DOI:
10.1039/D3RA06427A
(Paper)
RSC Adv., 2023,
13, 31092-31100
Polyether-based waterborne synergists: effect of polymer topologies on pigment dispersion†
Received
21st September 2023
, Accepted 18th October 2023
First published on 24th October 2023
Abstract
Control of polymer topologies is essential to determine their unique physical properties and potential applications. The polymer topologies can have a critical effect on pigment dispersion owing to their unique architectures; however, studies using polymer topologies on pigment dispersion in aqueous systems are scarce. Thus, this study proposes various topologies of polyether-based waterborne synergists, such as linear, hyperbranched, and branched cyclic structures. Specifically, we applied branched types of polyglycidols (PGs) as a synergist to provide polymer topology-dependent dispersibility for the surface-modification of Red 170 particles through adsorption and steric hindrance. The topology-controlled PG synergists (PGSs) were successfully prepared by post-polymerization modification with phthalimide and benzoyl groups. Particularly, the branched types of PGSs, branched cyclic PGS (bc-PGS), and hyperbranched PGS (hb-PGS) exhibited improved dispersibility through adsorption on top of the pigment, interaction between dispersant (BYK 190) and pigment, and steric effect. Surprisingly, hb-PGS conferred the Red 170 pigment particles with superior storage stability than that of bc-PGS despite their similar structural features. This study suggests the widespread potential application of PGSs as waterborne synergists for various dispersion applications.
Introduction
Dispersion technologies for organic and inorganic pigments used in various industries such as digital printing, paints, coating, cosmetics, and display have been extensively developed. This is because the dispersibility of pigments in a solution significantly affects optical densities, shape spectral properties, transmittance, and color resolution.1–5 In particular, the design of a high-performance dispersant system should consider numerous factors such as chemical structure, hydrophile–lipophile balance, molecular weight, and molecular-weight distribution for providing a stable dispersion with uniform particle size.5–10 Additionally, dispersants have received significant attention in dispersion applications, because they can enhance the dispersing ability and durability upon exposure, facilitating higher stability of pigment dispersions. However, obtaining high efficiency in applications by employing dispersing agents remains challenging because of incomplete dispersion and difficulty in complete particle separation. In addition, these limitations lead to uneven coloring, thereby further limiting the advantage of the fully optimized effect.11,12 To overcome the challenges associated with dispersants in pigment-based products, modulating pigment aggregation forces is essential to maximize the dispersing effect on pigment performance.
Numerous approaches to design the dispersing ability of dispersants using specific surfactants, functionalized polymers, and mineral oxides have been reported.13,14 Among them, the use of synergists to reinforce traditional dispersant-based methods has recently become a focus in dispersion systems. Specifically, the use of synergists offers interesting opportunities not only for forming strong adsorption of dispersants on pigment surface but also for stabilizing pigment particles by reducing interparticle flocculation through the steric hindrance effect.15–19 Many of these synergists have been designed based on chemical structures similar to the targeted pigment molecules. For example, in a phthalocyanine-based dispersion, the core part in the synergist consists of the phthalocyanine molecular structure to facilitate the adsorption of the synergist on the pigment surface.16 In addition, the edge side of this synergist modified with functional groups, such as primary amine or carboxylic acid, is effectively utilized in binding to anchoring groups of dispersants via strong hydrogen bonding. Moreover, Kelley et al. reported that acid-functionalized synergists can maintain a high dispersibility of organic pigment particles even in propylene glycol methyl ether acetate-based eco-friendly solvent systems.18 Although this synergist-based approach provides an optimized interaction with the targeted pigment and excellent dispersing performance, only a few studies using synergists for the preparation of pigment dispersion in aqueous systems have been reported as a result of the limited modification of pigment-based designed synergists. Moreover, to utilize organic pigments in aqueous systems, the organic synergist should essentially include a hydrophilic part or polar features.19 This synergist-based dispersing approach for aqueous systems is thus highly challenging because dispersibility via various types of dispersants and the corresponding properties, such as storage stability, are effectively controllable through the incorporation of synergists with functionalities for interacting between pigment particles and dispersants.
In the past few decades, the control of polymer topologies for managing their unique functions and physical properties has attracted attention.20,21 As representative examples, dendrimers and hyperbranched polymers have been investigated owing to their interesting physical properties such as excellent solubility, low solution viscosity, and modified rheological properties, which originate from their branched multifunctional structures compared with linear structures.22–25 Hawker et al. reported the polymeric architectural effects on a TiO2 dispersion using diverse branched poly(acrylic acid) derivatives in comparison to linear polymers in an aqueous system.26 In addition, Storey et al. demonstrated three types of polyisobutylene succinimide analog dispersants (linear, grafted, and comb type) for carbon black dispersion.27 Compared with linear dispersants in the organic system, the comb- and grafted-type dispersants exhibited greater affinities for adsorption but decreased packing efficiencies. Despite the successful improvement of the dispersion performance via controlling polymer topologies, most of these investigations have been limited to linear polymer systems;28–30 thus, we aim to seek further tuning of the dispersion performance via precise control of polymeric topology.
