DOI:
10.1039/D3SC00038A
(Edge Article)
Chem. Sci., 2023,
14, 5405-5414
Rational synthesis of elusive organic–inorganic hybrid metal-oxo clusters: formation and post-functionalization of hexavanadates†
Received
3rd January 2023
, Accepted 22nd April 2023
First published on 25th April 2023
Abstract
Paving the way towards new functional materials relies increasingly on the challenging task of forming organic–inorganic hybrid compounds. In that regard, discrete atomically-precise metal-oxo nanoclusters have received increasing attention due to the wide range of organic moieties that can be grafted onto them through functionalization reactions. The Lindqvist hexavanadate family of clusters, such as [V6O13{(OCH2)3C-R}2]2− (V6-R), is particularly interesting due to the magnetic, redox, and catalytic properties of these clusters. However, compared to other metal-oxo cluster types, V6-R clusters have been less extensively explored, which is mainly due to poorly understood synthetic challenges and the limited number of viable post-functionalization strategies. In this work, we present an in-depth investigation of the factors that influence the formation of hybrid hexavanadates (V6-R HPOMs) and leverage this knowledge to develop [V6O13{(OCH2)3CNHCOCH2Cl}2]2− (V6-Cl) as a new and tunable platform for the facile formation of discrete hybrid structures based on metal-oxo clusters in relatively high yields. Moreover, we showcase the versatility of the V6-Cl platform through its post-functionalization via nucleophilic substitution with various carboxylic acids of differing complexity and with functionalities that are relevant in multiple disciplines, such as supramolecular chemistry and biochemistry. Hence, V6-Cl was shown to be a straightforward and versatile starting point for the formation of functional supramolecular structures or other hybrid materials, thereby enabling their exploration in various fields.
Introduction
Over time, the ability to synthesize increasingly complex chemical structures has begun to blur the line between organic and inorganic materials. The formation of organic–inorganic hybrid materials that synergistically combine the properties of both organic and inorganic components has attracted attention especially for the development of functional materials.1 Therefore, in recent years, metal-oxo clusters have emerged as valuable building-blocks for the formation of a variety of discrete organic–inorganic hybrid structures. Of the multiple possible hybrid structures, hybrid polyoxometalates (HPOMs) have particularly garnered significant interest due to their applications in catalysis, materials science, energy storage, medicine, and supramolecular chemistry.2–8 HPOMs are a subclass of a diverse family of discrete anionic metal-oxo clusters, commonly known as polyoxometalates (POMs), that can be covalently grafted with a wide range of organic molecules through the replacement of a metal centre by organophosphorus, organosilicon, organogermanium or organotin species or through replacement of the oxo ligands by alkoxides or amines.2,9 Such functionalization of metal-oxo clusters with various moieties has already proven beneficial, for example, in enhancing their catalytic activity and efficiency.10–12 Additionally, organic derivatization has been recently demonstrated as a valuable strategy for addressing important challenges in renewable energy and energy storage.13,14 Moreover, these hybrid clusters can be regarded as analogues for MOx hybrid materials such as Metal Organic Frameworks (MOFs) and functionalized metal oxide nanoparticles or surfaces, due to similarities in their structure and electronic delocalization.15 In fact, several MOFs based on HPOM building units have been reported showing the close connection between such hybrid frameworks and HPOMs.16–19 However, the discrete atomically-precise structures of HPOMs and their solubility in many different solvents make them easier to study, thereby providing insights about metal-oxo based materials in general. Therefore, the synthetic challenges faced in the formation of MOx hybrid materials are more easily tackled at the scale of HPOMs. Hence, the synthesis of more complex novel HPOMs is likely to drive progress in many different fields.
Although hybrid metal-oxo clusters have been attracting increasing attention in recent years, the factors that influence their formation in solution are still not well understood. As a result, there is a rather limited selection of HPOMs that can be easily functionalized with various organic moieties. This is particularly the case for the Lindqvist hexavanadate HPOMs, such as [V6O13{(OCH2)3C-R}2]2− (V6-R), which due to their interesting chemical and redox properties have been investigated in several fields including catalysis,10,15 memory devices,20 energy conversion/storage,21 supramolecular self-assembly and host–guest chemistry, among others.22,23 Moreover, these hexavanadate HPOMs have a great structural versatility, since they can be mono-, bis-, tris- or tetra-functionalized with triol ligands, depending on the composition and oxidation state of the metal centres.24 However, despite V6-R HPOMs being one of the first examples of HPOMs to be reported,25 their synthesis and post-functionalization remain significantly less explored compared to other metal-oxo cluster types.24,27,28 The relatively limited literature available on V6-R HPOMs is likely due to the poor yields often obtained during their synthesis and the scarce number of V6-R HPOMs that can be easily derivatized with a wide range of organic species. Hence, elucidating the formation mechanism of such clusters is essential to address the issues typically encountered during their synthesis.
In general, the synthesis of metal-oxo hybrids with complex organic moieties attached onto the inorganic core is often achieved by starting from a relatively simple building unit with a reactive terminal group that can then be modified through a post-functionalization reaction.27 Therefore, [V6O13{(OCH2)3CCH2OH}2]2− (V6-OH), which can be easily obtained from decavanadate ([H3V10O28]3−; V10) and pentaerythritol ((HOCH2)3CCH2OH),29,30 is typically used for forming more complex V6-R HPOMs with various organic moieties through esterification reactions with carboxylic acids and acid anhydrides, but it is not reactive towards many substrates (Scheme 1).31,32 Alternatively, [V6O13{(OCH2)3CNH2}2]2− (V6-NH2) can be used as a post-functionalization platform with a more reactive amine terminal group. However, its synthesis from V10 and tris(hydroxymethyl)aminomethane ((HOCH2)3CNH2; Tris-NH2) typically results in very low yields (Scheme 1),22,33,34 in contrast to other metal-oxo clusters that have been successfully functionalized with Tris-NH2.26,35 Due to this limitation, pre-functionalization of Tris-NH2 is sometimes used for the direct formation of V6-R HPOMs, but this also often results in relatively low yields.28 Consequently, there is a need for post-functionalization platforms that can be employed to incorporate various organic functionalities onto V6-R HPOMs in high yields, thereby driving the formation of new hybrid materials and expanding their potential applications. To achieve this goal, a deeper understanding of the factors that affect the synthesis and modification of these hybrid metal-oxo clusters is still necessary. Hence, by investigating the key factors in the synthesis of V6-R HPOMs, a novel post-functionalization platform was developed. Moreover, this new HPOM was functionalized with multiple carboxylic acids of varying complexity to demonstrate the versatility of this platform in making the formation of hybrid polyoxovanadates more accessible compared to existing platforms.
