DOI:
10.1039/D3SC00198A
(Edge Article)
Chem. Sci., 2023,
14, 3462-3469
Direct formation of amide/peptide bonds from carboxylic acids: no traditional coupling reagents, 1-pot, and green†
Received
12th January 2023
, Accepted 27th February 2023
First published on 28th February 2023
Abstract
Technology for generating especially important amide and peptide bonds from carboxylic acids and amines that avoids traditional coupling reagents is described. The 1-pot processes developed rely on thioester formation, neat, using a simple dithiocarbamate, and are safe and green, and rely on Nature-inspired thioesters that are then converted to the targeted functionality.
Introduction
According to a paper back in 2016 by Brown and Boström, amide/peptide bonds are the number one type of construction practiced in medicinal chemistry.1 A virtual toolbox full of coupling reagents has been accumulated to meet the many challenges posed by reactions between carboxylic acids and amines which formally release an equivalent of water. Some of these reagents are quite aged while others are of relatively recent vintage, including those that are “green” in nature (e.g., see Fig. 1, and the very recent review in ref. 2). Although most of these reagents in use are now readily available, and in many cases considered even inexpensive, there can be no argument about the by-products formed, which may present separation issues. Most, with perhaps the exception of COMU leading to polypeptides formed in an aqueous medium,3 have also been utilized in strictly waste-generating organic solvents. Particularly noteworthy is the well-known early warning on the explosiveness of HOBt, and the more recent report by Nowick and co-workers4 highlighting the non-trivial safety issues (especially anaphylaxis) that surround use of common uronium couplings agents HATU, HBTU, and HCTU. Thus, while applications of these dehydrating agents continue to appear unabated due to the importance of the amide/peptide unit, there is more than ample justification for devising alternatives that avoid these issues in their entirety;5 indeed, a truly green chemical solution applicable to highly functionalized molecules and that can be used in a sequential fashion in the same reaction vessel seems long overdue.6
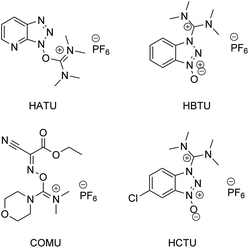 |
| Fig. 1 Commonly used amide/peptide coupling reagents. | |
In this report, therefore, is presented a 1-pot solution that appears to be general, safe, and very responsible in terms of its impact on the environment, and which allows for applications to several challenging yet representative targets within the pharmaceutical industry. At the heart of this approach lies recognition of the simple question: How does Nature make these bonds? And while one can quickly gain an appreciation for the complexities of such constructions, the overarching conclusion is that thioesters are usually key intermediates along the biosynthetic pathway to the desired functionality. The choice of the “right” thioester loomed large in developing this technology, since the required bonds must be formed using water as the reaction medium, which is Nature's chosen “solvent.” Prior art, albeit not related to amide/peptide bond formation involving thioesters certainly exists,7 and here, the work of Fukuyama stands out,8 although such alkyl thioester intermediates, made in, and isolated from, organic solvents are inappropriate for the goals at hand.
They typically involve initial acid chloride formation, and the derived thioesters are considered mainly as educts for reductions to aldehydes, as well as intermediates en route to ketones.9 It was also important to acknowledge and deal with the common criticism associated with much of sulfur chemistry; that is, the potential odor. Moreover, the reagent to make the thioester of choice had to be readily available and inexpensive, while the efficiency of its use in thioester formation had to be such that the overall conversion to the amide/peptide would be high-yielding.
Ultimately, the ideal thioester was that containing the 2-thiopyridyl residue, where DiPyridylDiThioCarbonate, or DPDTC (1) is readily derived in multi-gram quantities from treatment of commercially available 2-mercaptopyridine with triphosgene (Scheme 1), following an old recipe used to arrive at several common reagents (e.g., CDI) at scale.9 This odorless dithiocarbonate (DPDTC) had been made decades ago,10 and more recently, related albeit limited applications have also been reported by Lee.11 Preparation of thioester intermediates is typically performed in organic solvents (e.g., CH3CN), and usually isolated prior to conversion to various functionalities. The corresponding 1-pot process is also known in a single case.11 Hence, reagents such as DPDTC are rarely used, and could rightly be considered today, as far from sustainable technologies.
