DOI:
10.1039/D2SC04167G
(Edge Article)
Chem. Sci., 2023,
14, 103-112
Endofungal bacteria boost anthelminthic host protection with the biosurfactant symbiosin†
Received
26th July 2022
, Accepted 20th November 2022
First published on 21st November 2022
Abstract
Effective protection of soil fungi from predators is crucial for their survival in the niche. Thus, fungi have developed efficient defence strategies. We discovered that soil beneficial Mortierella fungi employ a potent cytotoxin (necroxime) against fungivorous nematodes. Interestingly, this anthelminthic agent is produced by bacterial endosymbionts (Candidatus Mycoavidus necroximicus) residing within the fungus. Analysis of the symbiont's genome indicated a rich biosynthetic potential, yet nothing has been known about additional metabolites and their potential synergistic functions. Here we report that two distinct Mortierella endosymbionts produce a novel cyclic lipodepsipeptide (symbiosin), that is clearly of bacterial origin, but has striking similarities to various fungal specialized metabolites. The structure and absolute configuration of symbiosin were fully elucidated. By comparative genomics of symbiosin-positive strains and in silico analyses of the deduced non-ribosomal synthetases, we assigned the (sym) biosynthetic gene cluster and proposed an assembly line model. Bioassays revealed that symbiosin is not only an antibiotic, in particular against mycobacteria, but also exhibits marked synergistic effects with necroxime in anti-nematode tests. By functional analyses and substitution experiments we found that symbiosin is a potent biosurfactant and that this particular property confers a boost in the anthelmintic action, similar to formulations of therapeutics in human medicine. Our findings illustrate that “combination therapies” against parasites already exist in ecological contexts, which may inspire the development of biocontrol agents and therapeutics.
Introduction
Symbiotic associations are widespread among all kingdoms, shaping not only the lifestyle of the involved partners, but also the surrounding environment and ecological systems.1–4 Omnipresent in all of the world's habitats, in marine environments, flora and soil biotopes, symbioses can influence the diversity and composition of species in an ecological community and thus play a central role in the development and maintenance of an ecological system.4–8 In mutualistic associations, partnerships that are beneficial to all symbionts, different organisms live together and combine their individual skills to promote assertiveness or supply nutrients to the alliance.3,9,10 While one partner may provide the food supply,11,12 the other partner may possess the genomic abilities to biosynthesize a selection of natural products, such as communication molecules, UV-protectants or antibiotics.13–17 A particularly important role is played by the diverse defense molecules provided by symbionts against competing bacteria, fungi or even higher organisms that can protect the host from predators. Many studies have shown that symbioses are valuable sources of natural products with pharmaceutically relevant activities such as antibiotics or anti-cancer compounds.16,18–22 The molecules not only provide a rich source of new, efficient drug candidates, but can teach us strategies which can be adapted in agriculture or medicine.
Among the different symbiotic lifestyles are associations between fungi and bacteria, with endosymbiotic interactions being the most intimate examples.23–26 In these partnerships the bacteria live inside the fungal hyphae and benefit from a steady environment and nutritional support. Meanwhile, the fungus can be influenced in its reproduction,27–29 growth behavior,30 energy dynamics,31 and by host-supporting secondary metabolites biosynthesized by the bacterium.32,33
Symbioses between fungi and endobacteria are most abundant in the fungal phylum Mucoromycota, harboring Burkholderia-related bacteria.26,34 Beside the well-studied symbiosis between Rhizopus microsporus and Mycetohabitans rhizoxinica, where the endosymbiont produces the highly cytotoxic macrolide rhizoxin,32 a high prevalence of endosymbiotic bacteria among the Mortierellomycotinan fungi was reported.34 Previous studies regarding the existence of endosymbionts in different Mortierella strains revealed the presence of bacteria in 37% of the tested species.34
Among the known endosymbiont harboring and soil beneficial Mortierella strains is Mortierella verticillata NRRL 6337. We recently discovered that the antihyperlipidaemic, but also highly cytotoxic necroximes C and D (1 and 2; syn. CJ12.950 and CJ13.357)35 (Fig. 1A), formerly believed to be fungal metabolites, are not produced by the fungus but its endosymbiotic bacteria.33 Moreover, the necroximes exhibit anthelmintic activity and function as potent protectants against nematodal micropredators.33
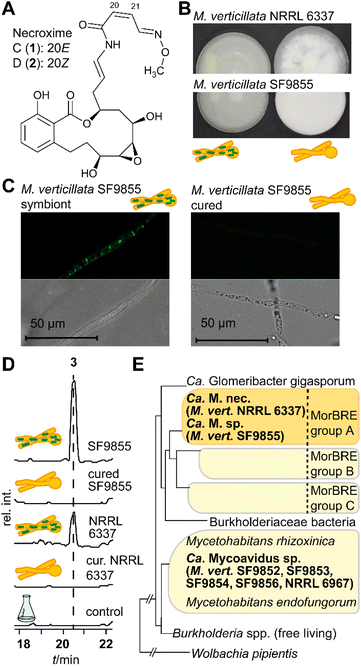 |
| Fig. 1 Natural products of Candidatus Mycoavidus endosymbionts inhabiting M. verticillata strains. (A) Structure of necroxime C (1) and D (2), produced by the endosymbiont of M. verticillata NRRL 6337 (Ca. Mycoavidus necroximicus). (B) Symbiotic (left) and cured (right) cultures of M. verticillata NRRL 6337 and SF9855. (C) Fluorescence microscopy pictures and the corresponding brightfield pictures (of M. verticillata SF9855 with its endosymbiont (left) and as a cured strain without endosymbionts (right)). (D) Metabolic profiles of the two symbiosin producer strains and their corresponding cured (cur.) strains. (E) Phylogenetic relationships of symbiosin producer strains, additional endosymbiotic bacteria from M. verticillata screened in this work (Candidatus Mycoavidus spp.), and other fungi. | |
Genome analysis of the necroxime-producing endobacterium Candidatus Mycoavidus necroximicus revealed 18 additional biosynthetic gene clusters for secondary metabolites, for which no corresponding metabolites have been identified.33 This high metabolic potential is unusual among Mycoavidus endosymbionts,33 and other symbiont metabolites that may have additional or synergistic protective effects for the host fungus are unknown.