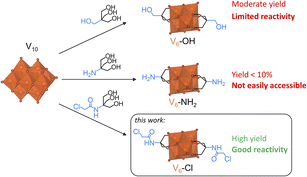 |
| Scheme 1 Synthesis of hexavanadate post-functionalization platforms starting from V10 and triol ligands with reactive terminal groups. | |
Results and discussion
The low yields often obtained in the synthesis of V6-R HPOMs are typically attributed to the ease of reduction of the V5+ centres.22,33,34 However, to the extent of our knowledge, this has not been experimentally confirmed. Nevertheless, previously reported literature procedures suggest that the solvent and the nature of the functional groups on the triol ligand have a significant influence on the outcome of the reaction.34,36,37 For example, literature reports show that V6-OH can be easily synthesized in acetonitrile (ACN) and water while V6-NH2 can only be obtained directly in dimethylacetamide (DMA) and even in DMA the yield is typically less than 10% unless protecting groups are used.22,29,30,33,34 Therefore, in order to understand what happens in solution during the synthesis of V6-R HPOMs, we set out to study the reactions between V10 and different triol ligands by multinuclear NMR, IR and UV-vis-NIR spectroscopy as well as ESI-MS. Furthermore, the reaction of V10 with (HOCH2)3CNHCOCH2Cl (Tris-Cl) was investigated in detail, since this triol ligand can be easily synthesized in high yields at the gram-scale and its highly electrophilic chloroacetamide functionality can be exploited for nucleophilic substitution. As a result, the Tris-Cl ligand can be leveraged for post-functionalization with a wide variety of carboxylic acids, amines and thiols, as has been previously demonstrated by our group with other hybrid metal-oxo clusters.38–40 Moreover, the high electrophilicity of the chloroacetamide carbon, and the SN2 mechanism favoured under polar aprotic conditions, allow for easier substitution reactions with relatively bulky or less nucleophilic substrates than with V6-OH and its derivatives. Additionally, the insights gained from following the reaction of V10 with various triol ligands were used to optimize the procedure for the synthesis of TBA2[V6O13{(OCH2)3CNHCOCH2Cl}2] (V6-Cl) as a novel post-functionalization platform formed from Tris-Cl and V10 (Scheme 1).
Elucidating the impact of ligand functionalities
Following changes in the speciation of reaction mixtures of V10 in the absence or in the presence of three triol ligands by multinuclear NMR, IR, UV-vis-NIR and ESI-MS gave distinct results depending on the triol ligand: (HOCH2)3CCH3 (Tris-CH3), Tris-NH2, or Tris-Cl. This demonstrates the important role of the ligands and the nature of their terminal group as critical factors in determining the outcome of the reaction (Scheme 2). Although Tris-CH3 and Tris-NH2 have very similar structures, simply switching the methyl group for an amine had a major effect on the speciation of vanadates in solution. With Tris-CH3, the desired hexavanadate structure, [V6O13{(OCH2)3CCH3}2]2− (V6-CH3), was observed to gradually form over time from the appearance of characteristic peaks in NMR and ESI-MS spectra while the peaks due to V10 disappeared (Fig. S1, S3, S14 and Table S6†).29,41 In contrast, when V10 was reacted with Tris-NH2 several peaks appeared in the 51V NMR spectrum within 0.5 h of heating (Fig. S1†), indicating that multiple vanadate species formed, with the main species being [V12O32]4− (V12) and [V13O34]3− (V13).42,43
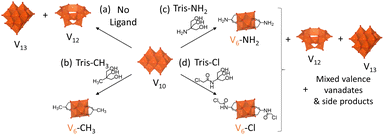 |
| Scheme 2 Speciation of V10 in ACN after heating at 80 °C for 0–24 h: (a) alone or with (b) Tris-CH3, (c) Tris-NH2 or (d) Tris-Cl. | |
The formation of V12 and V13 in the presence of Tris-NH2 may be due to the poor solubility of Tris-NH2 in ACN (Table S2†), especially in comparison with Tris-CH3, since the conversion of V10 into V12 and V13 was also observed when V10 was heated at 80 °C on its own in ACN (Scheme 2 and Fig. S1, S2†). Furthermore, V12 eventually became the main species in solution based on 51V NMR (Fig. S1†) and on negative mode ESI-MS (Fig. S14 and Table S5†). Similarly, the IR spectrum of V10 alone after heating gave rise to characteristic peaks of V12 between 1200 and 400 cm−1 that were also observed after heating with Tris-NH2 (Fig. S11†).43 Therefore, it is likely that in the reaction with Tris-NH2, only a small amount of the triol was present in solution at any given time, which reacted with V10 to form other vanadate species that gave rise to several peaks around 5 ppm in the 1H NMR spectrum (Fig. S4†) along with additional peaks in the 51V NMR spectrum. These are likely caused by Tris-NH2 reacting with V10 through both the –OH and –NH2 groups, since reacting with just the –OH groups would result in the formation of the desired hexavanadate as observed for Tris-CH3. In addition, over time, the peaks observed by 51V NMR gradually disappeared, except for a peak at −498 ppm, which may correspond to V6-NH2. However, this peak is relatively weak. Therefore, together with the IR and ESI-MS spectra (Fig. S14 and Table S7†), this suggests that V6-NH2 is only present in low concentration after 120 h.
In contrast, the reaction of V10 with Tris-Cl did not give rise to observable peaks (Fig. S1†) corresponding to V12 and V13 in the 51V NMR spectra, which may be due to the higher solubility of Tris-Cl in ACN with respect to Tris-NH2 (Table S2†). Instead, two broad peaks were observed to appear at −228 and −294 ppm in the 51V NMR spectrum of the reaction mixture (Fig. S1†), which correspond to V environments that are more deshielded than expected for fully oxidized vanadates with oxo or alkoxo ligands.44 Hence, these new peaks may be due to paramagnetic shifting of V5+ centres in mixed valence vanadates or due to coordination of the ligand to V5+ centres through nitrogen.44 Furthermore, as with Tris-NH2, the reactions of vanadate species with the Tris-Cl ligand resulted in the gradual disappearance of most peaks observed by 51V NMR except for two weak peaks: one at −364 ppm, belonging to VO2Cl2− formed through the decomposition of Tris-Cl, and another at around −498 ppm, likely due to V6-Cl present in low concentration (Fig. S1†).44 Likewise, the presence of V6-Cl in low concentration together with side-products after 120 h could be observed by 1H NMR (Fig. S5†). These changes observed by NMR can be attributed to reduction of V5+ centres to form mixed valence species.