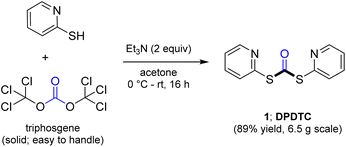 |
| Scheme 1 Formation of DPDTC for use in thioester formation. | |
Results and discussion
Formation of a 2-pyridylthioester using DPDTC could be accomplished in an aqueous micellar medium, as shown in Scheme 2. While such intermediates are isolable and have considerable shelf life, it was very straightforward to treat these in situ-formed thioesters directly with an amine to form the corresponding amide. And while this approach offers the desired simplicity and fulfils many of the criteria for “greenness”, the potential for competitive hydrolysis of the thioester back to its precursor acid imparted an element of unpredictability given the aqueous medium involved. Hence, as shown in Scheme 3, isolated yields of targeted amide could vary substantially, even when catalytic DMAP was present to enhance the rate of initial thioester formation.
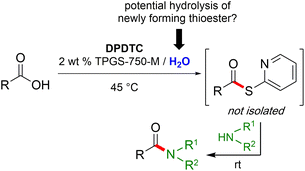 |
| Scheme 2 Thioester formation in water followed by conversion to amides/peptides. | |
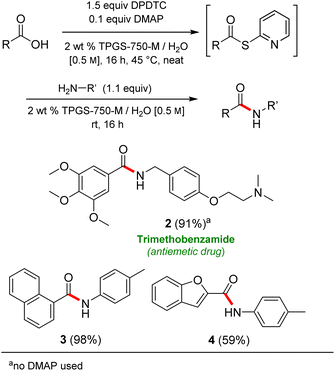 |
| Scheme 3 Representative examples of amide bond formation in 1-pot, all in water. | |
In full appreciation of the guidance offered years ago by Sheldon and co-workers, in whose monograph Green Chemistry and Catalysis it states: “The best solvent is no solvent…”,12 we found that by adding DPDTC to the acid (solid or otherwise) followed by gentle heating (i.e., neat) to ca. 60 °C, DPDTC melts leading to the desired thioester, which is formed cleanly (see ESI, Table S1†). At this stage, and without thioester isolation, three different approaches were developed for subsequent conversion to the corresponding amides/peptides (Scheme 4): (1) direct addition of an amine (neat; see ESI, Table S4†); (2) use of concentrated solutions of the amine in EtOAc (2 M; see ESI, Table S4†); and (3) dilution with aqueous 2 wt% TPGS-750-M13 (see ESI, Table S9†); followed by introduction of the amine in the presence of small amounts of co-solvent (e.g., EtOAc), which can aid in maintaining an emulsified reaction medium. Surfactant MC-1 is also viable for more polar cases.14 Examples using each of these 1-pot procedures are shown in Scheme 5A (neat conditions), 5B (in 2 M EtOAc), and 5C (in aqueous TPGS-750-M). These were all chosen to document the overall efficiencies to be expected regardless of the approach selected. It should also be appreciated that both amides and peptides (e.g., 16, along with the application of this technology to nirmatrelvir)15 are amenable, and are formed without loss of stereointegrity. Tolerance to a very wide array of functionality within these cases is also noteworthy. Method B, that relies on concentrated solutions of EtOAc, was selected since recovery of this single greener organic solvent is readily achieved. Likewise, when using an aqueous micellar medium (method C), its recycling following amide formation and after an extractive work up with recoverable ethyl acetate is routine. Moreover, the process associated with method C could be smoothly scaled to the gram level to give 5 in 94% isolated yield (see ESI, section 8†). Several additional applications of this 1-pot sequence have been made with a focus on targets in the pharmaceutical area. Thus, as illustrated in Scheme 6, compounds of known bioactivity, such as sonidegib (27), imatinib (28), olaparib (30), antimalarial tafenoquine analog 31, and fluxapyroxad (32) are all prepared in very good isolated yields. Highly functionalized drug analog 33, related to polypeptide nirmatrelvir, and as with nirmatrelvir itself,15 no indication as to the loss of stereointegrity (i.e., epimerization) was observed. Also, included within this same study are two amides (38) and (39) derived from heavily functionalized educts within the Merck Informer Library,16 suggestive of opportunities for late-stage functionalization. Taken together, these examples are indicative not only of the quality of the bond constructions and functional group tolerance, but importantly, the environmentally responsible conditions under which such couplings are now possible. In comparison to literature methods, this 1-pot conversion not only allows for an isolable and stable intermediate, but also affords equal or better yields, as demonstrated in Table 1 with representative compounds 6, 21, and 42.