In our previous work, hyperbranched polyglycidols (hb-PGs) and branched cyclic PGs (bc-PGs) were successfully synthesized via metal-free ring-opening polymerization of glycidol using frustrated Lewis pairs along with linear PGs (lin-PGs) having a similar degree of polymerization (DPn) as control (Scheme 1a)31,32 We found that precisely controlled polymer topologies are important factors in determining their unique properties, such as enhanced adhesion performance via cooperative hydrogen bonding between polymer chains with excellent biocompatibility and enhanced self-healing efficiency via different polymer network mesh space.33,34 Evidently, a highly flexible polyether backbone with multiple functional hydroxyl groups would be suitable as a polymer-based synergist in aqueous dispersion system because its strong hydrophilic feature can provide organic pigment particles with high aqueous solubility. Additionally, numerous hydroxy groups in pendants can allow for variable functionalization via post-polymerization modification, such as the introduction of hydrophobic groups or anchor moieties (Scheme 1b). Herein, we present the potential application of topology-controlled polyglycidol-based waterborne synergists (PGSs) with different anchor groups on dispersion performance. The Red 170 pigment iswidely used in various industries, and BYK 190 as a polymeric dispersant mixed with styrene and maleic acid has been applied to our aqueous dispersion system. On the basis of these topology-controlled PGSs, we establish their topological effect on pigment dispersibility and storage stability. Therefore, this study on pigment dispersion by applying topology-controlled polyethers can demonstrate their potential as synergists in the field of dispersion and further show the topological effect of polymers on dispersibility.
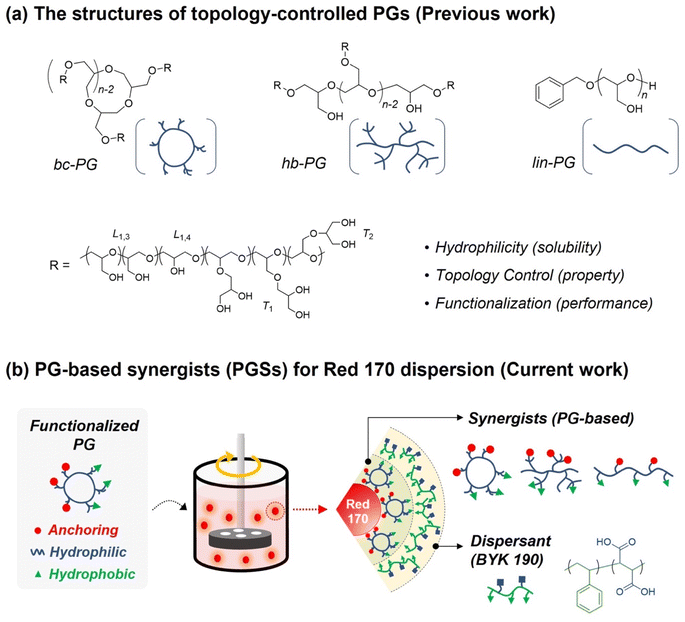 |
| Scheme 1 Research scheme of Red 170 dispersion based on polyether-based synergists. (a) The molecular structure of topology-controlled polyglycidols (PGs), and (b) the pigment dispersion strategy using functionalized PG-based synergists (PGSs) are described in this scheme. | |
Experimental
Materials
Glycine, 2,3-naphthalenedicarboxylic anhydride, dimethylformamide (DMF) (Sigma-Aldrich; ≥99.8%), ethanolamine (Sigma-Aldrich; ≥99.0%), acrylic acid (Sigma-Aldrich), N-(3-dimethylaminopropyl)-N′-ethylcarbodiimide hydrochloride (TCI; >98.0%), 4-dimethylaminopyridine (DMA) (TCI; >99.0%), methylene chloride (Sigma-Aldrich, ≥99.8%), 1,8-naphthalic anhydride (TCI; >98.0%), triethylamine (Sigma-Aldrich; ≥99%), benzoyl chloride (Alfa Aesar; 99+%), 1-ethyl-3-(3-dimethylaminopropyl)carbondiimide (EDCI) (TCI; >98.0%), diethyl ether (Burdick & Jackson, ACS), N-phthaloylglycine (A1) (TCI; >98.0%), 9-oxoacridine-10-acetic acid (A4) (TCI; >98.0%), and anthraquinone-2-carboxylic acid (A5) (TCI; >99.0%) were used as received without purification. Toluene (Sigma-Aldrich; >99%) was purified by column chromatography prior to use. The molecular sieves (4 Å) were dried at 300 °C using a heating gun under a reduced pressure for 30 min prior to use. Tetralin (Sigma-Aldrich; 99%), tris(pentafluorophenyl)borane (B(C6F5)3) (Sigma-Aldrich; 95%), glycidol (Sigma-Aldrich; 96%), and tributylamine (TBA) (Sigma-Aldrich; ≥98.5%) were dried overnight over calcium hydride and purified by distillation. Dimethyl sulfoxide (DMSO, SUNSEI; 99%), 3,4-dihydro-2H-pyran (Sigma-Aldrich; 97%), p-toluenesulfonic acid (Sigma-Aldrich; ≥98.5%), sodium bicarbonate (DUKSAN; 99%), sodium sulfate, ethyl acetate (Sigma-Aldrich, ≥99.5%), hexane (Sigma-Aldrich; ≥99.5%), phosphazene base P4-t-Bu solution (t-BuP4) (Sigma-Aldrich; ∼0.8 M in hexane), benzyl alcohol (Sigma-Aldrich; 99.8%), benzoic acid (Sigma-Aldrich; 99.5%), tetrahydrofuran (THF; ≥99.9%, inhibitor-free), HCl (Sigma-Aldrich, ACS reagent; 37%), MeOH (Sigma-Aldrich; 99%), and potassium carbonate (Sigma-Aldrich, ACS reagent; ≥99.0%) were used as received.