The formation of mixed valence vanadates in the presence of Tris-NH2 or Tris-Cl is confirmed by the observed change in colour of the solutions over time from orange/yellow (typical of V5+) to dark green, which is indicative of the reduction of some V5+ centres to form a mixture of V5+ and V4+.16,45 This was confirmed by the appearance of a characteristic peak in the absorbance spectrum at around 900 nm due to an Intervalence Charge Transfer (IVCT) between V4+ and V5+ (Fig. 1 and Tables S3, S4†). Since the appearance of this IVCT broad peak is not as noticeable for V10 alone or in the presence of Tris-CH3 (Fig. 1 and S10†), the reduction of V5+ is most likely due to N–H⋯O–V interactions. Such N–H⋯O–V interactions have indeed been recently reported in crystal structures of V10 with Tris-NH2.45 Furthermore, a similar reduction process has been proposed to result in the photoinduced reduction of alkylammonium salts of molybdates and is likely responsible for the reduction of NH4VO3 by the NH4+ counterion during its thermal decomposition.46–48
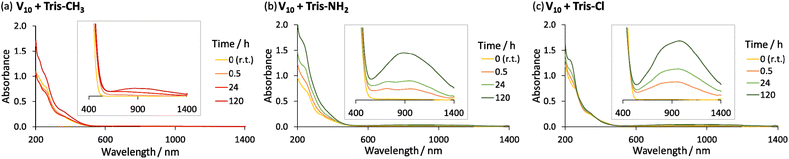 |
| Fig. 1 UV-vis-NIR absorbance spectra of V10 with (a) Tris-CH3, (b) Tris-NH2, or (c) Tris-Cl after 0 h at room temperature (r.t.) and after 0.5, 24, and 120 h at 80 °C, with the broad IVCT peak shown in the insert. | |
Reduction of V5+ may take place through the transfer of the N–H proton onto the oxo ligands of V10 or other vanadates that may form in solution.34 This likely occurs through a similar process to the formation of mixed valence or fully reduced V6-R HPOMs with protonated μ2-O ligands in the presence of hydrazine derivatives and organic acids, which has been reported to involve a proton-coupled electron transfer (PCET).15,29,34,49 However, since the structure of the side-products could not be identified, the exact nature of the reduction mechanism could not be unambiguously determined. Nevertheless, it can be concluded that the main product for the reaction of Tris-Cl and Tris-NH2 with V10 in ACN is not the desired V6-R HPOMs because of side-reactions giving rise to reduced vanadate species. This is evidenced by ESI-MS and IR spectroscopy (Fig. S11, S12, S14 and Tables S7, S8†), which after 120 h at 80 °C mainly showed peaks that did not correspond to the desired hexavanadates. Moreover, this indicates that the yield of V6-R HPOMs depends highly on how the speciation of vanadates is affected by the ligand's availability in solution and the reactivity of its functional groups.
Investigating the role of the solvent
Since DMA has also been previously reported as a solvent in optimized procedures,36,37 in this work the impact of the solvent on the formation of V6-Cl was investigated further. In doing so, the use of DMA as the solvent for the synthesis of V6-Cl was determined to be crucial for obtaining the product in relatively high yields, since the attempted synthesis in ACN or in water, following the typical procedures used for the formation of hexavanadates, resulted in many side products, which hindered the isolation of V6-Cl.29,30 The differences in the reaction of V10 with Tris-Cl in ACN vs. in DMA can be seen from the 1H and 51V NMR spectra of aliquots obtained over 20 h, which showed more intense peaks corresponding to V6-Cl when the reaction was conducted in DMA (Fig. 2 and S7†). This means that the formation of V6-Cl is more favourable in DMA, which may be in part due to the higher solubility of Tris-Cl in this solvent (Table S2†). Furthermore, when V10 is heated at 80 °C in DMA without any triol ligands, the speciation is quite different from that in ACN (Fig. S6†). While the V12 structure is highly favoured in ACN, V13 is the main species in DMA along with multiple other vanadate species of lower nuclearity, ranging from V1 to V5, which gradually disappear over time.44 This suggests that the vanadate structures are more labile and prone to interconversion in DMA than in ACN, generating a dynamic library of interconverting vanadate building blocks, which may also facilitate the formation of hexavanadates in DMA.
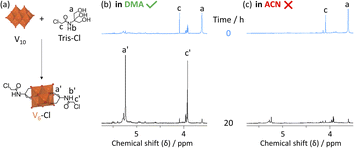 |
| Fig. 2 (a) Synthesis of V6-Cl from V10 and Tris-Cl along with the corresponding 1H NMR spectra of aliquots of the reaction mixture after heating at 80 °C for 0 and 20 h in (b) DMA or (c) ACN. All spectra were acquired for solutions in ACN-d3 of the precipitate formed by addition of excess of diethyl ether to the aliquot. | |
It was also observed that changing the solvent had an impact on the ability of V5+ centres to be reduced. The cyclic voltammogram (CV) of V6-Cl in either ACN or DMA (Fig. 3(a)) shows a similar reversible reduction/oxidation wave due to the V5+/V4+ redox couple in both solvents, but the half-wave potential (E1/2) is more negative in DMA compared to in ACN: −1.01 and −0.80 V vs. Fc/Fc+, respectively.50 Similarly, V6-CH3 gives rise to reversible reduction/oxidation waves with E1/2 of −1.07 and −0.92 V vs. Fc/Fc+ in DMA and in ACN, respectively (Fig. S18†). Hence, the reduction of V5+ occurs more easily in ACN. This solvent-induced shift in E1/2 is likely due to the higher Lewis acidity of ACN, as reflected by the Gutmann acceptor number of ACN (18.9) being higher than that of DMA (13.6).51 Furthermore, these results are in agreement with the previously reported trend in the redox potential of tungstate POMs in different solvents.52,53 However, the solvent-induced shift in E1/2 is greater for V6-Cl (215 mV) than for V6-CH3 (150 mV), which may be linked to the N–H⋯O–V interactions observed in the crystal structure obtained by crystallization in ACN (Fig. 3(c)) since protonation of oxo ligands in the vanadate clusters has been shown to result in shifts towards lower potentials.29 In contrast, the crystal structure of a similar V6-R HPOM with an amide group obtained by Bamba et al. in DMA shows that DMA forms H-bonding interactions between its C
O group and the –NH group of the ligand, thereby preventing N–H⋯O–V interactions from occurring.54 Therefore, reduction of V5+ induced by transfer of the proton from the ligand is less likely to occur in DMA than in ACN, making it overall a more suitable solvent.
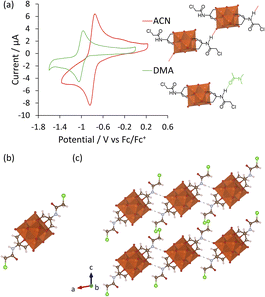 |
| Fig. 3 (a) CV of 0.5 mM V6-Cl in ACN vs. in DMA with 0.1 M TBA-PF6 as the electrolyte (100 mV s−1). (b) Structural representation of V6-Cl viewed along the crystallographic b axis and (c) crystal packing of V6-Cl into a 1D H-bond network (H-bond distances shown as dashed grey lines). The counter ions were omitted from the structural representations for clarity. Hydrogen in white, carbon in brown, nitrogen in light blue, oxygen in red, chlorine in green and vanadium in orange. | |
Isolation and characterisation of V6-Cl
Through optimization of the synthesis and purification procedures based on the insights obtained by following the reactions of the triols, it was possible to obtain V6-Cl directly from V10 and Tris-Cl in relatively high yield after reacting in DMA at 80 °C under an argon atmosphere for 5 days. However, despite the synthesis of V6-Cl being more successful in DMA than in ACN, some side reactions resulting in the reduction of V5+ do still occur, as evidenced by the observed colour change of the solution. This is due to the formation of a mixture of the desired fully oxidized V6-Cl and mixed valence V6-Cl. Nevertheless, through the removal of DMA by rotary evaporation and the addition of a minimal amount of ACN, the mixed valence species were preferentially dissolved, forming a green solution, and the pure fully oxidized V6-Cl could be separated by centrifugation as an orange crystalline solid. Furthermore, the mixed valence V6-Cl in the green supernatant was successfully oxidized into the desired fully oxidized V6-Cl by the addition of 35 wt% H2O2. Additionally, washing with water as well as re-precipitation with ACN/Et2O and MeOH/Et2O allowed for further purification of the re-oxidized sample as described in the SI. Moreover, through this re-oxidation and purification procedure the yield was increased to 61%.