Here we show that obligate endosymbiotic bacteria of two M. verticillata strains produce a novel cyclic lipodepsipeptide, symbiosin (3). We report that 3 not only acts as an antimycobacterial agent, but also boosts the toxic effect of necroxime D against nematodes and thereby enhances the protective effect of the bacterial metabolites. Our findings illustrate that “combination therapies”, known from human medicine, also occur in an ecological context as a strategy to shield fungi from predators.
Results and discussion
Discovery of a novel cyclic depsipeptide from a fungal-bacterial symbiosis
To investigate the biosynthetic potential of bacterial endosymbionts of M. verticillata, we monitored the metabolic profiles of seven fungal strains for which we have verified the presence of Mycoavidus bacteria by PCR (16S rDNA).33 We varied culture conditions and media, and analyzed the culture extracts by high-performance liquid chromatography (HPLC). In order to assign specialized metabolites to the endosymbionts, we compared symbiotic M. verticillata strains with symbiont-free (cured) M. verticillata strains (Fig. 1B and C). In the culture broths of two strains, M. verticillata NRRL 6337 and M. verticillata SF9855, we detected a previously unknown compound (3), named symbiosin (Fig. 1D and S1, ESI†). Using high-resolution electrospray ionization mass spectrometry (HRESI/MS) we assigned a mass of 962.5 Da to 3 and deduced its chemical formula of C49H70N8O12 (calcd m/z 963.5186 [M + H]+ and found m/z 963.5184 [M + H]+). The MS/MS fragmentation pattern indicated a peptide backbone. Retention times, exact masses and MS/MS fragmentation patterns of the metabolites detected in the symbiotic M. verticillata NRRL 6337 and M. verticillata SF9855 cultures proved to be identical.
Interestingly, a phylogenetic analysis of amplified 16S rDNA of the endosymbionts shows that the symbionts associated with a symbiosin-positive phenotype are more closely related to each other than to the other fungal endosymbionts of M. verticillata (Fig. 1E, adapted from previous findings).33 Notably, the new compound could not be detected in cultures of the sterile fungal strains lacking the endosymbiotic Ca. Mycoavidus strains (Fig. 1D), suggesting that either symbiosin is produced by the endobacteria or the presence of the endosymbionts triggers symbiosin production in the fungal host.
To characterize the new metabolite, we subjected the ethyl acetate extract of a five week-old holobiont culture (4 L) to size-exclusion chromatography with Sephadex LH-20, followed by preparative HPLC, yielding 8.8 mg of pure 3. By a combination of 1D- and 2D-NMR measurements, LC-HRESI/MS, hydrolysis and LC-HRESI/MS/MS fragmentation, we elucidated the structure of 3 (Fig. 2A). The number of proton and carbon signals measured in 1H and 13C NMR experiments supports the chemical formula deduced from HRESI/MS data. 13C NMR and DEPT135 measurements identified 13 quaternary carbon atoms, 16 methines, 18 methylenes, and two methyl groups. Additional signals in the 1H spectrum indicated the presence of seven primary amide protons. 1H–1H COSY spectra in combination with HMBC couplings revealed the amino acid sequence of Gln, Thr, β-Ala, Trp, Ser, and Tyr with an ester bond between the carbonyl-group of Tyr and the hydroxy-group of Thr. Furthermore, we found that 3-hydroxy-myristic acid is attached to the N-terminus of Gln (Fig. 2A and Table S10, ESI†). By means of Marfey's method we determined the absolute configurations of the amino acids, D-Gln, L-Thr, D-Trp, L-Ser and D-Tyr. Mosher esterification followed by HPLC analysis revealed the 3R-configuration of the hydroxy fatty acid (Fig. 2B, C and S4, ESI†). Taken together, symbiosin is a previously unknown compound belonging to the family of cyclic lipodepsipeptides.
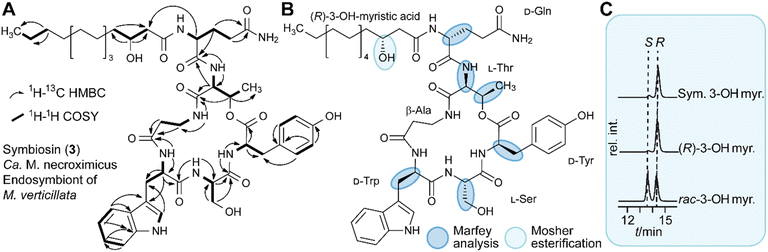 |
| Fig. 2 Structure elucidation of symbiosin. (A) Structure of symbiosin (3) with key 1H–1H COSY and 1H–13C HMBC couplings from 2D-NMR experiments. (B) Absolute configuration of 3 and used analysis methods. (C) Chromatographic profiles of Mosher ester analysis for configuration elucidation of the hydroxy myristic acid residue (myr.) in symbiosin (sym). | |
Bacteria-produced symbiosin resembles fungal metabolites
Interestingly, symbiosin is structurally similar to the known natural products colisporifungin (4), ophiotine (5), verruculin (6), and aselacin A (7) (Fig. 3A and B).36–39 It is remarkable that all of these compounds (4–7) have been isolated from fungi of the phylum Ascomycota, which are not described to harbor endosymbionts. Except for the terminal amino acids that are involved in lactone ring formation, the peptide backbones of lipopeptides 3–7 are almost identical. The main differences between these lipopeptides are notable in the fatty acid side chains. In contrast to 4–7, 3 has a β-hydroxy fatty acid attached to the extracyclic glutamine residue (Fig. 3B). The presence of a β-hydroxy fatty acid is a hallmark of lipopeptides from Gram-negative bacteria,40 thus implicating endobacteria as the source of 3. Validations of the biosynthetic assembly line of 4–7 are not possible, as no genomic data of the fungi are available.