Preparation of topology-controlled poly(glycidol)s (PG)
Branched cyclic (bc-PG), hyperbrached (hb-PG), and linear polyglycidols (lin-PG) were synthesized according to procedures previously reported in the literature.31,32,35
General procedure for the preparation of bc-PG-BzAn synergists
The post-polymerization modification of bc-PG was performed using syringe technique under dry argon in a baked round-bottom flask equipped with a three-way stopcock. A typical procedure for preparing polymeric synergists using bc-PG is described as follows. For example, for bc-PG-BzA1, 4.00 g of bc-PG (54.0 mmol for total hydroxyl groups) was placed in a round-bottom flask (250 mL) and completely dissolved in 80 mL of DMF. After the addition of 1.51 mL of triethylamine (11.0 mmol), the mixture was cooled to 0 °C, and 1.26 mL of benzoyl chloride (11.0 mmol) was then slowly added to the mixture. The mixture was stirred at room temperature for 24 h. After the subsequent reaction was completed, 1.04 g of EDCI (5.40 mmol) and 0.330 g of DMAP (2.70 mmol) were introduced to the flask, and 1.11 g of N-phthaloylglycine (5.4 mmol) (A1) was subsequently added. After the completion of the reaction, the solution was precipitated by cold diethyl ether several times to obtain the highly pure product. The resultant bc-PG-BzA1 was vacuum-dried and characterized by size-exclusion chromatography (SEC) (bc-PG-BzA1 in Table 1, Mn = 1,070, Mw/Mn = 2.40).
Table 1 Characterizations of bc-PGSs with different anchor groups in this study
Entry |
Synergist |
Mna |
Mw/Mna |
Tgb |
Ratio (–OH/BzCl/anchor) |
The number-averaged molecular weight (Mn) and molecular weight distribution (Mw/Mn) of the synergists were determined by size-exclusion chromatography (SEC) with PEO standard for calibration in DMF. Glass transition temperature (Tg) was analyzed by differential scanning calorimetry (DSC) at a heating rate of 10 °C min−1 under nitrogen atmosphere. The molecular weight of bc-PG-BzA2 synergist was not measured because this product was not dissolved in DMF as an eluent used in SEC analysis. |
0 |
bc-PG |
1350 |
2.1 |
−43 |
10/0/0 |
1 |
bc-PG-BzA1 |
1070 |
2.4 |
−20 |
7/2/1 |
2 |
bc-PG-BzA2 |
n/dc |
n/dc |
−22 |
7/2/1 |
3 |
bc-PG-BzA3 |
970 |
2.9 |
−20 |
7/2/1 |
4 |
bc-PG-BzA4 |
960 |
2.7 |
−11 |
7/2/1 |
5 |
bc-PG-BzA5 |
870 |
2.8 |
−22 |
7/2/1 |
Synthesis of the hb-PG-BzA1 synergist
The post-polymerization modification of hb-PG was performed using syringe technique under dry argon in baked round-bottom flask equipped with a three-way stopcock. A typical procedure for preparing polymeric synergists using hb-PG is described as follows. For example, for hb-PG-BzA1, 4.00 g of hb-PG (54.0 mmol for total hydroxyl groups) was placed in a round-bottom flask (250 mL) and completely dissolved in 80 mL of DMF. After the addition of 1.51 mL of triethylamine (11.0 mmol), the mixture was cooled to 0 °C, and 1.26 mL of benzoyl chloride (11.0 mmol) was slowly added to the mixture. The mixture was stirred at room temperature for 24 h. After reaction completion, 1.04 g of EDCI (5.40 mmol) and 0.330 g of DMAP (2.70 mmol) were introduced to the flask and 1.11 g of N-phthaloylglycine (5.4 mmol) (A1) was subsequently added. After the subsequent reaction was completed, the solution was precipitated by cold diethyl ether several times to obtain the highly pure product. The resultant hb-PG-BzA1 was vacuum-dried and characterized by SEC (hb-PG-BzA1 in Table S1,† Mn = 570, Mw/Mn = 1.40).
Synthesis of the lin-PG-BzA1 synergist
The post-polymerization modification of lin-PG was performed using syringe technique under dry argon in a baked round-bottom flask equipped with a three-way stopcock. A typical procedure for preparing polymeric synergists using lin-PG is described as follows. For example, lin-PG-BzA1, 4.00 g of lin-PG (54.0 mmol for total hydroxyl groups) was placed in a round-bottom flask (250 mL) and completely dissolved in 80 mL of DMF. After the addition of 1.51 mL of triethylamine (11.0 mmol), the mixture was cooled to 0 °C, and 1.26 mL of benzoyl chloride (11.0 mmol) was slowly added to the mixture. The mixture was stirred at room temperature for 24 h. After the reaction was completed, 1.04 g of EDCI (5.40 mmol) and 0.330 g of DMAP (2.70 mmol) were introduced to the flask, and 1.11 g of N-phthaloylglycine (5.4 mmol) (A1) was added. After the subsequent reaction was completed, the solution was precipitated by cold diethyl ether several times to obtain the highly pure product. The resultant lin-PG-BzA1 was vacuum-dried and characterized by SEC (lin-PG-BzA1 in Table S1,† Mn = 600, Mw/Mn = 1.50).