The composition of V6-Cl in high purity in the combination of the first fully oxidized sample and the re-oxidized sample was confirmed by ESI-MS, NMR, IR, and elemental analysis (Fig. 4). ESI-MS gave a peak at m/z = 1145.8 in negative mode, corresponding to V6-Cl with only one TBA counter cation ([V6-Cl + TBA]−; calcd. m/z = 1145.3) while in positive mode it gave a peak at m/z = 1630.1 that can be attributed to the POM with three TBA counter ions ([V6-Cl + 3TBA]+; calcd. m/z = 1630.2). Furthermore, 1H NMR showed a downfield shift from 3.57 ppm for Tris-Cl to 5.11 ppm for V6-Cl, corresponding to the –CH2O– group of the triol ligand becoming grafted onto the V6 POM core, while 51V NMR gave a single peak at −494 ppm that is characteristic of trans-functionalized hexavanadates.34,54 Furthermore, the peaks in the IR spectrum in the range 700–1000 cm−1 are characteristic of ν V
O and ν V–O–V vibrations of the V6 POM core, while the peaks at 1114 and 1063 cm−1 due to ν C-O vibrations confirm the successful attachment of the triol ligands.30,55 The exact structure of V6-Cl was further confirmed by single crystal X-ray diffraction (SC-XRD). V6-Cl crystallized in the triclinic space group
1 with half of one POM and one TBA cation in the asymmetric unit. The obtained crystal structure (Fig. 3(b)) shows a classical Lindqvist structure composed of six edge sharing {VO6} octahedra in an octahedral arrangement. Moreover, two Tris-Cl moieties are attached on opposite faces of the V6 POM core via three double-bridged oxygens (μ2-O) each. In addition, V6-Cl was observed to form a 1D H-bond network due to H-bonding interactions between the –NH group of the amide and a terminal oxygen of the V6 POM core with N⋯O distances of 2.91 Å (Fig. 3(c)). Overall, the crystal structure together with the complementary spectroscopic techniques unambiguously confirm the formation of this novel post-functionalization platform.
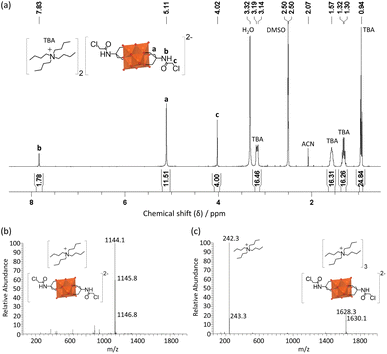 |
| Fig. 4 (a) 1H NMR spectrum of V6-Cl in DMSO-d6 as well as (b) negative mode and (c) positive mode ESI-MS spectra of V6-Cl in ACN. | |
Post-functionalization of V6-Cl
The potential of V6-Cl as a valuable post-functionalization platform was evaluated via nucleophilic substitution reactions with different carboxylic acids: valeric acid, phenylacetic acid, adamantanecarboxylic acid, and biotin (Scheme 3). These carboxylic acids were selected to showcase the applicability of this post-functionalization strategy to yield compounds with widely different functionalities. Furthermore, functionalities that are of interest in supramolecular chemistry were chosen since HPOMs have been shown to produce new dynamic supramolecular structures that often result in enhanced properties.8 Consequently, four novel V6-R HPOMs were synthesized from V6-Cl and the aforementioned carboxylic acids: TBA2[V6O13{(OCH2)3CNHCOCH2-R}2] where R = –OOC(CH2)3CH3 (V6-Val), –OOCCH2C6H5 (V6-Ph), –OOCC10H15 (V6-Ad), and –OOCC9H15N2OS (V6-Biot).
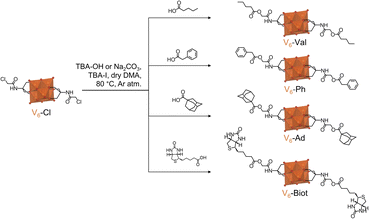 |
| Scheme 3 Post-functionalization of V6-Clvia nucleophilic substitution with valeric acid, phenylacetic acid, adamantanecarboxylic acid, and biotin to form V6-Val, V6-Ph, V6-Ad, and V6-Biot (top to bottom). | |
The nucleophilic substitution reactions were performed by mixing V6-Cl with tetrabutylammonium iodide (TBA-I), a carboxylic acid and a base in dry DMA for 2–5 h at 80 °C. Either Na2CO3 or tetrabutylammonium hydroxide (TBA-OH) were used as the base and both successfully promoted the nucleophilic substitution reaction. Na2CO3 was found to be more suitable for post-functionalization of V6-Cl since hydrolysis of the ester bond after post-functionalization was observed by ESI-MS if the solution was left stirring for too long when using TBA-OH (Fig. S15–S17†). As a result, the yield and the purity of the product were generally higher with Na2CO3 than with TBA-OH. For example, the synthesis of V6-Ad gave a yield of 42% with TBA-OH while with Na2CO3 the yield was 50%. Nevertheless, when using Na2CO3, a small amount of the Na salt of the HPOM or mixed salts were sometimes obtained, but these could be easily converted back to the desired TBA salt by the addition of excess tetrabutylammonium bromide (TBA-Br). Hence, once the reaction conditions were optimized, post-functionalization of V6-Cl resulted in yields of around 50% or higher overall.
It is worth noting that the post-functionalization of V6-Cl with all selected carboxylic acids was possible despite their differences in steric hindrance and functionality. However, the nature of the organic species being used can have an impact on the outcome of the reaction. This was especially noticeable during the synthesis of V6-Ph since the solution turned green due to the formation of mixed valence V6-Ph. Hence, in order to obtain the desired fully oxidized V6-Ph, an additional oxidation step with H2O2 was required. The higher propensity of V6-Ph towards reduction over the other V6-R HPOMs reported in this study is likely due to the ability of the aromatic ring to transfer electrons to the POM.56 Additionally, reduction of the V6 core may be favoured by the stabilising electron-withdrawing inductive effect of the aromatic group.50,57 This resulted in a slightly lower yield for V6-Ph compared to other V6-R HPOMs. In contrast, V6-Biot was successfully obtained in relatively high yield, even when using TBA-OH as the base. This is significant because the attempted post-functionalization of V6-OH with biotin via esterification – using N,N′-dicyclohexylcarbodiimide (DCC) as the coupling agent and 4-dimethylaminopyridine (DMAP) to promote the reaction – was unsuccessful, and the previously reported esterification with stearic acid only gave a relatively low yield (10%).31 This shows the limitations of V6-OH as a post-functionalization platform. Furthermore, modifications of the platform to improve its reactivity, such as the incorporation of an alkyne terminal group that has been reported to allow for the attachment of biotin in high yields via Huisgen 1,3-cycloaddition, are often not as straightforward as the single-step synthesis of Tris-Cl.55,58 Therefore, this highlights the versatility and accessibility of V6-Cl for the derivatization of hexavanadates with a wide range of different species to obtain new V6-R HPOMs in reasonable yields.