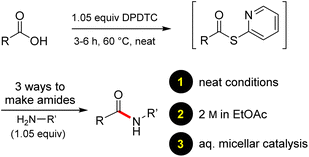 |
| Scheme 4 Approaches to amide formation from the intermediate thioester. | |
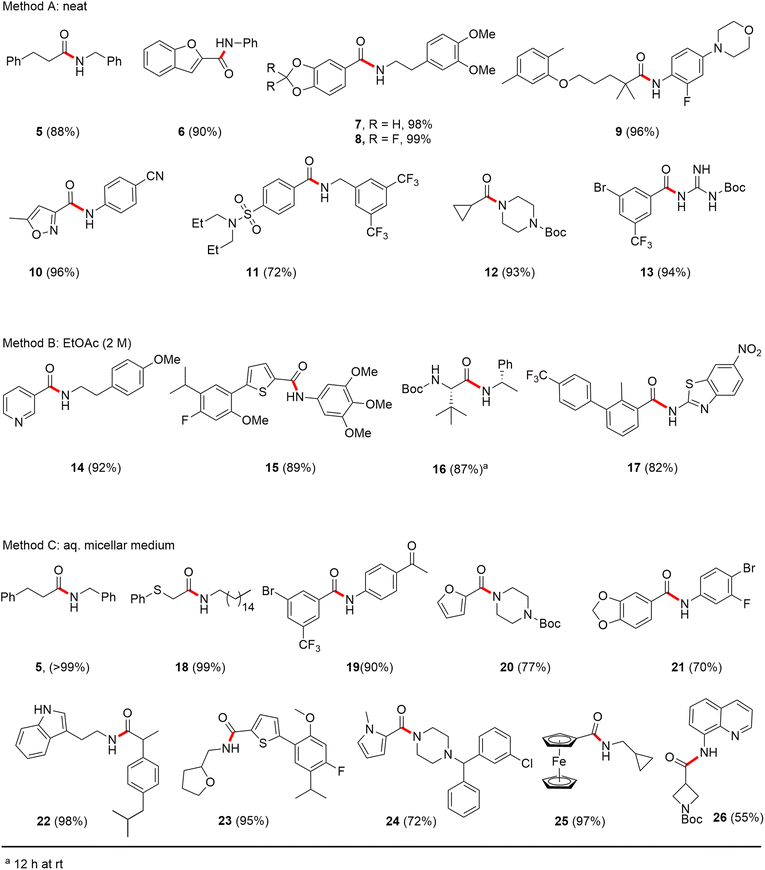 |
| Scheme 5 (A) Examples of amide bond formation using neat conditions (method A). (B) Examples of amide bond formation using highly concentrated mixtures in EtOAc (2 M; method B). (C) Examples of amide bond formation using thioesters to which is added an aqueous micellar medium (method C). | |
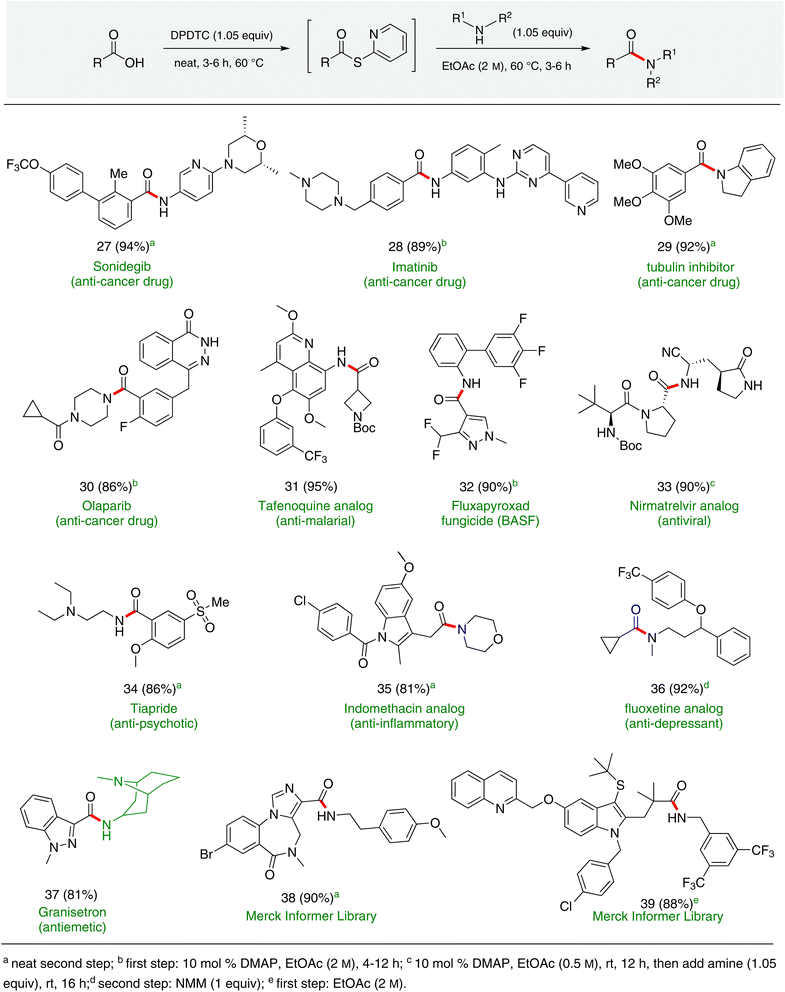 |
| Scheme 6 Amide/peptide bond formations featuring highly functionalized reaction partners. | |
Table 1 Comparison cases with existing literature examples
Substrate |
This work |
Literature |
|
90% |
87% (ref. 17a) |
77% (ref. 17b) |
|
77% |
71% (ref. 18) |
|
80% |
40% (ref. 19) |
Opportunities also exist to form primary amides using this same approach. Examples of four such isolable products are shown in Scheme 7. In these cases, after formation of the intermediate thioesters, addition of aqueous ammonium hydroxide (2 equiv.) afforded the targeted amides in good yields.