Preparation of pigment dispersions with PG-based synergists under bead milling process
The colorant (pigment dispersion) was prepared following the composition shown in Table S2.† All raw materials at the milling step were charged into the vessel. The mixture was allowed to mix in the high-speed disperser at 2000 rpm to achieve homogeneous dispersion. The pigment dispersion was characterized without further purification.
Evaluation of pigment dispersions
The viscosities of pigment dispersions were analyzed by using Brookfield viscometer (DV-II+Pro) under the condition of 5 rpm for 6 min at 25 °C. To evaluate the storage stability, the dispersion viscosity was analyzed after 5 days at 50 °C of preparing the dispersions and compared with the viscosity of initial sample.
Measurement
The Mn and Mw/Mn of polymers were measured by SEC at 45 °C using DMF as an eluent. For the DMF-SEC, three polystyrene-gel columns [KD-802 (from Shodex); pore size, 150 Å; 8 mm i.d. × 300 mm, KD-803 (from Shodex); pore size, 500 Å; 8 mm i.d. × 300 mm, KD-804 (from Shodex); pore size, 1500 Å; 8 mm i.d. × 300 mm] were connected to a PU-4180 pump, a RI-4030 refractive-index detector, and a UV-4075 ultraviolet detector (JASCO), and the flow rate was set to 1.0 mL min−1. The columns were calibrated against 13 standard poly(ethylene glycol) (PEO) samples (Agilent Technologies; Mp = 980–811
500; Mw/Mn = 1.03–1.11). 1H NMR was recorded on a Bruker Ultrashield spectrometer operating at 300 MHz. All spectra were recorded in ppm units with deuterated solvents at room temperature. Differential scanning calorimetry (DSC) was conducted on polymer samples under a dry nitrogen flow at a heating or cooling rate of 10 °C min−1 on a Q2000 calorimeter (TA Instruments). The viscosities of pigment dispersions were analyzed by using Brookfield viscometer (DV-II+Pro) with CPE-40 spindle. Size analyses were performed using dynamic light scattering (DLS, Zetasizer Nano ZS90, Malvern Instruments).
Results and discussion
Design and synthesis of bc-PG based synergists (PGSs)
Numerous synergists have been fabricated with chemically polar moiety or structurally planar molecules because these functional groups facilitate the affinity between dye or pigment and dispersants, leading to the high dispersion and stability in solution. Recently, phthalocyanine derivates modified with functional groups have been reported as a synergists to improve the affinity between pigments and dispersants.16 Specifically, in anthraquinone derivates, the carbonyl, amine, and hydroxyl groups enable hydrogen bonding with pigment, while the phenoxy group provides affinity with nonpolar bulky hydrocarbons.
To design a synergist having such functionalities, benzoyl chloride was employed as a hydrophobic part with a planar structure, which readily reacted with the hydroxy group in the polymer backbone. In addition, a series of anchoring groups represented by phthalimide, naphthalic imides, acridone, or anthraquinone including carbonyl, amine, or arene groups were individually employed to benzoyl-functionalized PG to improve adhesion performance with red pigment via EDCI coupling (Fig. 1).16 The anchor molecules with naphthalic imides had two types of structures, which were synthesized using naphthalenedicarboxylic anhydride or naphthalic anhydride (see ESI† for Experimental section and Fig. S1†).
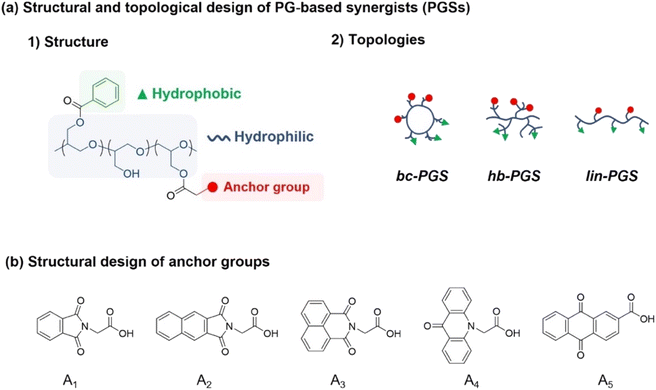 |
| Fig. 1 (a) Design of PG-based synergists (PGSs) with controlled structure and topologies. (b) Design of anchors with functional molecular structures. | |
To evaluate the effect of various anchor groups on the dispersibility and corresponding stability of dispersions, five polymeric synergists based on the topology-controlled bc-PG were first synthesized (Table 1 and Fig. S2†). For example, the aromatic groups from benzoyl and phthalimide groups were clearly confirmed with the peaks in the range of 7.2–8.2 ppm in 1H NMR measurement (Fig. 2). Peak integration between aromatic groups and polymer backbone leads to the ratio of functional groups in synergist, showing 70% of hydroxyl, 20% of benzoyl, and 10% of anchor groups for bc-PG-BzA1 (entry 2 in Table 1). In addition, the corresponding relevant molecular weight of obtained product, which was confirmed by SEC analysis, clearly decreased after the reaction owing to the change in hydrodynamic diameter in the solution, and the glass transition temperature (Tg) of bc-PG-BzA1 increased Tg possibly because of increased molecular weight and rigid groups from hydrophobic moieties. The characteristics of all samples having similar hydrophobic compositions in the bc-PGs in this study are summarized in Table 1.