Successful post-functionalization of V6-Cl to form and isolate V6-Val, V6-Ph, V6-Ad, and V6-Biot was confirmed by 1H NMR (Fig. S8†) from the disappearance of the peak at around 12 ppm due to the –COOH environment and a downfield shift in the chemical shift of the –COCH2– group from 4 ppm to around 4.45 ppm due to the chloro (–Cl) functionality being substituted by the carboxylate (–OOC–). The chemical shift and integration of the other peaks in the spectra of each compound were also consistent with the attachment of the carboxylate moieties to both tripodal anchors on the V6 HPOM (Fig. S8 and S9†). Furthermore, 51V NMR (Fig. S8†) showed that the V6 POM core structure remained intact after the post-functionalization, giving rise to a single peak at around −495 ppm for all compounds. This was further confirmed from the characteristic peaks at around 700–1000 cm−1 in the IR spectra of the compounds due to ν V
O and ν V–O–V vibrations (Fig. S13†). Negative ion mode ESI-MS also showed peaks corresponding to the molecular ions of the POM alone (M2−) or with one TBA counter cation ([M + TBA]−) for each compound. Peaks in positive ion mode due to the POM with extra TBA counter cations ([M + 3TBA]+) were also observed for the POMs with molecular weights within the measurement range. Moreover, the crystal structure of V6-Val (Fig. 5), obtained from crystals formed by slow evaporation of a solution of V6-Val in CHCl3, matched that of V6-Cl with Cl replaced by the carboxylate. However, unlike V6-Cl, V6-Val crystallized in the monoclinic P21/c space group. Yet, V6-Val also formed a 1-D H-bond network due to H-bonding interactions between the –NH group of the amide bond and a terminal oxygen of the hexavanadate POM core with N⋯O distances of 2.88 Å (Fig. 5(b)). Furthermore, aside from having two TBA molecules per hexavanadate unit as expected, two chloroform molecules per hexavanadate unit were also present in the unit cell (Fig. 5(a)). Overall, this confirms the successful post-functionalization of V6-Cl with carboxylic acids.
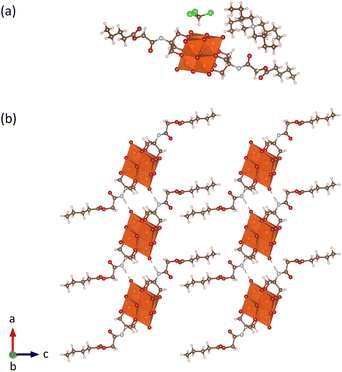 |
| Fig. 5 (a) Structural representation of V6-Val showcasing the relative position of the CHCl3 and TBA molecules with respect to V6-Val in the crystal structure (two of each per hexavanadate unit that are symmetrically equivalent). (b) Crystal packing of V6-Val into a 1D H-bond network (H-bond distances shown as dashed grey lines) viewed along the crystallographic b axis without showing the TBA counter ions and CHCl3 for clarity. Hydrogen in white, carbon in brown, nitrogen in light blue, oxygen in red, chlorine in green and vanadium in orange. | |
Conclusions
In this work, an in-depth investigation of the factors that affect the synthesis of hexavanadates was leveraged to develop V6-Cl as a novel post-functionalization platform. The study showed that the low yields often observed in the synthesis of hexavanadates are linked to the solution properties of the ligand and the reactivity of its functional groups, both of which affect the speciation of vanadates in solution. In general, the formation of a suitable post-functionalization platform requires a ligand with a reactive terminal group, however this may also cause undesired side reactions. Overall, the key factors influencing the synthesis of hybrid hexavanadate clusters were determined to be: (i) the reactivity of the terminal group of the organic ligand with respect to vanadate species in solution, (ii) the solubility of the organic ligand, (iii) the interactions of the solvent with the ligand, which can prevent side reactions, and (iv) the Lewis acidity of the solvent, which affects the ease of reduction of V5+. Controlling these factors was shown to be crucial for the facile, one-step synthesis of V6-Cl in relatively high yields from a decavanadate cluster and Tris-Cl, using DMA as the solvent. We also showed that V6-Cl could be easily post-functionalized with a wide range of carboxylic acids that differ significantly in composition and complexity. The formation of such (bio)organic hybrids with biomolecules, such as biotin, in particular may have potential for the development of antitumor, antiviral, anticancer and anti-amyloid agents,7,59–62 while adamantane derivatives could be used for drug carrier systems in combination with cyclodextrin, among many other possible applications.23,61,63–67 Furthermore, this post-functionalization strategy can be extended to functionalization with other organic moieties or even other inorganic clusters, resulting in novel hybrid metal-oxo clusters for potential use in catalysis, medicine and molecular magnetism.8,10,23,68–70 Hence, through the successful synthesis of V6-Cl as a novel, accessible, and versatile starting point for the formation of various hybrid structures the range of potential applications of polyoxovanadates can be expanded.
Data availability
Crystallographic data for compounds V6-Cl and V6-Val has been deposited at The Cambridge Crystallographic Data Centre (CCDC) and allocated the deposition numbers CCDC 2217991 and 2217992, respectively. All other data supporting the results discussed in this work is available within the paper and its ESI.†
Author contributions
D. E. S. M. and G. K. performed the synthesis and characterisation of all compounds with the assistance of M. A. M. K. V. H. solved the crystal structures. T. N. P.-V. and M. A. M. helped with the design and interpretation of the experiments. The manuscript was written through contributions of all authors. All authors have given approval to the final version of the manuscript.
Conflicts of interest
There are no conflicts to declare.
Acknowledgements
We thank KU Leuven and the Research Foundation Flanders (FWO) for funding. D. E. S. M. (1183021N), G. K. (1185522N) and M. A. M. (1279721N) thank the FWO for PhD and post-doctoral fellowships.
Notes and references
- C. Sanchez, P. Belleville, M. Popall and L. Nicole, Applications of advanced hybrid organic–inorganic nanomaterials: from laboratory to market, Chem. Soc. Rev., 2011, 40, 696–753 RSC.
- J. Zhang, Y. Huang, G. Li and Y. Wei, Recent advances in alkoxylation chemistry of polyoxometalates: From synthetic strategies, structural overviews to functional applications, Coord. Chem. Rev., 2019, 378, 395–414 CrossRef CAS.