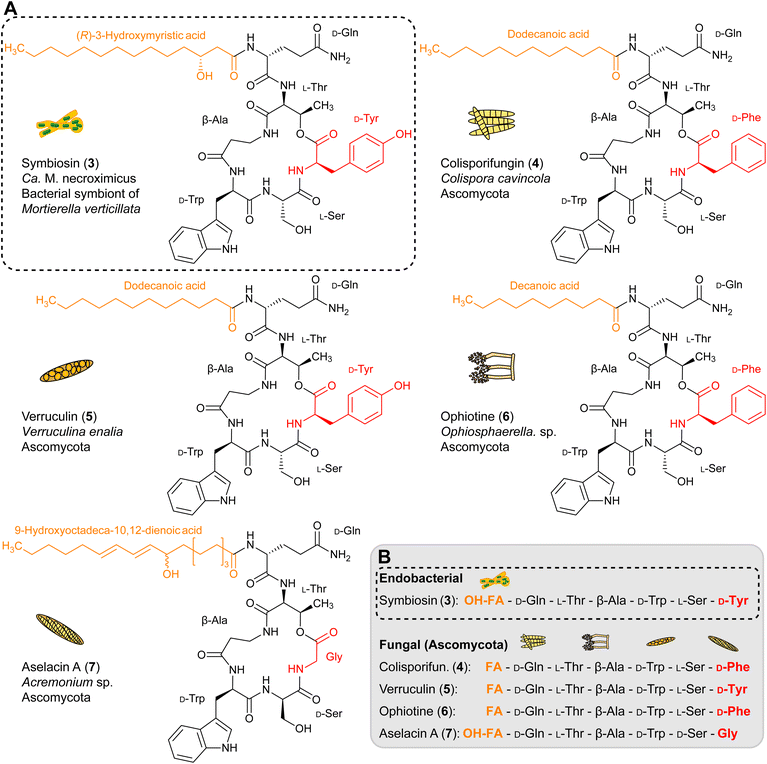 |
| Fig. 3 Structural similarities between symbiosin and related fungal lipopeptides. (A) Structures of compounds similar to symbiosin (3) (colisporifungin (4), ophiotine (5), verruculin (6), and aselacin A (7)) produced by Ascomycota. Structural differences are colour-coded. (B) Comparison of fatty acid and amino acid sequences of 3 and similar compounds. | |
Genetic origin and model of symbiosin biosynthesis
To support the assumption that bacteria are the producers of 3, and to rule out a fungal biosynthesis, we searched for the gene cluster coding for the symbiosin assembly line. First, we used fungal antiSMASH to search for a possible biosynthetic gene cluster in the fungal genome of M. verticillata NRRL 6337.41 Although genes encoding NRPSs could be identified, the deduced assembly lines do not fit the structure of 3 (Fig. S5†). Thus, we turned to the endobacterial genomes.
We reasoned that the discovery of the candidate gene clusters would be facilitated by comparison of the endobacterial genomes of both symbiosin-positive symbioses. Therefore, we isolated and sequenced the genomic DNA of the Candidatus Mycoavidus sp. of M. verticillata SF9855 (GenBank: CP102085) in a similar way as previously performed for Ca. M. necroximicus.33 The availability of two related genome sequences proved to be helpful in the reassessment of the genome of Ca. M. necroximicus (GenBank: CP076444) and the assembly of related contigs by means of Sanger sequencing allowed us to rectify the genome sequence. The average nucleotide identity of both genomes is 96.91%, meaning that they can be considered the same species.42 Mining of the endosymbiont genome sequences revealed several putative NRPS gene clusters (Fig. 4A). Among the deduced NRPS-type assembly lines, we identified one in both genomes that is the best candidate for the biosynthesis of 3 (Fig. 4B and S7, ESI†).
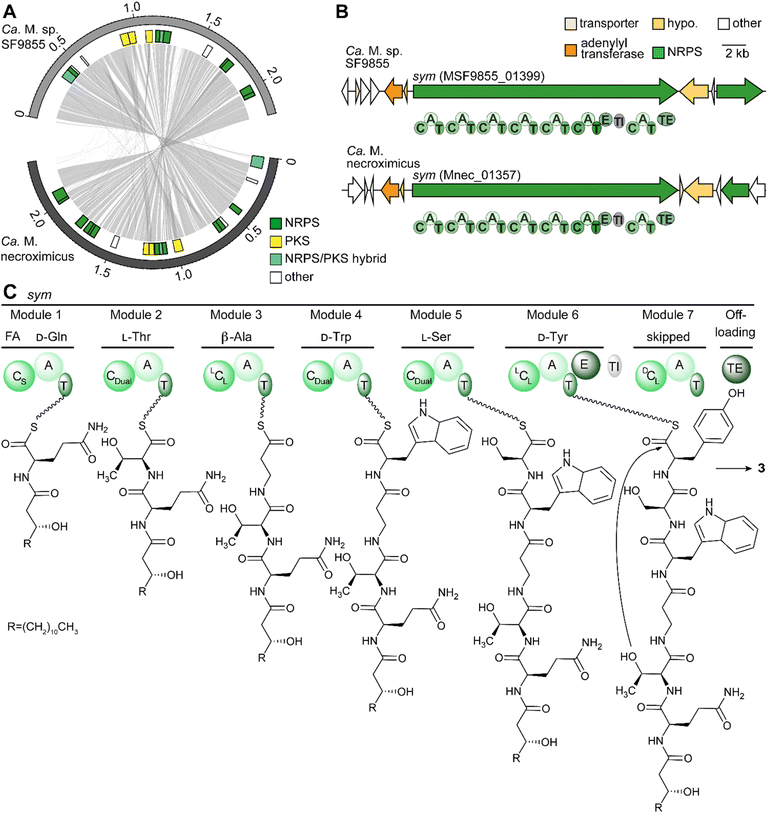 |
| Fig. 4 Secondary metabolites encoded in bacterial genomes and the model of symbiosin (3) biosynthesis. (A) Comparison between the genomes of Ca. M. sp. SF9855 (GenBank: CP102085) and Ca. M. necroximicus (GenBank: CP076444). (B) Symbiosin biosynthesis gene cluster in the genomes of Ca. M. sp. SF9855 and Ca. M. necroximicus; hypo: hypothetical protein. (C) Proposed assembly line for symbiosin. FA: fatty acid; C: condensation domain (S: starter; dual: condensation/epimerization); A: adenylation domain; T: thiolation domain; E: epimerization domain; TI: TIGR01720 domain, domain of unknown function; TE: thioesterase domain. | |
The deduced sym NRPS consists of seven modules. In silico prediction of the adenylation (A) domain specificities43 indicated that the first six modules would produce a heptapeptide composed of Gln, Thr, β-Ala, Trp, Ser, and Tyr, which is in full agreement with the hexapeptide backbone of 3 (Tables S3–S6, ESI†). The A domains of these modules have a similarity between 64.5% and 82.8% in both strains (Table S4, ESI†).