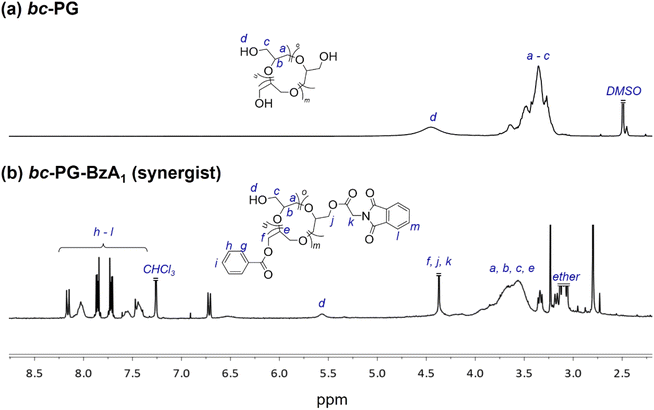 |
| Fig. 2 1H NMR spectra of (a) bc-PG in DMSO and (b) bc-PG-BzA1 synergist in CDCl3. | |
Effect of synergists on Red 170 dispersions
Red 170 dispersions were prepared through the bead-milling process. To understand the effect of as-prepared synergists on the dispersing performance, the viscosity of Red 170 dispersions, which were formulated with additives such as BYK 190 polymeric dispersant and waterborne bc-PG synergists, were monitored at a specific time (Fig. 3 and Table S3†). As a control, in the presence of only the BYK 190 dispersant, the viscosity of the dispersion was optimized under bead-milling process after 12 h, after which an increase in viscosity was observed (square in Fig. 3a). Interestingly, with the addition of imide-type synergists, the optimal bead-milling time was 6 h, which was clearly shorted than that of the control experiment, indicating that these bc-PGSs successfully achieved strong adsorption of dispersants on the pigment surface and stabilized the pigment particles at the early dispersion stage. In particular, the red pigment dispersion with bc-PG-BzA1 exhibited enhanced dispersion performance of 32% compared to the control at the optimal bead-milling time, indicating relatively excellent dispersibility (red dot in Fig. 3a). These results suggest that the bc-PGSs influenced the dispersing performance and that the phthalimide moiety of bc-PG-BzA1 synergist had the most efficient interaction with the pigment among the different types of imides for improving the dispersibility of pigment particles. Although bc-PG-BzA2 and bc-PG-BzA3 showed similar trends as synergists to that of bc-PG-BzA1, their increased hydrophobicity in the anchor group might adversely affect the interaction with the pigment in aqueous solutions (blue and pink dots in Fig. 3a).
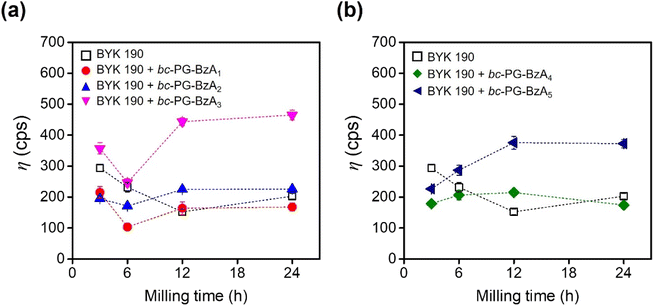 |
| Fig. 3 Viscosities of Red 170 dispersion formulated with BYK 190 dispersant and a series of bc-PG synergists as a function of milling time. | |
In addition to the viscosity results, the particle size of the dispersion samples was analyzed to investigate the cohesiveness of the particles in relation to the dispersibility of the pigment solution (Table S3†). Among the four BYK190 dispersion samples prepared with dispersant and synergists, the samples with only a dispersant and those with synergist dispersions containing phthalimide and naphthalene were investigated because of relative potential for improving dispersion performance. The particle of bc-PG-BzA1 solution was smaller than that of the BYK 190-only dispersion, indicating that bc-PG-BzA1 synergist show superior dispersing performance. Considering that bc-PG-BzA2 and bc-PG-BzA3, with particle sizes of 320.2 nm and 324.1 nm, respectively, had similar sizes to that of the solution applied with only the dispersant, a well-dispersed pigment solution was formed without flocculation. Nevertheless, the sizes of pigment particles applied with bc-PG-BzA2 and bc-PG-BzA3 were slightly larger than that of the pigment solution without synergists, thus supporting that bc-PG-BzA2 and bc-PG-BzA3 used in pigment dispersion had poorer dispersing performance. Based on the results from viscosity and DLS analyses, the bc-PG-BzA1 with phthalimide moiety could be potentially utilized as the optimal synergist to achieve a high dispersion performance in the BYK 190 system.
Meanwhile, the bc-PG-BzA4 and bc-PG-BzA5 synergists tend to increase the viscosity by milling (Fig. 3b). For example, the bc-PG-BzA4 and bc-PG-BzA5, which had the acridone and anthraquinone groups, respectively, showed 35.9 and 149.2 cps point increases, respectively (Table S3†). These results indicate that bc-PG-BzA4 synergist with acridone molecule is relatively advantageous as a synergist to improve dispersibility. However, because the dispersion viscosity with both synergists was increased through ball milling, improving dispersibility by applying these synergists is difficult.