- Y. Zhang, F. de Azambuja and T. N. Parac-Vogt, The forgotten chemistry of group(IV) metals: A survey on the synthesis, structure, and properties of discrete Zr(IV), Hf(IV), and Ti(IV) oxo clusters, Coord. Chem. Rev., 2021, 438, 213886 CrossRef CAS.
- S.-S. Wang and G.-Y. Yang, Recent Advances in Polyoxometalate-Catalyzed Reactions, Chem. Rev., 2015, 115, 4893–4962 CrossRef CAS PubMed.
-
Y.-F. Song, Polyoxometalate-Based Assemblies and Functional Materials, Springer International Publishing, Cham, 2018, vol. 176 Search PubMed.
- Y. Ji, L. Huang, J. Hu, C. Streb and Y.-F. Song, Polyoxometalate-functionalized nanocarbon materials for energy conversion, energy storage and sensor systems, Energy Environ. Sci., 2015, 8, 776–789 RSC.
- J. T. Rhule, C. L. Hill, D. A. Judd and R. F. Schinazi, Polyoxometalates in Medicine, Chem. Rev., 1998, 98, 327–358 CrossRef CAS PubMed.
- M. Stuckart and K. Y. Monakhov, Polyoxometalates as components of supramolecular assemblies, Chem. Sci., 2019, 10, 4364–4376 RSC.
- A. Proust, R. Thouvenot and P. Gouzerh, Functionalization of polyoxometalates: towards advanced applications in catalysis and materials science, Chem. Commun., 2008, 1837–1852 RSC.
- K. P. Sullivan, W. A. Neiwert, H. Zeng, A. K. Mehta, Q. Yin, D. A. Hillesheim, S. Vivek, P. Yin, D. L. Collins-Wildman, E. R. Weeks, T. Liu and C. L. Hill, Polyoxometalate-based gelating networks for entrapment and catalytic decontamination, Chem. Commun., 2017, 53, 11480–11483 RSC.
- Y. Hou, H. An, S. Chang and J. Zhang, Versatile catalysts constructed from hybrid polyoxomolybdates for simultaneously detoxifying sulfur mustard and organophosphate simulants, Catal. Sci. Technol., 2019, 9, 2445–2455 RSC.
- P. Yin, J. Wang, Z. Xiao, P. Wu, Y. Wei and T. Liu, Polyoxometalate–Organic Hybrid Molecules as Amphiphilic Emulsion Catalysts for Deep Desulfurization, Chem. – Eur. J., 2012, 18, 9174–9178 CrossRef CAS PubMed.
- M. Anjass, G. A. Lowe and C. Streb, Molecular Vanadium Oxides for Energy Conversion and Energy Storage: Current Trends and Emerging Opportunities, Angew. Chem., Int. Ed., 2021, 60, 7522–7532 CrossRef CAS PubMed.
- S. Amthor, S. Knoll, M. Heiland, L. Zedler, C. Li, D. Nauroozi, W. Tobaschus, A. K. Mengele, M. Anjass, U. S. Schubert, B. Dietzek-Ivanšić, S. Rau and C. Streb, A photosensitizer–polyoxometalate dyad that enables the decoupling of light and dark reactions for delayed on-demand solar hydrogen production, Nat. Chem., 2022, 14, 321–327 CrossRef CAS PubMed.
- A. A. Fertig, W. W. Brennessel, J. R. McKone and E. M. Matson, Concerted Multiproton–Multielectron Transfer for the Reduction of O2 to H2O with a Polyoxovanadate Cluster, J. Am. Chem. Soc., 2021, 143, 15756–15768 CrossRef CAS PubMed.
- J. W. Han and C. L. Hill, A Coordination Network That Catalyzes O2-Based Oxidations, J. Am. Chem. Soc., 2007, 129, 15094–15095 CrossRef CAS PubMed.
- X.-X. Li, Y.-X. Wang, R.-H. Wang, C.-Y. Cui, C.-B. Tian and G.-Y. Yang, Designed Assembly of Heterometallic Cluster Organic Frameworks Based on Anderson-Type Polyoxometalate Clusters, Angew. Chem., Int. Ed., 2016, 55, 6462–6466 CrossRef CAS PubMed.
- X.-X. Li, L.-J. Zhang, C.-Y. Cui, R.-H. Wang and G.-Y. Yang, Designed Construction of Cluster Organic Frameworks from Lindqvist-type Polyoxovanadate Cluster, Inorg. Chem., 2018, 57, 10323–10330 CrossRef CAS PubMed.
- H.-R. Tian, Z. Zhang, T.-Y. Dang, S.-M. Liu, Y. Lu and S.-X. Liu, Hollow Lindqvist-like-Shaped {V6} Cluster-Based Metal–Organic Framework for the Highly Efficient Detoxification of Mustard Gas Simulant, Inorg. Chem., 2021, 60, 840–845 CrossRef CAS PubMed.
- O. Linnenberg, M. Moors, A. Notario-Estévez, X. López, C. de Graaf, S. Peter, C. Baeumer, R. Waser and K. Y. Monakhov, Addressing Multiple Resistive States of Polyoxovanadates: Conductivity as a Function of Individual Molecular Redox States, J. Am. Chem. Soc., 2018, 140, 16635–16640 CrossRef CAS PubMed.
- L. E. VanGelder, A. M. Kosswattaarachchi, P. L. Forrestel, T. R. Cook and E. M. Matson, Polyoxovanadate-alkoxide clusters as multi-electron charge carriers for symmetric non-aqueous redox flow batteries, Chem. Sci., 2018, 9, 1692–1699 RSC.
- D. Li, J. Song, P. Yin, S. Simotwo, A. J. Bassler, Y. Aung, J. E. Roberts, K. I. Hardcastle, C. L. Hill and T. Liu, Inorganic-organic hybrid vesicles with counterion- and pH-controlled fluorescent properties, J. Am. Chem. Soc., 2011, 133, 14010–14016 CrossRef CAS PubMed.
- I. Fa Bamba, C. Falaise, J. Marrot, P. Atheba, G. Gbassi, D. Landy, W. Shepard, M. Haouas and E. Cadot, Host-Guest Complexation Between Cyclodextrins and Hybrid Hexavanadates: What are the Driving Forces?, Chem. – Eur. J., 2021, 27, 15516–15527 CrossRef CAS PubMed.
-
O. Linnenberg, A. Kondinski and K. Y. Monakhov, in Supramolecular Systems: Chemistry, Types and Applications, 2017, pp. 39–66 Search PubMed.
- Q. Chen and J. Zubieta, Synthesis and structural characterization of a polyoxovanadate coordination complex with a hexametalate core: [(n-C4H9)4N]2[V6O13{O2NC(CH2O)3}2], Inorg. Chem., 1990, 29, 1456–1458 CrossRef CAS.