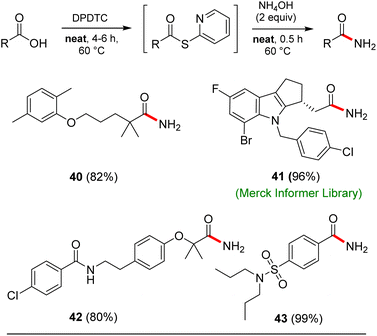 |
| Scheme 7 Examples of neat conditions applied to formation of primary amides. | |
This particular conversion takes on special significance in that the newly formed primary amides are immediate precursors to the corresponding nitrile derivatives via dehydration. For example, starting with probenecid (Scheme 8), arylnitrile-containing 4-cyano-N,N-dipropylbenzenesulfonamide 44 was ultimately formed via: (a) thioester generation; (b) treatment with aqueous ammonia, and (c) Pd-catalyzed dehydration using methoxyacetonitrile as the sacrificial, water-absorbing nitrile.20 The overall isolated yield for this 1-pot process was 90%. Likewise, the derived alkylnitrile of bezafibrate 45 was formed via dehydration of the primary amide 42 with TFAA21 in 1-pot (Scheme 9).
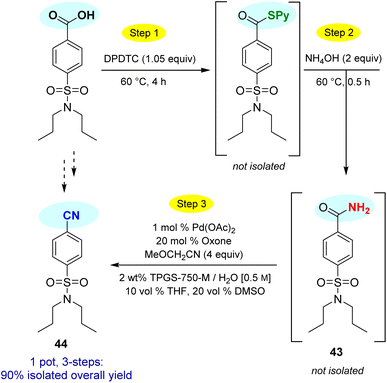 |
| Scheme 8 1-Pot conversion of a carboxylic acid to its derived nitrile via Pd-catalyzed dehydration. | |
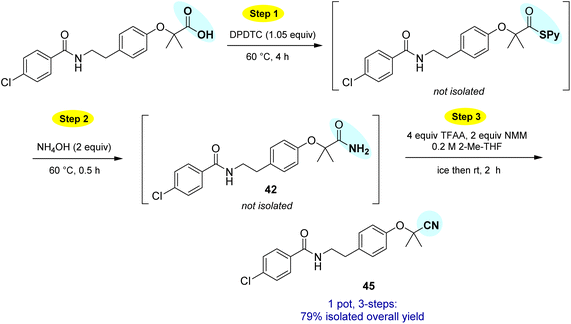 |
| Scheme 9 1-Pot conversion of a carboxylic acid to its derived nitrile. | |
The use of neat conditions to form intermediate thioesters followed by addition of ethyl acetate (2 M), allows for more than initial amide formation, these additional bonds also being made in the same 1-pot fashion (Scheme 10). Thus, from the extensive toolbox of reactions that can now be run under green conditions,22 after generating the initial amide bond between 4-aminoacetophenone and 3-bromo-5-(trifluoromethyl)-benzoic acid to afforded N-(4-acetylphenyl)-3-bromo-5-(trifluoro-methyl)benzamide 19. Addition of sodium borohydride affords secondary benzylic alcohol 46. Without isolation, introduction of a Merck Informer Library-derived thioester 47 ultimately arrives at highly functionalized ester 48 (45%), a 4-step, 1-pot sequence. The ester bond made in this fashion via the 2-pyridyl thioester is representative of the additional technology envisioned (e.g., to make esters) and which is currently under further development. The potential to benefit from both time23 and pot economies,24 not to mention the savings to be gained in terms of waste creation, should be quite apparent.