The presence of a seventh NRPS module is, however, surprising. Scrutinizing the amino acid sequence of this terminal module indicated several variations in conserved core motifs (Table S4 and Fig. S8, ESI†) and the complete loss of a flavodoxin-like A subdomain with catalytically important core residues in one of the deduced NRPS sequences (Fig. S8 and S9, ESI†).44,45 The identity between these extra modules is high at 96.36%, whereas the identities to all other domains are between 38.7% and 44.7% (Table S4, ESI†). Thus, we concluded that module seven does not incorporate any additional amino acids. There has been precedence that such catalytically non-functional NRPS modules are skipped.46,47
A particularly valuable indicator for the identity of the sym NRPS is the β-Ala specificity of module 3. We determined the Stachelhaus-code sequence and active site residues proposed for β-Ala specific domains (Fig. S10, ESI†).48,49 Specifically, the position of the aspartate residue, which is usually conserved in A domains in the A4 motif of α-amino acids (FDxS), differs in all described β-Ala specific A domains (Fig. S10, ESI†).44,49 This deviation is plausible because the negatively charged carboxy group of this aspartate residue interacts with the amino group of the incorporated amino acid,44 and the spatial arrangements of the α- and β-amino groups clearly differ in the active site.
In addition to the A domain specificities of the other six modules, the condensation (C) domains of the deduced modules fit the experimentally determined structure and absolute configuration of 3 (Fig. 4C). Specifically, the CStarter domain would load (R)-3-hydroxy-myristic acid, as these domains are known to introduce fatty acids onto the C-terminus of initiating NRPs.50 The dual condensation/epimerization C domains (CDual) in modules 2 and 5 are responsible for the epimerization of the prior amino acid, resulting in D-Gln and D-Trp.51 A third CDual domain is found in module 4, which would act on β-Ala. Since β-Ala has no stereocenter, no epimerization can take place on this amino acid. In the case of D-Tyr, an additional epimerization domain changes the configuration of the introduced L-amino acid building block into the D-isomer. The remaining LCL-domains in modules 3 and 6 are in accordance with the determined configurations of L-Ser and L-Thr.
Although it is impossible to rigorously verify the gene cluster assignment by functional gene analysis in the as-yet unculturable symbionts, the genomic and bioinformatic analyses provide strong evidence for the identity of the sym gene cluster encoded in the bacterial genome.
Symbiosin is an antimycobacterial agent
To identify potential biological functions of the symbiont-derived lipopeptide, we subjected 3 to a panel of whole-cell bioassays using representative bacterial and fungal strains, as well as cancer cell lines. No cytotoxicity was observed on HeLa cells or HUVEC cells and only a moderate antiproliferative effect on K-562 (37.5 μM) was observed (Table S7, ESI†). In an initial antimicrobial assay 3 showed moderate activity against several bacterial strains, including Bacillus subtilis 6633B1, Staphylococcus aureus 511B3 and vancomycin-resistant Enterococcus faecalis 1528R10, and was found to be particularly active against Mycobacterium vaccae (Table S8, ESI†). Thus, we tested a range of mycobacteria and determined MIC values of 3 against M. vaccae (6.49 μM), Mycobacterium smegmatis (6.49 μM), and Mycobacterium aurum (12.98 μM). Interestingly, anti-mycobacterial activities, which have been evaluated for 5 and 6, were only reported for 6,39 which shares tyrosine with 3 as the macrocyclic ring-forming amino acid.
Synergistic effect of bacterial metabolites protects the fungal host from nematodes
Since the structurally related 5 has moderate nematocidal activities,37 and because Ca. M. necroximicus has been found to protect the host from nematode attacks,33 we next tested the anthelmintic activity of 3. Surprisingly, 3 alone showed no effect on the model nematode Caenorhabditis elegans (concentrations up to 100 μg mL−1). To evaluate a potential synergistic effect with the necroximes, we evaluated the combined activity of necroxime (2) on C. elegans in the presence of three different concentrations of 3 (0.2 μg mL−1, 2 μg mL−1 and 20 μg mL−1; notably, 2.2 μg mL−1 corresponds to the amount of 3 isolated from fungal holobiont cultures). Therefore, we incubated the nematodes and their bacterial food source in liquid media with increasing concentrations of 2, while keeping a steady concentration of 3. Subsequently, we measured the OD600 of the bacterial suspension, which is an indirect measurement for nematode viability.52 The IC50 of necroxime alone was determined to be 10.78 μg mL−1 (11.4 μg mL−1,33 95% confidence interval 9.83–13.00 μg mL−1; Fig. 5A). If necroxime (2) was combined with 3, the anthelmintic activity increased with IC50 values of 6.22 μg mL−1, 5.79 μg mL−1 and 4.13 μg mL−1 necroxime, if 0.2 μg mL−1, 2 μg mL−1 or 20 μg mL−1 of 3 was present, respectively (Fig. 5A, B and S11, ESI†).
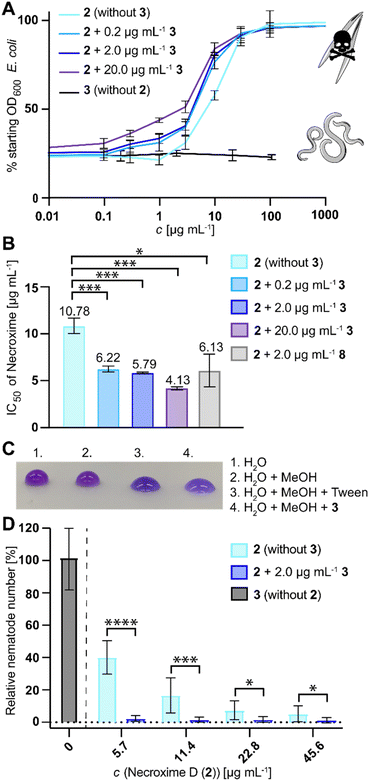 |
| Fig. 5 Biological activities. (A) Synergistic anthelmintic effect of necroxime D (2) and symbiosin (3). Nematode viability measurements in the presence of 3, different concentrations of 2 and of 2 in combination with 0.2 μg mL−1, 2 μg mL−1 or 20 μg mL−13. (B) Differences in IC50 measured for necroxime alone (IC50: 10.78 μg mL−1; 23.54 μM, 95% CI 20.67–26.79 μM) and in combination with 3 in concentrations of 0.2 μg mL−1 (IC50: 6.22 μg mL−1; 13.58 μM, 95% CI 11.80–15.57 μM), 2 μg mL−1 (IC50: 5.79 μg mL−1; 12.64 μM, 95% CI 11.07–14.38 μM) and 20 μg mL−1 (IC50: 4.13 μg mL−1; 9.01 μM, 95% CI 6.76–11.70 μM). CI, confidence interval; *, p < 0.05; ***, p < 0.001. (C) Biosurfactant activity of 3. 1% Tween (positive control); 1 mM 3. (D) Activity of 3 alone (20 μg mL−1) and synergistic activity of 2 in different concentrations in combination with 3 against the fungivorous nematode Aphelenchus avenae. The numbers of harvested nematodes are relative to the numbers of nematodes harvested from cultures without 2 and 3. *, p < 0.05; **, p < 0.01; ***, p < 0.001; ****, p < 0.0001. | |
Considering that 3 alone has no effect on the nematodes, we wondered how this synergistic effect is achieved. The structure of 3, consisting of a lipophilic fatty acid residue and partially hydrophilic amino acids, suggested that it acts as a biosurfactant, which might influence the permeability of substances into the nematodes. We verified the predicted biosurfactant activity of 3 by means of drop collapse assays (Fig. 5C). To test whether the synergistic effect of 3 is based on its tenside activity, we exchanged 3 with another lipodepsipeptide biosurfactant. Therefore, we selected surfactin (8), as it is a strong biosurfactant with a similar size (1036.3 Da) and a cyclic lipodepsipeptide with a structure similar to that of 3.53 Using a steady concentration of 2.0 μg mL−18 and increasing concentrations of 2, we determined an IC50 of 6.13 μg mL−1 for the combination of 2 and 2.0 μg mL−18 (5.92 μM), which is comparable to the results of 2 and 2.0 μg mL−13 (6.01 μM; Fig. 5B and S11, ESI†). Thus, the biosurfactant activity is a plausible reason for the synergistic activity of 3.