- B. Hasenknopf, R. Delmont, P. Herson and P. Gouzerh, Anderson-Type Heteropolymolybdates Containing Tris(alkoxo) Ligands: Synthesis and Structural Characterization, Eur. J. Inorg. Chem., 2002, 2002, 1081–1087 CrossRef.
- A. V. Anyushin, A. Kondinski and T. N. Parac-Vogt, Hybrid polyoxometalates as post-functionalization platforms: From fundamentals to emerging applications, Chem. Soc. Rev., 2020, 49, 382–432 RSC.
- B. Huang, Z. Xiao, B. Wu, X. Hu, X. Hu, P. Wu and Y. Wei, Synthesis, crystal structure and spectroscopic studies of a series of hexavanadate hybrids with multiple functional groups, Inorg. Chem. Front., 2017, 4, 165–170 RSC.
- Q. Chen, D. P. Goshorn, C. P. Scholes, X. L. Tan and J. Zubieta, Coordination compounds of polyoxovanadates with a hexametalate core. Chemical and structural characterization of [VV6O13[(OCH2)3CR]2]2−, [VV6O11(OH)2[(OCH2)3CR]2], [VIV4VV2O9(OH)4[(OCH2)3CR]2]2−, and [VIV6O7(OH)6](OCH2)3CR]2]2−, J. Am. Chem. Soc., 1992, 114, 4667–4681 CrossRef CAS.
- P. Wu, J. Chen, P. Yin, Z. Xiao, J. Zhang, A. Bayaguud and Y. Wei, Solvent-induced supramolecular chirality switching of bis-(trisalkoxy)-hexavanadates, Polyhedron, 2013, 52, 1344–1348 CrossRef CAS.
- P. Yin, P. Wu, Z. Xiao, D. Li, E. Bitterlich, J. Zhang, P. Cheng, D. V. Vezenov, T. Liu and Y. Wei, A Double-Tailed Fluorescent Surfactant with a Hexavanadate Cluster
as the Head Group, Angew. Chem., Int. Ed., 2011, 50, 2521–2525 CrossRef CAS PubMed.
- P. Wu, Z. Xiao, J. Zhang, J. Hao, J. Chen, P. Yin and Y. Wei, DMAP-catalyzed esterification of pentaerythritol-derivatized POMs: A new route for the functionalization of polyoxometalates, Chem. Commun., 2011, 47, 5557–5559 RSC.
- A. Bayaguud, K. Chen and Y. Wei, Facile synthesis of an organically-derivatized hexavanadate containing the remote amino group, TBA2[V6O13{(OCH2)3CNH2}2], CrystEngComm, 2016, 18, 4042–4045 RSC.
- C. Qin and J. Zubieta, Structural investigations of the hexavanadium core {V6O19} in ‘oxidized’, mixed valence and ‘reduced’ clusters of the type [VV6−nVIVnO13−n(OH)n{(OCH2)3CR}2]2−, n=0, 3 and 6, Inorg. Chim. Acta, 1992, 198–200, 95–110 CrossRef.
- C. P. Pradeep, D.-L. Long, G. N. Newton, Y.-F. Song and L. Cronin, Supramolecular Metal Oxides: Programmed Hierarchical Assembly of a Protein-Sized 21 kDa [(C16H36N)19{H2NC(CH2O)3P2V3W15O59}4]5− Polyoxometalate Assembly, Angew. Chem., 2008, 120, 4460–4463 CrossRef.
- J. W. Han, K. I. Hardcastle and C. L. Hill, Redox-Active Coordination Polymers from Esterified Hexavanadate Units and Divalent Metal Cations, Eur. J. Inorg. Chem., 2006, 2006, 2598–2603 CrossRef.
- C. L. Hill, T. M. Anderson, J. W. Han, D. A. Hillesheim, Y. V. Geletii, N. M. Okun, R. Cao, B. Botar, D. G. Musaev and K. Morokuma, New complexes and materials for O2-based oxidations, J. Mol. Catal. A: Chem., 2006, 251, 234–238 CrossRef CAS.
- S. Vanhaecht, T. Quanten and T. N. Parac-Vogt, A Simple Nucleophilic Substitution as a Versatile Postfunctionalization Method for the Coupling of Nucleophiles to an Anderson-Type Polyoxometalate, Inorg. Chem., 2017, 56, 3095–3101 CrossRef CAS PubMed.
- S. Vanhaecht, J. Jacobs, L. Van Meervelt, T. N. Parac-Vogt, V. Meervelt and T. N. Parac-Vogt, A versatile and highly efficient post-functionalization method for grafting organic molecules onto Anderson-type polyoxometalates, Dalton Trans., 2015, 44, 19059–19062 RSC.
- S. Vanhaecht, T. Quanten and T. N. Parac-Vogt, A mild post-functionalization method for the vanadium substituted P2W15V3 Wells-Dawson polyoxometalate based on a copper catalyzed azide-alkyne cycloaddition, Dalton Trans., 2017, 46, 10215–10219 RSC.
- V. W. Day, W. G. Klemperer and D. J. Maltbie, Where are the protons in H3V10O283−?, J. Am. Chem. Soc., 1987, 109, 2991–3002 CrossRef CAS.
- V. W. Day, W. G. Klemperer and O. M. Yaghi, Synthesis and characterization of a soluble oxide inclusion complex, [CH3CN.cntnd.(V12O324−)], J. Am. Chem. Soc., 1989, 111, 5959–5961 CrossRef CAS.
- Y. Kikukawa, K. Ogihara and Y. Hayashi, Structure Transformation among Deca-, Dodeca- and Tridecavanadates and Their Properties for Thioanisole Oxidation, Inorganics, 2015, 3, 295–308 CrossRef CAS.
- D. Rehder, T. Polenova and M. Bühl, Annu. Rep. NMR Spectrosc., 2007, 62, 49–114 CrossRef CAS.
- J. M. Missina, L. B. P. Leme, K. Postal, F. S. Santana, D. L. Hughes, E. L. de Sá, R. R. Ribeiro and G. G. Nunes, Accessing decavanadate chemistry with tris(hydroxymethyl)aminomethane, and evaluation of methylene blue bleaching, Polyhedron, 2020, 180, 114414 CrossRef CAS.
- T. Yamase, Photo- and electrochromism of polyoxometalates and related materials, Chem. Rev., 1998, 98, 307–325 CrossRef CAS PubMed.
- L. Bi, E. Wang, L. Xu and R. Huang, Synthesis, properties and crystal structure of some polyoxometallates containing the tris(hydroxymethyl)aminomethane cation, Inorg. Chim. Acta, 2000, 305, 163–171 CrossRef CAS.
- K.-J. Range, R. Zintl and A. M. Heyns, The Thermal Decomposition of Ammonium Metavanadate(V) in Open and Closed Systems, Z. Für Naturforschung B, 1988, 43, 309–317 CrossRef CAS.
- A. A. Fertig and E. M. Matson, Connecting Thermodynamics and Kinetics of Proton Coupled Electron Transfer at Polyoxovanadate Surfaces Using the Marcus Cross Relation, Inorg. Chem., 2023, 62, 1958–1967 CrossRef CAS PubMed.
