS PubMed.
- Y. Zhou, X. Huang, C. Liu, R. Zhang, X. Gu, G. Guan, C. Jiang, L. Zhang, S. Du, B. Liu, M.-Y. Han and Z. Zhang, Anal. Chem., 2016, 88, 6105–6109 CrossRef CAS PubMed.
- X. Yuan, J. Zhang, H. Yang, Q. Yang, L. Li, M. Zhang and K. Huang, Sens. Actuators, B, 2022, 372, 132686 CrossRef CAS.
- Q. Mei, H. Jing, Y. Li, W. Yisibashaer, J. Chen, B. Nan Li and Y. Zhang, Biosens. Bioelectron., 2016, 75, 427–432 CrossRef CAS PubMed.
- K. Kaur, B. K. Sahu, K. Swami, M. Chandel, A. Gupta, L.-H. Zhu, J. P. Youngblood, S. Kanagarajan and V. Shanmugam, ACS Appl. Mater. Interfaces, 2022, 14, 27507–27514 CrossRef CAS PubMed.
- C. Y. Shi, N. Deng, J. J. Liang, K. N. Zhou, Q. Q. Fu and Y. Tang, Anal. Chim. Acta, 2015, 854, 202–208 CrossRef CAS PubMed.
- N. Cao, J. Xu, H. Zhou, Y. Zhao, J. Xu, J. Li and S. Zhang, Microchem. J., 2020, 159, 105406 CrossRef CAS.
- Y. Kim, G. Jang and T. S. Lee, ACS Appl. Mater. Interfaces, 2015, 7, 15649–15657 CrossRef CAS PubMed.
- A. Gupta, N. C. Verma, S. Khan, S. Tiwari, A. Chaudhary and C. K. Nandi, Sens. Actuators, B, 2016, 232, 107–114 CrossRef CAS.
- I. Ortiz-Gomez, M. Ortega-Muñoz, A. Marín-Sánchez, I. de Orbe-Payá, F. Hernandez-Mateo, L. F. Capitan-Vallvey, F. Santoyo-Gonzalez and A. Salinas-Castillo, Microchim. Acta, 2020, 187, 421 CrossRef CAS PubMed.
- X. Liu, Z. Chen, R. Gao, C. Kan and J. Xu, J. Environ. Chem. Eng., 2022, 10, 107650 CrossRef CAS.
- Y. Zhang, L. Gao, S. Ma and T. Hu, Spectrochim. Acta, Part A, 2022, 267, 120525 CrossRef CAS PubMed.
- S. Qu, X. Wang, Q. Lu, X. Liu and L. Wang, Angew. Chem., Int. Ed., 2012, 51, 12215–12218 CrossRef CAS PubMed.
- J. Y. Wei, Q. Lou, J. H. Zang, Z. Y. Liu, Y. L. Ye, C. L. Shen, W. B. Zhao, L. Dong and C. X. Shan, Adv. Opt. Mater., 2020, 8, 1901938 CrossRef CAS.
- F. Zu, F. Yan, Z. Bai, J. Xu, Y. Wang, Y. Huang and X. Zhou, Microchim. Acta, 2017, 184, 1899–1914 CrossRef CAS.
- X. Mu, M. Wu, B. Zhang, X. Liu, S. Xu, Y. Huang, X. Wang, D. Song, P. Ma and Y. Sun, Talanta, 2021, 221, 121463 CrossRef CAS PubMed.
- M. He, N. Shang, B. Zheng and G. Yue, RSC Adv., 2021, 11, 21137–21144 RSC.
- X. B. Cui, Y. L. Wang, J. Liu, Q. Y. Yang, B. Zhang, Y. Gao, Y. Wang and G. Y. Lu, Sens. Actuators, B, 2017, 242, 1272–1280 CrossRef CAS.
- J. Korram, P. Koyande, S. Mehetre and S. N. Sawant, ACS Omega, 2023, 8, 31410–31418 CrossRef CAS PubMed.
- Y. S. Kuang, X. Wang, X. K. Tian, C. Yang, Y. Li and Y. L. Nie, J. Photochem. Photobiol., A, 2019, 372, 140–146 CrossRef CAS.
Footnote |
† Electronic supplementary information (ESI) available: Partial experiments, zeta potential and FT-IR spectrum of CDs, condition optimization, selectivity of PADs and the fitting results of fluorescence lifetime. See DOI: https://doi.org/10.1039/d3ra05320b |
|
This journal is © The Royal Society of Chemistry 2023 |
Click here to see how this site uses Cookies. View our privacy policy here.