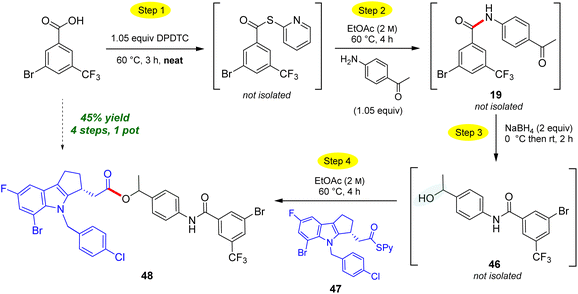 |
| Scheme 10 A representative 4-step, 1-pot sequence. | |
In forming intermediate thioesters, DPDTC leads to loss of only CO2 and an equivalent of (odorless) 2-pyridinethiol. The mercaptan can be recovered via manipulation of the pH of the aqueous medium. Hence, both “by-products” are easily removed relative to handling of most other traditional coupling reagents. Amide formation using this 1-pot sequence also eliminates the need for base in the coupling step, which could be advantageous when epimerizable stereocenters are present.
The calculated Process Mass Intensity (PMI)25 associated with formation of 43 was determined on the basis of a 0.25 mmol scale reaction, while the data provided in Table 2 are an average of multigram reactions run by GSK.26 With increasing scale it is likely that the PMI derived from the workup for this methodology would decrease. For reactions where the product readily precipitates out, e.g., for some primary amides, the PMI decreases by ca. 50% since the product is simply filtered and hence, there is no need for a workup (see last entry in Table 2).
Table 2 Comparisons of PMI values en route to 43 with literature values26
Reagent |
PMI from reaction |
PMI from work-up |
Overall PMI |
Acid chloride |
24 |
11 |
35 |
Mixed anhydride |
24 |
19 |
43 |
T3P |
12 |
31 |
43 |
CDI |
16 |
36 |
52 |
HATU |
11 |
23 |
34 |
EDC |
15 |
31 |
46 |
Oxalyl chloride |
12 |
48 |
60 |
DPDTC (neat; compound 43) |
0.94
|
14.4
|
15.3
|
Finally, it is worth noting that these same thioesters are also amenable to conversion to several additional functional groups other than amides/peptides, including, aldehydes, esters, thioesters, and ketones, in addition to esters (see formation of 48 in Scheme 10, and Fig. 2). For example, net conversion of a carboxylic acid to the corresponding aldehyde without resorting to DIBAL reductions of initially prepared esters or Weinreb amides, or alternative reductions that require initial acid halide formation, are processes now in hand. Likewise, reductions of acids directly to alcohols, commonly described within textbooks as under the domain of LAH, can be done safely in 1-pot, in water. Details for these new processes will be reported shortly.
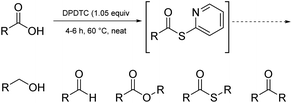 |
| Fig. 2 Functional groups realizable via the same in situ-formed thioesters. | |
Conclusions
A safe, effective, and general 1-pot procedure to arrive at highly desired amides has been developed that follows Nature's lead: using a readily formed thioester intermediate, followed by its conversion in 1-pot to the desired product. Applications to several important and highly functionalized amide/peptide-containing targets have been documented herein. The key aspects to this contribution can be summarized as follows:
• Traditional peptide coupling reagents are no longer needed.
• Relies on initial thioester formation involving an easily made, odorless reagent DPDTC (dipyridyl dithiocarbonate).
• Three approaches have been developed, each leading to the same end, are less waste-generating and hence, are far “greener” than are existing technologies.
• Where present, stereocenters are maintained.
• Several sequences are illustrated highlighting options for multi-step processes in a single pot, illustrative of both time and pot economies.
• A novel process that converts, in 1-pot, an acid to a nitrile via the in situ-formed primary amides has been disclosed, applicable to both aryl- and alkyl-substituted carboxylic acids.
Data availability
The synthetic procedures, characterization, and spectral data supporting this article have been uploaded as part of the ESI.†
Author contributions
All authors have given approval to the final version of the manuscript. K. M. F. and R. D. K. contributed equally. R. M. T. contributed to the 1-pot, four step sequence. D. M. R. contributed to initial optimization. P. D. contributed to optimization of the formation of the thioester. M. S. contributed to purification of substrates, and 1-pot sequences. B. H. L. oversaw work and aided in drafting the manuscript.
Conflicts of interest
There are no conflicts to declare.
Acknowledgements
Financial support from the Bill and Melinda Gates Foundation, PHT International, and Anthem Biosciences is warmly acknowledged with thanks.