- A. J. Kibler and G. N. Newton, Tuning the electronic structure of organic–inorganic hybrid polyoxometalates: The crucial role of the covalent linkage, Polyhedron, 2018, 154, 1–20 CrossRef CAS.
- U. Mayer, V. Gutmann and W. Gerger, The acceptor number – A quantitative empirical parameter for the electrophilic properties of solvents, Monatshefte Für Chem. Chem. Mon., 1975, 106, 1235–1257 CrossRef CAS.
- B. Keita, D. Bouaziz and L. Nadjo, Solvent Effects on the Redox Potentials of Potassium 12-Tungstosilicate and 18-Tungstodiphosphate, J. Electrochem. Soc., 1988, 135, 87 CrossRef CAS.
- S. Ogo, S. Moroi, T. Ueda, K. Komaguchi, S. Hayakawa, Y. Ide, T. Sano and M. Sadakane, Preparation of tetrabutylammonium salt of a mono-Ru(iii)-substituted α-Keggin-type silicotungstate with a 4,4′-bipyridine ligand and its electrochemical behaviour in organic solvents, Dalton Trans., 2013, 42, 7190–7195 RSC.
- I. Fa Bamba, C. Falaise, G. K. Gbassi, P. Atheba, M. Haouas and E. Cadot, N-tert-butoxycarbonyl (BOC) protected [V6O13{(OCH2)3CNH2}2]2−: synthesis, structural characterization, and solution behavior, J. Coord. Chem., 2020, 73, 2567–2578 CrossRef CAS.
- H. Jia, Q. Li, A. Bayaguud, S. She, Y. Huang, K. Chen and Y. Wei, Tosylation of alcohols: an effective strategy for the functional group transformation of organic derivatives of polyoxometalates, Sci. Rep., 2017, 7, 12523 CrossRef PubMed.
- P. Le Maguerès, S. M. Hubig, S. V. Lindeman, P. Veya and J. K. Kochi, Novel Charge-Transfer Materials via Cocrystallization of Planar Aromatic Donors and Spherical Polyoxometalate Acceptors, J. Am. Chem. Soc., 2000, 122, 10073–10082 CrossRef.
-
E. V. Anslyn and D. A. Dougherty, Modern Physical Organic Chemistry, University Science Books, 2006 Search PubMed.
- O. Linnenberg, A. Kondinski, C. Stöcker and K. Y. Monakhov, The Cu(I)-catalysed Huisgen 1,3-dipolar cycloaddition route to (bio-)organic functionalisation of polyoxovanadates, Dalton Trans., 2017, 46, 15636–15640 RSC.
- N. Gao, H. Sun, K. Dong, J. Ren, T. Duan, C. Xu and X. Qu, Transition-metal-substituted polyoxometalate derivatives as functional anti-amyloid agents for Alzheimer's disease, Nat. Commun., 2014, 5, 3422 CrossRef PubMed.
- V. A. Zamolo, G. Modugno, E. Lubian, A. Cazzolaro, F. Mancin, L. Giotta, D. Mastrogiacomo, L. Valli, A. Saccani, S. Krol, M. Bonchio and M. Carraro, Selective Targeting of Proteins by Hybrid Polyoxometalates: Interaction Between a Bis-Biotinylated Hybrid Conjugate and Avidin, Front. Chem., 2018, 6, 278 CrossRef PubMed.
- Y. Gu, Q. Li, Y. Huang, Y. Zhu, Y. Wei and L. Ruhlmann, Polyoxovanadate-iodobodipy supramolecular assemblies: new agents for high efficiency cancer photochemotherapy, Chem. Commun., 2020, 56, 2869–2872 RSC.
- S. Park, E. Kim, W. Y. Kim, C. Kang and J. S. Kim, Biotin-guided anticancer drug delivery with acidity-triggered drug release, Chem. Commun., 2015, 51, 9343–9345 RSC.
- C. Falaise, M. A. Moussawi, S. Floquet, P. A. Abramov, M. N. Sokolov, M. Haouas and E. Cadot, Probing Dynamic Library of Metal-Oxo Building Blocks with γ-Cyclodextrin, J. Am. Chem. Soc., 2018, 140, 11198–11201 CrossRef CAS PubMed.
- F. Schibilla, J. Voskuhl, N. A. Fokina, J. E. P. Dahl, P. R. Schreiner and B. J. Ravoo, Host–Guest Complexes of Cyclodextrins and Nanodiamonds as a Strong Non-Covalent Binding Motif for Self-Assembled Nanomaterials, Chem. – Eur. J., 2017, 23, 16059–16065 CrossRef CAS PubMed.
- L. Ni, H. Li, H. Xu, C. Shen, R. Liu, J. Xie, F. Zhang, C. Chen, H. Zhao, T. Zuo and G. Diao, Self-Assembled Supramolecular Polyoxometalate Hybrid Architecture as a Multifunctional Oxidation Catalyst, ACS Appl. Mater. Interfaces, 2019, 11, 38708–38718 CrossRef CAS PubMed.
- A. Štimac, M. Šekutor, K. Mlinarić-Majerski, L. Frkanec and R. Frkanec, Adamantane in Drug Delivery Systems and Surface Recognition, Mol. Basel Switz., 2017, 22, 297 Search PubMed.
- C. F. Chew, A. Guy and P. C. Biggin, Distribution and Dynamics of Adamantanes in a Lipid Bilayer, Biophys. J., 2008, 95, 5627–5636 CrossRef CAS PubMed.
- N. Bošnjaković-Pavlović, X. Xu, D. Krstić, J.-M. Gillet, Y. Wei, P. Wu, M. Čolović and A. Spasojević-de Biré, Experimental and theoretical insights of functionalized hexavanadates on Na+/K+-ATPase activity; molecular interaction field, ab initio calculations and in vitro assays, J. Inorg. Biochem., 2019, 198, 110720 CrossRef PubMed.
- P. Wu, Y. Wang, W. Chen, X. Hu, B. Huang and Z. Xiao, Structural and Magnetical Studies of Mixed-Valence Hexavanadate Hybrids: How Organic Ligands Affect the Magnetism of Polyoxometalates?, Inorg. Chem., 2021, 60, 4347–4351 CrossRef CAS PubMed.
- D. E. Salazar Marcano, M. A. Moussawi, A. V. Anyushin, S. Lentink, L. Van Meervelt, I. Ivanović-Burmazović and T. N. Parac-Vogt, Versatile post-functionalisation strategy for the formation of modular organic–inorganic polyoxometalate hybrids, Chem. Sci., 2022, 13, 2891–2899 RSC.
Footnotes |
† Electronic supplementary information (ESI) available. CCDC 2217991 and 2217992. For ESI and crystallographic data in CIF or other electronic format see DOI: https://doi.org/10.1039/d3sc00038a |
‡ Authors contributed equally to this work. |
|
This journal is © The Royal Society of Chemistry 2023 |
Click here to see how this site uses Cookies. View our privacy policy here.