Colloid stability is the most important property in pigment dispersion solutions. Poor dispersion stability can lead to coagulation or agglomeration, increase in size and viscosity, aggregation and precipitation, and phase separation or gelation.36–38 Additives such as dispersants and synergists should improve the dispersion of pigment solutions, as well as provide long-term storage stability without disturbing solution stability. To evaluate the storage stability of the pigment dispersion solutions, we investigated the solution viscosity and particle size in solution after storage at 50 °C for 5 days (Fig. 4 and Table S4†). For example, for entry 1 in Table S4,† that is, the dispersion using BYK 190 without synergist, viscosity and particle size increased by 88.0 cps and 28.8 nm, respectively, after storage owing to the coagulation. Although the dispersions using BYK 190 and bc-PG-BzA1 showed similar increases to those of the control, the change in particle size was relatively smaller than that of the dispersion using BYK 190. In particular, the low standard deviation for viscosity of the dispersion using BYK 190 and bc-PG-BzA1 indicates uniform particles and a relatively stable solution state in the long-term storage at high temperature. By contrast, the decreased viscosity of bc-PG-BzA3 in Fig. 4 was attributed to particle agglomeration, causing precipitation or gelation and indicating low storage stability. Nevertheless, most of the prepared pigment dispersions showed proper stability under severe conditions. These results support that the bc-PGS influence the stability of the dispersion as well as the dispersibility.
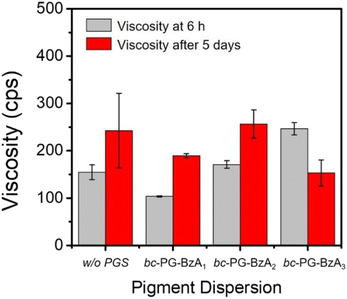 |
| Fig. 4 Storage stability of Red 170 dispersions in bc-PG synergist systems. | |
Topology-controlled polymeric synergists in dispersion
Based on the results of dispersant- or synergist-based pigment dispersion experiments, bc-PG modified with benzoyl and anchor groups are suitable in pigment dispersion systems. In particular, among anchor molecules, the phthalimide structure was demonstrated to be useful as a synergist for pigment dispersion. Given the excellent dispersing performance of the combination of BYK 190 and bc-PG-BzA1, the phthalimide and benzoyl moieties were employed in topology-controlled PGs (linear and hyperbranched PGs). The topology-controlled synergists were similarly synthesized and analyzed in the same manner as bc-PG-BzA1 (Fig. S3†).
To investigate the effect of polymer topologies on the dispersion system, topology-controlled hb-PGS and lin-PGS were employed in the dispersion formulation, which was identical to that of the bc-PG-BzA1 system. Therefore, each viscosity change of dispersions was first monitored to optimize the bead-milling time (Fig. 5a). Similarly, the optimal bead-milling time for each case was 6 h, which was identical to that of the bc-PGS system, indicating that the topology-controlled hb-PGS and lin-PGS successfully achieved a strong adsorption of dispersants on the pigment surface and were stabilized (Fig. 5a). However, the lin-PG-BzA1 synergist system was more viscous than the others, supporting that the branched polymer structures, bc-PG-BzA1 and hb-PG-BzA1, which had steric conformance, exhibited better binding ability with pigment particles and dispersants than the linear analog. Zeng et al. reported that branched polyurethane dispersants exhibited outstanding dispersing property because of their three-dimensional structure and numerous functional groups. In particular, the structure of the branch of the polymer dispersant adsorbed on the surface of the pigment particle formed a steric hindrance to prevent the aggregation of the pigment particle, thereby ensuring dispersion stability.11 In addition, because the lin-PG-BzA1 system had higher viscosity than that with only the BYK 190 dispersant, the use of lin-PG-BzA1 as a synergist eventually disturbed the dispersing performance in the pigment solution.
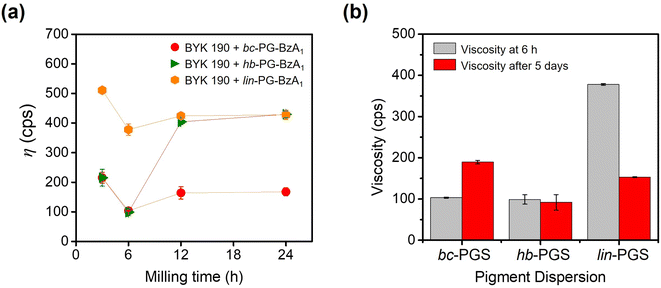 |
| Fig. 5 (a) Viscosities of Red 170 dispersion depending on topology-controlled PG synergists and (b) long-term stability of pigment dispersion with BYK 190 depending on the topology-controlled PGSs after storage for 5 days at 50 °C. | |
To understand the effect of polymer topologies on the storage stability in the dispersion, we analyzed the changes in the viscosity and particle sizes of each pigment solution stored at 50 °C for 5 days (Fig. 5b and Table S5†). While the bc-PG-BzA1 provided relatively good high-temperature storage stability, hb-PG-BzA1 showed excellent high-temperature storage stability. For example, in contrast to that using bc-PG-BzA1, the viscosity of dispersion using hb-PG-BzA1 was 91.9 cps after 5 days of storage, which was similar to the viscosity of the system before storage. In addition, the coagulation behavior of dispersed particles was not induced during high-temperature storage. Coagulation did not occur because the steric hindrance on the surface of pigment by hyperbranched structure of hb-PG-BzA1 prevented the flocculation of pigment particles during storage.11,26 However, the lin-PG-BzA1 system showed a decrease in viscosity of dispersion to 152.9 cps from the initial 378.0 cps, and the particle size was reversibly increased to 352.2 nm. This finding demonstrated that such a linear structure had low dispersing ability in the initial dispersion and was not effective for preventing particle aggregation by flocculation during long-term storage.39,40 Therefore, the lin-PG-BzA1 dispersion system was not suitable for improving the dispersibility and stability of pigment solution.