Notes and references
- D. G. Brown and J. Boström, J. Med. Chem., 2016, 59, 4443–4458 CrossRef CAS PubMed.
-
(a) J. R. Dunetz, J. Magano and G. A. Weisenburger, Org. Process Res. Dev., 2016, 20, 140–177 CrossRef CAS;
(b) A very recent review has appeared covering most of the commonly used reagents, past and present, for making amide bonds, including those processes that are considered “green.” However, there is no mention in this extensive contribution of any thioester-based approach(es), let alone one that relies on DPDTC. See: J. Magano, Org. Process Res. Dev., 2022, 26, 1562–1689 CrossRef CAS;
(c) For other leading references to procedures considered within the green chemistry space, see: M. Shi, N. Ye, W. Chen, H. Wang, C. Cheung, M. Parmentier, F. Gallou and B. Wu, Org. Process Res. Dev., 2020, 24, 1543–1548 CrossRef CAS;
(d) D. Petkova, N. Borlinghaus, S. Sharma, J. Kaschel, T. Lindner, J. Klee, A. Jolit, V. Haller, S. Heitz, K. Britze, J. Dietrich, W. Braje and S. Handa, ACS Sustainable Chem. Eng., 2020, 8, 12612–12617 CrossRef CAS;
(e) S. Sharma, G. Kaur and S. Handa, Org. Process Res. Dev., 2021, 25(8), 1960–1965 CrossRef CAS;
(f) S. Sharma, T. N. Ansari and S. Handa, ACS Sustainable Chem. Eng., 2021, 9, 12719–12728 CrossRef CAS;
(g) S. Hazra, F. Gallou and S. Handa, ACS Sustainable Chem. Eng., 2022, 10, 5299–5306 CrossRef CAS;
(h) C. Manske, M. Schmiedtchen, S. Gellhaar, M. Kiesel and J. Becker, ACS Sustainable Chem. Eng., 2022, 10, 5307–5314 CrossRef CAS;
(i) S. Sharma, N. W. Buchbinder, W. M. Braje and S. Handa, Org. Lett., 2020, 22(15), 5737–5740 CrossRef CAS PubMed;
(j) M. Parmentier, M. K. Wagner, K. Magra and F. Gallou, Org. Process Res. Dev., 2016, 20, 1104–1107 CrossRef CAS;
(k) K. Ishihara, S. Ohara and H. Yamamoto, J. Org. Chem., 1996, 61, 4196–4197 CrossRef CAS PubMed;
(l) R. M. Al-Zoubi, O. Marion and D. G. Hall, Angew. Chem., Int. Ed., 2008, 47, 2876–2879 CrossRef CAS PubMed;
(m) S. Fatemi, N. Gernigon and D. G. Hall, Green Chem., 2015, 17, 4016–4028 RSC;
(n) M. T. Sabatini, L. T. Boulton and T. D. Sheppard, Sci. Adv., 2017, 3, e1701028 CrossRef;
(o) Z. Liu, H. Noda, M. Shibasaki and N. Kumagai, Org. Lett., 2018, 20, 612–615 CrossRef CAS;
(p) P. H. Huy and C. Mbouhom, Chem. Sci., 2019, 10, 7399 RSC;
(q) K. Michigami, T. Sakaguchi and Y. Takemoto, ACS Catal., 2020, 10, 683–688 CrossRef CAS.
-
(a) C. M. Gabriel, M. Keener, F. Gallou and B. H. Lipshutz, Org. Lett., 2015, 17, 3968–3971 CrossRef CAS;
(b) F. Gallou, P. Guo, M. Parmentier and J. Zhou, Org. Process Res. Dev., 2016, 20(7), 1388–1391 CrossRef CAS;
(c) M. Cortes-Clerget, S. E. Spink, G. P. Gallagher, L. Chaisemartin, E. Filaire, J.-Y. Berthon and B. H. Lipshutz, Green Chem., 2019, 21, 2610–2614 RSC;
(d) M. Cortes-Clerget, N. R. Lee and B. H. Lipshutz, Nat. Protoc., 2019, 14, 1108–1129 CrossRef CAS.
-
(a) K. J. McKnelly, W. Sokol and J. S. Nowick, J. Org. Chem., 2020, 85, 1764–1768 CrossRef CAS PubMed;
(b)
https://cen.acs.org/safety/lab-safety/Peptide-coupling-agents-cause-severe/98/web/2020/01, accessed on January 06, 2023;
(c) J. C. Graham, A. Trejo-Martin, M. L. Chilton, J. Kostal, J. Bercu, G. L. Beutner, U. S. Bruen, D. G. Dolan, S. Gomez, J. Hillegass, J. Nicolette and M. Schmitz, Chem. Res. Toxicol., 2022, 35, 1011–1022 Search PubMed.