Based on the synergistic anthelmintic effects demonstrated with the model nematode C. elegans, it appeared feasible that the increased anthelmintic effect could also play a role in the fungal protection against fungivorous nematodes, especially as 2 was previously shown to be active against the fungivorous nematode Aphelenchus avenae.33 Therefore, we next tested if the presence of 3 affects the nematodes or enhances the protective effect of 2 against A. avenae. We compared the number of harvested nematodes from nematode-fungus co-cultures of a necroxime- and symbiosin-negative M. verticillata strain that was treated with 3 or varying amounts of 2, to cultures that were treated with a combination of 2 and 3. This experimental setup enabled us to quantify the relative reduction of fungivorous nematodes in cultures containing 2 , but even more clearly it showed a significant reduction of nematodes in cultures containing a combination of 2 and 3. Already low concentrations of 2 (5.7 μg mL−1) were sufficient in the combination with physiological amounts of 3 (2.0 μg mL−1) to almost completely eradicate the presence of nematodes (Fig. 5D), which is comparable with reported numbers for M. verticillata NRRL 6337 wild-type cultures.33 In contrast, experiments with 3 alone did not show any effect on nematode numbers, even when tested with concentrations up to 20 μg mL−1 (Fig. 5D). These results show that 2 and 3 synergistically provide protection to the fungus against fungivorous nematodes.
Conclusion
In competitive environments such as soil, elaborate protection strategies are necessary for inhabitants to assert spatial claims against predators and competitors. Particularly effective are mixtures of metabolites, which exhibit synergistically acting defensive activities.54–60 In this study, we report the discovery of the endobacterially produced metabolite symbiosin (3) and demonstrate how it is utilized in fungal host protection to enhance the anthelminthic effect of necroxime (2), illustrating how “combination therapies” are applied in an ecological context. Our data show that the endosymbiont of M. verticillata NRRL 6337 not only produces the toxin necroxime (2), but also synthesises a cyclic lipodepsipeptide (3), which significantly enhances the anthelmintic activity of the toxin 2. Our experiments demonstrate that the protection of the fungal host, even against fungivorous predators, works at physiological concentrations of the two compounds measured in fungal cultures.
The production of secondary metabolites by endofungal bacteria instead of the host fungus has previously been demonstrated for other fungal symbionts. One example is the compound rhizoxin, a virulence factor employed by the plant-pathogenic fungus Rhizopus microsporus.32 The discovery of endosymbionts (Mycetohabitans rhizoxinica syn. Burkholderia rhizoxinica) as the true producers of this toxic metabolite was unforeseen but illustrates the benefits of an endosymbiont for fungi. The toxin is not only the major virulence factor, but additionally plays a role in fungal protection.61 Notably, also other Burkholderia-derived metabolites, such as the toxin rhizonin or the antibiotic icosalide, were originally thought to be fungal metabolites and later proven to be of bacterial origin.62–64
Although functions of these and other endobacterial metabolites include symbiosis promoting activities,25,65–67 host reproduction control68 and pathogenicity causing traits,32,63,69 research on their role in host protection strategies against predators was only conducted recently.33,61 Specifically the combination of protective metabolites in M. verticillata NRRL 6337 plays an extraordinary role, as they are the first metabolites from one endofungal bacterium that synergistically provide host protection.
Examples of effective symbiotic defense strategies are widespread among the different kingdoms, including bacteria, fungi, plants and animals.25,32,54,60 They play important roles in protecting plants,55 guarding fungal gardens in termite or leaf-cutter ant communities,58,59 providing an antimicrobial shield for egg clutches of solitary wasps54 and beetles,60 or protection against grazing of marine sponges.57 In these ecological contexts especially the combination of different complementary substances provides enhanced protection against a range of potential threats.
The synergistic activity of combined bioactive molecules is a known effect in pharmaceutical research and especially useful for the treatment of certain difficult to treat infections or viral infections.70–72 It was shown that not only the therapeutic selectivity and efficacy are improved by the combination of synergistically acting drugs,73,74 but also the side effects of a medication can be reduced, if the concentration of therapeutics can be lowered.75 Similar combined effects are also likely to occur in ecological settings.
While synthetic compounds have been screened for synergistic biocontrol agents against nematodes,76–79 to date little is known about synergistic protection against parasites such as nematodes in the soil. Several studies identified plant-derived compound mixtures to have anthelmintic activities with synergistic effects potentially protecting plants from nematodes,80–82 yet synergistic effects of nematocidal small molecule protectants from bacteria or fungi were previously unknown. This study illustrates the first case of anthelmintic protection of a fungal soil habitant using the combination of a bacterium-derived toxin and biosurfactant. In particular the usage of biosurfactants as activity-enhancing or drug-delivery promoting substances was discussed in recent studies as effective improvements of pharmaceutical formulations.83–85
Our study demonstrates that the principle of combinatorial therapies, common in medical contexts, is also already used in natural contexts, and that further studies of the boosting effects of biosurfactants might be useful in the future developments of combinatorial biocontrol strategies.