Conclusions
This study successfully synthesized bc-PG-based waterborne synergists with different anchor groups through simple post-polymerization modification and employed to a Red 170 dispersion system with BYK 190 as polymeric dispersant. Particularly, the phthalimide group as an anchor group enhanced the performance of the Red 170 dispersion system and was identically applied to the hb-PG and lin-PG to evaluate the effect of polymer topologies. Although these three types of waterborne synergists improved the dispersion performance, branched type PGSs were the most effective for enhancing the Red 170 dispersibility because they achieved strong adsorption of dispersants on the pigment surface and stabilized the pigment particles at the early dispersion stage. Despite similar structural features between bc-PGS and hb-PGS, interestingly, hb-PGS promoted stable storage stability at high temperatures through steric hindrance on the surface of the pigment particles. Therefore, we expect that this new approach on the topological effects of polymeric synergists for the pigment dispersion to contribute to the development of advanced waterborne synergists with considerable potential for dispersion systems.
Author contributions
The manuscript was written with contributions from all authors. All of the authors approved the final version of the manuscript. H. K. and S. E. K.: experimentation, analysis, and writing; Y. I. P., J. C. K, J.-E. J., H. J., H. L: analysis; S. Y. H., I. W. C., S.-H. L., and E. S.: conceptualization, supervision, and editing.
Conflicts of interest
The authors declare no competing financial interest.
Acknowledgements
This study was supported by the Ministry of Trade, Industry and Energy (MOTIE, Korea) under the Industrial Technology Innovation Program (No. 20011123) and the Korea Research Institute of Chemical Technology (KRICT) (No. KS2341-20). This work was supported by the National Research Foundation of Korea (NRF) grant funded by the Korean government (MSIT) (RS-2022-00165897). This work was also supported by the Ministry of Science, ICT & Future Planning (NRF-2022M3J4A1091450).
References
- T. Masuda, Y. Kudo and D. Banerjee, Coatings, 2018, 8, 282 CrossRef
. - S. Singh, S. K. Pandey and N. Vishwakarma, in Handbook of functionalized nanomaterials for industrial applications, Elsevier, 2020, pp. 717–730 Search PubMed
. - G. Carotenuto, Y. S. Her and E. Matijevic, Ind. Eng. Chem. Res., 1996, 35, 2929–2932 CrossRef CAS
. - A. G. Abel, Paint and Surface Coatings: Theory and Practice, 1999, pp. 91–165 Search PubMed
. - E. Baez, N. Quazi, I. Ivanov and S. N. Bhattacharya, Adv. Powder Technol., 2009, 20, 267–272 CrossRef CAS
. - A. Gürses, M. Açıkyıldız, K. Güneş and M. S. Gürses, Dyes Pigm., 2016, 13–29 Search PubMed
. - H. Widiyandari, F. Iskandar, N. Hagura and K. Okuyama, J. Appl. Polym. Sci., 2008, 108, 1288–1297 CrossRef CAS
. - H. J. Spinelli, Adv. Mater., 1998, 10, 1215–1218 CrossRef CAS
. - Z. Pu, X. Fan, J. Su, M. Zhu and Z. Jiang, Colloid Polym. Sci., 2022, 300, 167–176 CrossRef CAS
. - C. Auschra, E. Eckstein, A. Muhlebach, M. O. Zink and F. Rime, Prog. Org. Coat., 2002, 45, 83–93 CrossRef CAS
. - T. Zeng, G. He, X. Li and C. Wang, J. Appl. Polym. Sci., 2021, 138, 50790 CrossRef CAS
. - J. Choi, W. Lee, J. W. Namgoong, T. M. Kim and J. P. Kim, Dyes Pigm., 2013, 99, 357–365 CrossRef CAS
. - F. O. H. Pirrung, P. H. Quednau and C. Auschra, Chimia, 2002, 56, 170–176 CrossRef CAS
. - C. Agbo, W. Jakpa, B. Sarkodie, A. Boakye and S. H. Fu, J. Dispersion Sci. Technol., 2018, 39, 874–889 CrossRef CAS
. - M. M. Gacek and J. C. Berg, Electrophoresis, 2014, 35, 1766–1772 CrossRef CAS PubMed
. - J. W. Namgoong, S. W. Chung, H. Jang, Y. H. Kim, M. S. Kwak and J. P. Kim, J. Ind. Eng. Chem., 2018, 58, 266–277 CrossRef CAS