-
(a) L. S. Liebeskind, P. Gangireddy and M. G. Lindale, J. Am. Chem. Soc., 2016, 138, 6715–6718 CrossRef CAS PubMed;
(b) G. L. Beutner, I. S. Young, M. L. Davies, M. R. Hickey, H. Park, J. M. Stevens and Q. Ye, Org. Lett., 2018, 20, 4218–4222 CrossRef CAS;
(c) S. M. Akondi, P. Gangireddy, T. C. Pickel and L. S. Liebeskind, Org. Lett., 2018, 20, 538–541 CrossRef CAS PubMed;
(d) W. D. G. Brittain and S. L. Cobb, Org. Lett., 2021, 23, 5793–5798 CrossRef CAS;
(e) T. G. Bolduc, C. Lee, W. P. Chappell and G. M. Sammis, J. Org. Chem., 2022, 87, 7308–7318 CrossRef CAS PubMed;
(f) W. Muramatsu and H. Yamamoto, Org. Lett., 2022, 24, 7194–7199 CrossRef CAS;
(g) E. Tosi, J.-M. Campagne and R. Marcia de Figueiredo, J. Org. Chem., 2022, 87, 12148–12163 CrossRef CAS;
(h) B. D. Mkhonazi, E. M. Mabila and P. T. Moshapo, SynOpen, 2022, 6, 211–218 CrossRef CAS.
-
(a) M. R. Petchey and G. Grogan, Adv. Synth. Catal., 2019, 361, 3895–3914 CrossRef CAS;
(b) M. Petchey, A. Cuetos, B. Rowlinson, S. Dannevald, A. Frese, P. W. Sutton, S. Lovelock, R. C. Lloyd and I. J. S. Fairlamb, Angew. Chem., Int. Ed., 2018, 57, 11584–11588 CrossRef CAS;
(c) A. J. L. Wood, N. J. Weise, J. D. Frampton, M. S. Dunstan, M. A. Hollas, S. R. Derrington, R. C. Lloyd, D. Quaglia, F. Parmeggiani, D. Leys, N. J. Turner and S. L. Flitsch, Angew. Chem., Int. Ed., 2017, 56, 14498–14501 CrossRef CAS.
-
(a) E. J. Corey and D. A. Clark, Tetrahedron Lett., 1979, 20, 2875–2878 CrossRef;
(b) N. Scardovi, P. P. Garner and J. D. Protasiewicz, Org. Lett., 2003, 5, 1633–1635 CrossRef CAS PubMed;
(c) S. Hanessian, V. Mascitti and O. Rogel, J. Org. Chem., 2002, 67, 3346–3354 CrossRef CAS PubMed.
-
(a) H. Tokuyama, S. Yokoshima, T. Yamashita and T. Fukuyama, Tetrahedron Lett., 1998, 39, 3189–3192 CrossRef CAS;
(b) H. Tokuyama, S. Yokoshima, T. Yamashita, S.-C. Lin, L. Li and T. Fukuyama, J. Braz. Chem. Soc., 1998, 9, 381–387 CrossRef CAS;
(c) T. Fukuyama and H. Tokuyama, Aldrichimica Acta, 2004, 37, 87–96 CAS.
-
(a) H. A. Staab, Liebigs Ann. Chem., 1957, 609, 75–83 CrossRef CAS;
(b) H. A. Staab and K. Wendel, Org. Synth., 1968, 48, 44 CrossRef CAS.
- J. I. Lee and H. Park, Bull. Korean Chem. Soc., 2001, 22, 421–423 CAS.
- J. I. Lee, Bull. Korean Chem. Soc., 2020, 41, 735–747 CrossRef.
-
(a) R. A. Sheldon, Green Chem., 2005, 7, 267–278 RSC;
(b)
R. A. Sheldon, I. W. C. E. Arends and U. Hanefeld, Green Chemistry and Catalysis, Wiley-VCH, Weinheim, 2007 CrossRef;
(c) A. Sarkar, S. Santra, S. K. Kundu, A. Hajra, G. V. Zyryanov, O. N. Chupakhin, V. N. Charushinbd and A. Majee, Green Chem., 2016, 18, 4475–4525 RSC;
(d) K. Tanaka and F. Toda, Chem. Rev., 2000, 100, 1025–1074 CrossRef CAS PubMed;
(e) A. L. Garay, A. Pichon and S. L. James, Chem. Soc. Rev., 2007, 36, 846 RSC;
(f) M. A. P. Martins, C. P. Frizzo, D. N. Moreira, L. Buriol and P. Machado, Chem. Rev., 2009, 109, 4140–4182 CrossRef CAS;
(g) R. S. Varma, Green Chem., 1999, 1, 43–55 RSC;
(h) P. J. Walsh, H. Li and C. Anaya de Parrodi, Chem. Rev., 2007, 107, 2503–2545 CrossRef CAS PubMed;
(i) S. Zangade and P. Patil, Curr. Org. Chem., 2019, 23, 2295–2318 CrossRef CAS.