Data availability
Genome sequence data have been deposited in the GenBank Project PRJNA733818 (Ca. M. necroximicus: CP076444; Ca. M. sp. SF9855: CP102085).
Author contributions
H. B. and C. H. conceived the study. H. B. carried out the project administration and designed the experiments. H. B. and S. J. P. conducted the experiments. H. B., S. J. P. and K. S. analysed and interpreted the data. H. B. and C. H. wrote the manuscript.
Conflicts of interest
There are no conflicts to declare.
Acknowledgements
We thank A. Perner for LC-MS measurements, H. Heinecke for NMR measurements, C. Weigel for antimicrobial assays, and H. M. Dahse for cytotoxicity assays. Thanks go to S. P. Niehs, B. Dose, B. Urbansky and H. Kries for helpful discussions. This work was financially supported by the Deutsche Forschungsgemeinschaft (DFG, German Research Foundation) – Project-ID 239748522 – SFB 1127 (B01) and Leibniz Award (to C. H.). Mortierella strains were supplied by the ARS Culture Collection (NRRL) and the Jena Microbial Resource Collection (JMRC). C. elegans was provided by the CGC, which is funded by the NIH Office of Research Infrastructure Programs (P40 OD010440). A. avenae was received as a kind gift from Prof. M. Künzler (ETH Zürich).
Notes and references
- J. M. Smith, Nature, 1989, 341, 284–285 CrossRef CAS PubMed.
- J. B. Raina, L. Eme, F. J. Pollock, A. Spang, J. M. Archibald and T. A. Williams, Biol. Open, 2018, 7, bio032524 CrossRef PubMed.
- G. Chomicki, M. Weber, A. Antonelli, J. Bascompte and E. T. Kiers, Trends Ecol. Evol., 2019, 34, 698–711 CrossRef PubMed.
-
A. E. Douglas, The symbiotic habit, Princeton University Press, 2021 Search PubMed.
- G. Chomicki, E. T. Kiers and S. S. Renner, Annu. Rev. Ecol. Evol. Syst., 2020, 51, 409–432 CrossRef.
- G. C. diCenzo, M. Tesi, T. Pfau, A. Mengoni and M. Fondi, Nat. Commun., 2020, 11, 2574 CrossRef CAS PubMed.
- A. Satjarak, G. K. Golinski, M. T. Trest and L. E. Graham, Sci. Rep., 2022, 12, 6423 CrossRef CAS PubMed.
- M. K. Rich, N. Vigneron, C. Libourel, J. Keller, L. Xue, M. Hajheidari, G. V. Radhakrishnan, A. Le Ru, S. I. Diop, G. Potente, E. Conti, D. Duijsings, A. Batut, P. Le Faouder, K. Kodama, J. Kyozuka, E. Sallet, G. Bécard, M. Rodriguez-Franco, T. Ott, J. Bertrand-Michel, G. E. D. Oldroyd, P. Szövényi, M. Bucher and P.-M. Delaux, Science, 2021, 372, 864–868 CrossRef CAS.
- G. C. Drew, E. J. Stevens and K. C. King, Nat. Rev. Microbiol., 2021, 19, 623–638 CrossRef CAS PubMed.
- F. Zélé, S. Magalhães, S. Kéfi and A. B. Duncan, Nat. Commun., 2018, 9, 4869 CrossRef PubMed.
- A. Kouzuma, S. Kato and K. Watanabe, Front. Microbiol., 2015, 6, 477 Search PubMed.
- B. E. Morris, R. Henneberger, H. Huber and C. Moissl-Eichinger, FEMS Microbiol. Rev., 2013, 37, 384–406 CrossRef CAS PubMed.
-
J. F. White Jr and M. S. Torres, Defensive mutualism in microbial symbiosis, CRC Press, 2009 Search PubMed.
- K.-H. Nguyen, M. Chollet-Krugler, N. Gouault and S. Tomasi, Nat. Prod. Rep., 2013, 30, 1490–1508 RSC.
- K. Scherlach and C. Hertweck, Nat. Prod. Rep., 2018, 35, 303–308 RSC.
- N. Adnani, S. R. Rajski and T. S. Bugni, Nat. Prod. Rep., 2017, 34, 784–814 RSC.
- E. B. Van Arnam, C. R. Currie and J. Clardy, Chem. Soc. Rev., 2018, 47, 1638–1651 RSC.
- K. Aschheim, Nat. Biotechnol., 2012, 30, 60 Search PubMed.
- J. M. Crawford and J. Clardy, Chem. Commun., 2011, 47, 7559–7566 RSC.
- J. Piel, Nat. Prod. Rep., 2009, 26, 338–362 RSC.
- J. Piel, Curr. Med. Chem., 2006, 13, 39–50 CrossRef CAS PubMed.
- E. W. Schmidt, Nat. Chem. Biol., 2008, 4, 466–473 CrossRef CAS PubMed.
- A. Deveau, G. Bonito, J. Uehling, M. Paoletti, M. Becker, S. Bindschedler, S. Hacquard, V. Hervé, J. Labbé, O. A. Lastovetsky, S. Mieszkin, L. J. Millet, B. Vajna, P. Junier, P. Bonfante, B. P. Krom, S. Olsson, J. D. van Elsas and L. Y. Wick, FEMS Microbiol. Rev., 2018, 42, 335–352 CrossRef CAS PubMed.
- K. Scherlach and C. Hertweck, Annu. Rev. Microbiol., 2020, 74, 267–290 CrossRef CAS PubMed.
- T. E. Pawlowska, M. L. Gaspar, O. A. Lastovetsky, S. J. Mondo, I. Real-Ramirez, E. Shakya and P. Bonfante, Annu. Rev. Phytopathol., 2018, 56, 289–309 CrossRef CAS PubMed.
- P. Bonfante and A. Desiro, ISME J., 2017, 11, 1727–1735 CrossRef PubMed.
- L. P. Partida-Martinez, S. Monajembashi, K.-O. Greulich and C. Hertweck, Curr. Biol., 2007, 17, 773–777 CrossRef CAS.
- S. J. Mondo, O. A. Lastovetsky, M. L. Gaspar, N. H. Schwardt, C. C. Barber, R. Riley, H. Sun, I. V. Grigoriev and T. E. Pawlowska, Nat. Commun., 2017, 8, 1–9 CrossRef CAS.