. - C. Yoon, J. H. Choi and J. P. Kim, Mol. Cryst. Liq. Cryst., 2010, 533, 102–112 CrossRef CAS
. - A. T. Kelley, P. J. Alessi, J. E. Fornalik, J. R. Minter, P. G. Bessey, J. C. Garno and T. L. Royster, ACS Appl. Mater. Interfaces, 2010, 2, 61–68 CrossRef CAS
. - C. Yoon and J. H. Choi, Dyes Pigm., 2014, 101, 344–350 CrossRef CAS
. - G. Nian, J. Kim, X. Bao and Z. Suo, Adv. Mater., 2022, 34, e2206577 CrossRef PubMed
. - T. Liu, W. Chen, K. Li, S. Long, X. Li and Y. Huang, Polymers, 2023, 15, 2644 CrossRef CAS PubMed
. - B. I. Voit and A. Lederer, Chem. Rev., 2009, 109, 5924–5973 CrossRef CAS PubMed
. - R. A. Shenoi, J. K. Narayanannair, J. L. Hamilton, B. F. Lai, S. Horte, R. K. Kainthan, J. P. Varghese, K. G. Rajeev, M. Manoharan and J. N. Kizhakkedathu, J. Am. Chem. Soc., 2012, 134, 14945–14957 CrossRef CAS
. - C. J. Hawker and J. M. J. Frechet, J. Am. Chem. Soc., 1990, 112, 7638–7647 CrossRef CAS
. - S. Y. Lee, M. Kim, T. K. Won, S. H. Back, Y. Hong, B. S. Kim and D. J. Ahn, Nat. Commun., 2022, 13, 6532 CrossRef CAS PubMed
. - D. J. Lunn, S. Seo, S. H. Lee, R. B. Zerdan, K. M. Mattson, N. J. Treat, A. J. McGrath, W. R. Gutekunst, J. Lawrence, A. Allison, A. Anastasaki, A. S. Knight, B. V. K. J. Schmidt, M. W. ates, P. G. Clark, J. P. DeRocher, A. K. Van Dyk and C. J. Hawker, J. Polym. Sci., Part A: Polym. Chem., 2019, 57, 716–725 CrossRef CAS
. - T. P. Holbrook, G. M. Masson and R. F. Storey, J. Polym. Sci., Part A: Polym. Chem., 2019, 57, 1682–1696 CrossRef CAS
. - G. Y. Wang, Y. Y. Bai, X. Y. Ma, W. X. Wang, Q. W. Yin and Z. P. Du, J. Mol. Liq., 2017, 225, 333–338 CrossRef CAS
. - G. P. Lokhande and R. N. Jagtap, Prog. Org. Coat., 2016, 90, 359–368 CrossRef CAS
. - Y. Y. Xu, J. C. Liu, C. S. Du, S. H. Fu and X. Y. Liu, Prog. Org. Coat., 2012, 75, 537–542 CrossRef CAS
. - S. E. Kim, H. J. Yang, S. Choi, E. Hwang, M. Kim, H.-J. Paik, J.-E. Jeong, Y. I. Park, J. C. Kim, B.-S. Kim and S.-H. Lee, Green Chem., 2022, 24, 251–258 RSC
. - S. E. Kim, Y. R. Lee, M. Kim, E. Seo, H. J. Paik, J. C. Kim, J. E. Jeong, Y. I. Park, B. S. Kim and S. H. Lee, Poly. Chem., 2022, 13, 1243–1252 RSC
. - J. Kim, S. Choi, J. Baek, Y. I. Park, J. C. Kim, J. E. Jeong, H. Jung, T. H. Kwon, B. S. Kim and S. H. Lee, Adv. Funct. Mater., 2023, 2302086 CrossRef CAS
. - S. Choi, J. Kim, E. Seo, H. Y. C. Jung, J. E. Jeong, Y. I. Park, J. C. Kim, D. W. Lee, B. S. Kim and S. H. Lee, Poly. Chem., 2023, 14, 1184–1194 RSC
. - J. Song, L. Palanikumar, Y. Choi, I. Kim, T. Y. Heo, E. Ahn, S. H. Choi, E. Lee, Y. Shibasaki, J. H. Ryu and B. S. Kim, Poly. Chem., 2017, 8, 7119–7132 RSC
. - J. Dong, S. Chen, D. S. Corti, E. I. Franses, Y. Zhao, H. T. Ng and E. Hanson, J. Colloid Interface Sci., 2011, 362, 33–41 CrossRef CAS PubMed
. - E. S. Fabjan, M. Otonicar, M. Gaberscek and A. S. Skapin, Dyes Pigm., 2016, 127, 100–109 CrossRef
. - C. C. Li, S. J. Chang, C. W. Wu, C. W. Chang and R. H. Yu, J. Colloid Interface Sci., 2017, 506, 180–187 CrossRef CAS PubMed
. - Y. Wang, J. E. Q. Quinsaat, T. Ono, M. Maeki, M. Tokeshi, T. Isono, K. Tajima, T. Satoh, S. I. Sato, Y. Miura and T. Yamamoto, Nat. Commun., 2020, 11, 6089 CrossRef CAS PubMed
. - M. A. Aboudzadeh, J. Kruse, M. Sanroman Iglesias, D. Cangialosi, A. Alegria, M. Grzelczak and F. Barroso-Bujans, Soft Matter, 2021, 17, 7792–7801 RSC
.
Footnotes |
† Electronic supplementary information (ESI) available: Experimental details; synthetic procedures and characterization of anchor molecules, analysis of topology-controlled PG-based synergists (PDF). See DOI: https://doi.org/10.1039/d3ra06427a |
‡ These authors contributed equally to the work. |
|
This journal is © The Royal Society of Chemistry 2023 |
Click here to see how this site uses Cookies. View our privacy policy here.