- S. Ghorai, A. R. Abela, R. Moser, T. Nishikata, C. Duplais, A. Krasovskiy, R. D. Gaston, R. C. Gadwood and B. H. Lipshutz, J. Org. Chem., 2011, 76, 4379–4391 CrossRef.
- M. Cortes-Clerget, S. E. Spink, G. P. Gallagher, L. Chaisemartin, E. Filaire, J. Berthonb and B. H. Lipshutz, Green Chem., 2019, 21, 2610–2614 RSC.
- J. R. A. Kincaid, J. C. Caravez, K. S. Iyer, R. D. Kavthe, N. Fleck, D. H. Aue and B. H. Lipshutz, Commun. Chem., 2022, 5, 1–7 CrossRef PubMed.
- P. S. Kutchukian, J. F. Dropinski, K. D. Dykstra, B. Li, D. A. DiRocco, E. C. Streckfuss, L.-C. Campeau, T. Cernak, P. Vachal, I. W. Davies, S. W. Krska and S. D. Dreher, Chem. Sci., 2016, 7, 2604–2613 RSC.
-
(a) M. Choi, H. Jo, H.-J. Park, A. S. Kumar, J. Lee, J. Yun, Y. Kim, S. Han, J.-K. Jung, J. Cho, K. Lee, J.-H. Kwak and H. Lee, Bioorg. Med. Chem. Lett., 2015, 25, 2545–2549 CrossRef CAS PubMed;
(b) M. Oschmann, L. J. Holm, M. Pourghasemi-Lati and O. Verho, Molecules, 2020, 25, 361 CrossRef CAS.
- O. W. M. Baldwin, L. H. Conrad-Marut, G. L. Beutner and D. A. Vosburg, J. Chem. Educ., 2022, 99, 3747–3751 CrossRef PubMed.
- X. Chen, J. Kang, Y. Ma and Y. Miao, Green Chem., 2021, 23, 3595–3599 RSC.
- A. Wood, J. Kincaid and B. H. Lipshutz, Green Chem., 2022, 24, 2853–2858 RSC.
- V. V. Sureshbabu, R. Venkataramanarao, S. A. Naik and G. Chennakrishnareddy, Tetrahedron Lett., 2007, 48, 7038–7041 CrossRef CAS.
- See, for example, M. Cortes-Clerget, J. Yu, J. R. A. Kincaid, P. Walde, F. Gallou and B. H. Lipshutz, Chem. Sci., 2021, 12, 4237–4266 RSC , and references therein..
- Y. Hayashi, J. Org. Chem., 2021, 86, 1–23 CrossRef CAS PubMed.
- Y. Hayashi, Chem. Sci., 2016, 7, 866–880 RSC.
-
(a) E. R. Monteith, P. Mampuys, L. Summerton, J. H. Clark, B. U. W. Maes and C. R. McElroy, Green Chem., 2020, 22, 123–135 RSC;
(b) R. A. Sheldon, ACS Sustainable Chem. Eng., 2018, 6, 32–48 CrossRef CAS;
(c) C. Jimenez-Gonzalez, C. S. Ponder, Q. B. Broxterman and J. B. Manley, Org. Process Res. Dev., 2011, 15, 912–917 CrossRef CAS;
(d) D. Cespi, E. S. Beach, T. E. Swarr, F. Passarini, I. Vassura, P. J. Dunn and P. T. Anastas, Green Chem., 2015, 17, 3390–3400 RSC.
- J. P. Adams, C. M. Alder, I. Andrews, A. M. Bullion, M. Campbell-Crawford, M. G. Darcy, J. D. Hayler, R. K. Henderson, C. A. Oare, I. Pendrak, A. M. Redman, L. E. Shuster, H. F. Sneddon and M. D. Walker, Green Chem., 2013, 15, 1542–1549 RSC.
|
This journal is © The Royal Society of Chemistry 2023 |
Click here to see how this site uses Cookies. View our privacy policy here.