- Y. Takashima, Y. Degawa, T. Nishizawa, H. Ohta and K. Narisawa, Microbes Environ., 2020, 35, ME19167 Search PubMed.
- J. P. Shaffer, J. M. U'Ren, R. E. Gallery, D. A. Baltrus and A. E. Arnold, Front. Microbiol., 2017, 8, 350 Search PubMed.
- A. Salvioli, S. Ghignone, M. Novero, L. Navazio, F. Venice, P. Bagnaresi and P. Bonfante, ISME J., 2016, 10, 130–144 CrossRef CAS PubMed.
- L. P. Partida-Martinez and C. Hertweck, Nature, 2005, 437, 884–888 CrossRef CAS PubMed.
- H. Büttner, S. P. Niehs, K. Vandelannoote, Z. Cseresnyés, B. Dose, I. Richter, R. Gerst, M. T. Figge, T. P. Stinear, S. J. Pidot and C. Hertweck, Proc. Natl. Acad. Sci. U. S. A., 2021, 118, e2110669118 CrossRef PubMed.
- Y. Takashima, K. Seto, Y. Degawa, Y. Guo, T. Nishizawa, H. Ohta and K. Narisawa, Microbes Environ., 2018, 33, 417–427 CrossRef PubMed.
- K. A. Dekker, R. J. Aiello, H. Hirai, T. Inagaki, T. Sakakibara, Y. Suzuki, J. F. Thompson, Y. Yamauchi and N. Kojima, J. Antibiot., 1998, 51, 14–20 CrossRef CAS PubMed.
- F. J. Ortíz-López, M. C. Monteiro, V. González-Menéndez, J. R. Tormo, O. Genilloud, G. F. Bills, F. Vicente, C. Zhang, T. Roemer, S. B. Singh and F. Reyes, J. Nat. Prod., 2015, 78, 468–475 CrossRef.
- S. E. Helaly, S. Ashrafi, R. B. Teponno, S. Bernecker, A. A. Dababat, W. Maier and M. Stadler, J. Nat. Prod., 2018, 81, 2228–2234 CrossRef CAS PubMed.
- J. E. Hochlowski, P. Hill, D. N. Whittern, M. H. Scherr, R. R. Rasmussen, S. A. Dorwin and J. B. McAlpine, J. Antibiot., 1994, 47, 528–535 CrossRef CAS PubMed.
- T. Bunyapaiboonsri, S. Yoiprommarat, R. Suntivich, S. Preedanon, S. Komwijit, T. Teerawatananond and J. Sakayaroj, Tetrahedron, 2020, 76, 131497 CrossRef CAS.
- C. C. C. R. De Carvalho and M.-J. Caramujo, Molecules, 2014, 19, 5570–5598 CrossRef PubMed.
- K. Blin, S. Shaw, A. M. Kloosterman, Z. Charlop-Powers, G. P. van Wezel, M. H. Medema and T. Weber, Nucleic Acids Res., 2021, 49, W29–W35 CrossRef CAS PubMed.
- M. J. Figueras, R. Beaz-Hidalgo, M. J. Hossain and M. R. Liles, Genome Announc., 2014, 2, e00927 Search PubMed.
- T. Stachelhaus, H. D. Mootz and M. A. Marahiel, Chem. Biol., 1999, 6, 493–505 CrossRef CAS.
- M. A. Marahiel, T. Stachelhaus and H. D. Mootz, Chem. Rev., 1997, 97, 2651–2674 CrossRef CAS.
- H. Kries, D. L. Niquille and D. Hilvert, Chem. Biol., 2015, 22, 640–648 CrossRef CAS PubMed.
- T. K. Shishido, J. Jokela, D. P. Fewer, M. Wahlsten, M. F. Fiore and K. Sivonen, ACS Chem. Biol., 2017, 12, 2746–2755 CrossRef CAS.
- S. C. Wenzel, P. Meiser, T. M. Binz, T. Mahmud and R. Müller, Angew. Chem., Int. Ed., 2006, 45, 2296–2301 CrossRef CAS PubMed.
- L. Du, C. Sánchez, M. Chen, D. J. Edwards and B. Shen, Chem. Biol., 2000, 7, 623–642 CrossRef CAS.
- B. I. Khayatt, L. Overmars, R. J. Siezen and C. Francke, PLoS One, 2013, 8, e62136 CrossRef CAS PubMed.
- K. Bloudoff and T. M. Schmeing, Biochim. Biophys. Acta, Proteins Proteomics, 2017, 1865, 1587–1604 CrossRef CAS.
- C. J. Balibar, F. H. Vaillancourt and C. T. Walsh, Chem. Biol., 2005, 12, 1189–1200 CrossRef CAS.
- M. P. Smith, T. R. Laws, T. P. Atkins, P. C. Oyston, D. I. de Pomerai and R. W. Titball, FEMS Microbiol. Lett., 2002, 210, 181–185 CrossRef CAS PubMed.
- K. Arima, A. Kakinuma and G. Tamura, Biochem. Biophys. Res. Commun., 1968, 31, 488–494 CrossRef CAS PubMed.
- J. Kroiss, M. Kaltenpoth, B. Schneider, M. G. Schwinger, C. Hertweck, R. K. Maddula, E. Strohm and A. Svatos, Nat. Chem. Biol., 2010, 6, 261–263 CrossRef CAS PubMed.
- M. J. Ek-Ramos, R. Gomez-Flores, A. A. Orozco-Flores, C. Rodríguez-Padilla, G. González-Ochoa and P. Tamez-Guerra, Front. Microbiol., 2019, 10, 463 CrossRef PubMed.
- P. N. Leão, A. R. Pereira, W.-T. Liu, J. Ng, P. A. Pevzner, P. C. Dorrestein, G. M. König, V. M. Vasconcelos and W. H. Gerwick, Proc. Natl. Acad. Sci. U. S. A., 2010, 107, 11183–11188 CrossRef.
- M. Rust, E. J. N. Helfrich, M. F. Freeman, P. Nanudorn, C. M. Field, C. Ruckert, T. Kundig, M. J. Page, V. L. Webb, J. Kalinowski, S. Sunagawa and J. Piel, Proc. Natl. Acad. Sci. U. S. A., 2020, 117, 9508–9518 CrossRef CAS.
- S. Schmidt, S. Kildgaard, H. Guo, C. Beemelmanns and M. Poulsen, Nat. Prod. Rep., 2022, 39, 231–248 RSC.
- I. Schoenian, M. Spiteller, M. Ghaste, R. Wirth, H. Herz and D. Spiteller, Proc. Natl. Acad. Sci. U. S. A., 2011, 108, 1955–1960 CrossRef PubMed.
- L. V. Flórez, K. Scherlach, P. Gaube, C. Ross, E. Sitte, C. Hermes, A. Rodrigues, C. Hertweck and M. Kaltenpoth, Nat. Commun., 2017, 8, 15172 CrossRef PubMed.
- I. Richter, S. Radosa, Z. Cseresnyés, I. Ferling, H. Büttner, S. P. Niehs, R. Gerst, K. Scherlach, M. T. Figge, F. Hillmann and C. Hertweck, mBio, 2022, e01440 Search PubMed.
- M. Jenner, X. Jian, Y. Dashti, J. Masschelein, C. Hobson, D. M. Roberts, C. Jones, S. Harris, J. Parkhill, H. A. Raja, N. H. Oberlies, C. J. Pearce, E. Mahenthiralingam and G. L. Challis, Chem. Sci., 2019, 10, 5489–5494 RSC.
- L. P. Partida-Martinez, C. Flores de Looß, K. Ishida, M. Ishida, M. Roth, K. Buder and C. Hertweck, Appl. Environ. Microbiol., 2007, 73, 793–797 CrossRef CAS PubMed.
- B. Dose, S. P. Niehs, K. Scherlach, L. V. Flórez, M. Kaltenpoth and C. Hertweck, ACS Chem. Biol., 2018, 13, 2414–2420 CrossRef CAS PubMed.
- S. P. Niehs, B. Dose, K. Scherlach, M. Roth and C. Hertweck, ChemBioChem, 2018, 19, 2167–2172 CrossRef CAS PubMed.
- S. P. Niehs, K. Scherlach and C. Hertweck, Org. Biomol. Chem., 2018, 16, 8345–8352 RSC.
- J. E. Spraker, L. M. Sanchez, T. M. Lowe, P. C. Dorrestein and N. P. Keller, ISME J., 2016, 10, 2317–2330 CrossRef CAS PubMed.
- C. Almeida, C. S. Pereira, V. Gonzalez-Menendez, G. Bills, J. Pascual, M. Sanchez-Hidalgo, S. Kehraus and O. Genilloud, Appl. Environ. Microbiol., 2018, 84, e00660 CrossRef CAS.
- S. P. Niehs, B. Dose, S. Richter, S. J. Pidot, H. M. Dahse, T. P. Stinear and C. Hertweck, Angew. Chem., Int. Ed., 2020, 59, 7766–7771 CrossRef CAS PubMed.
- L. Kalan and G. D. Wright, Expert Rev. Mol. Med., 2011, 13, e5 CrossRef PubMed.
- E. J. Hasenoehrl, T. J. Wiggins and M. Berney, Front. Cell. Infect. Microbiol., 2021, 10, 611683 CrossRef PubMed.
- Z. A. Shyr, Y.-S. Cheng, D. C. Lo and W. Zheng, Drug Discovery Today, 2021, 26, 2367–2376 CrossRef CAS PubMed.
- J. Lehár, A. S. Krueger, W. Avery, A. M. Heilbut, L. M. Johansen, E. R. Price, R. J. Rickles, G. F. Short 3rd, J. E. Staunton, X. Jin, M. S. Lee, G. R. Zimmermann and A. A. Borisy, Nat. Biotechnol., 2009, 27, 659–666 CrossRef.
- M. Cokol, H. N. Chua, M. Tasan, B. Mutlu, Z. B. Weinstein, Y. Suzuki, M. E. Nergiz, M. Costanzo, A. Baryshnikova and G. Giaever, Mol. Syst. Biol., 2011, 7, 544 CrossRef PubMed.
- A. León-Buitimea, C. R. Garza-Cárdenas, J. A. Garza-Cervantes, J. A. Lerma-Escalera and J. R. Morones-Ramírez, Front. Microbiol., 2020, 11, 1669 CrossRef.
- B. Huang, J. Li, Q. Wang, M. Guo, D. Yan, W. Fang, Z. Ren, Q. Wang, C. Ouyang and Y. Li, PLoS One, 2018, 13, e0188245 CrossRef PubMed.
- C. R. Silva, A. L. Lifschitz, S. R. Macedo, N. R. Campos, M. Viana-Filho, A. C. Alcântara, J. G. Araújo, L. M. Alencar and L. M. Costa-Junior, Vet. Parasitol., 2021, 290, 109345 CrossRef CAS PubMed.
- H. Abdelnabby, Z. Hu, H. Wang and X. Zhang, J. Pest Sci., 2018, 91, 203–218 CrossRef.
- F. Nicolay, A. Harder, G. von Samson-Himmelstjerna and H. Mehlhorn, Parasitol. Res., 2000, 86, 982–992 CrossRef CAS PubMed.
- A. H. Valente, M. de Roode, M. Ernst, M. Peña-Espinoza, L. Bornancin, C. S. Bonde, M. Martínez-Valladares, S. Ramünke, J. Krücken, H. T. Simonsen, S. M. Thamsborg and A. R. Williams, Int. J. Parasitol.: Drugs Drug Resist., 2021, 15, 105–114 CAS.
- M. A. Helal, A. M. Abdel-Gawad, O. M. Kandil, M. M. Khalifa, G. W. Cave, A. A. Morrison, D. J. Bartley and H. M. Elsheikha, Pathogens, 2020, 9, 740 CrossRef CAS PubMed.
- M. Liu, P. Kipanga, A. H. Mai, I. Dhondt, B. P. Braeckman, W. De Borggraeve and W. Luyten, Int. J. Parasitol., 2018, 48, 833–844 CrossRef CAS PubMed.
- F. Rivardo, M. G. Martinotti, R. J. Turner and H. Ceri, Int. J. Antimicrob. Agents, 2011, 37, 324–331 CrossRef CAS PubMed.
- K. Joshi-Navare and A. Prabhune, BioMed Res. Int., 2013, 2013, 512595 Search PubMed.
- H. L. Lydon, N. Baccile, B. Callaghan, R. Marchant, C. A. Mitchell and I. M. Banat, Antimicrob. Agents Chemother., 2017, 61, e02547 CrossRef CAS PubMed.
|
This journal is © The Royal Society of Chemistry 2023 |
Click here to see how this site uses Cookies. View our privacy policy here.