DOI:
10.1039/D3SC01677C
(Edge Article)
Chem. Sci., 2023,
14, 9704-9723
Understanding C–H activation in light alkanes over Cu-MOR zeolites by coupling advanced spectroscopy and temperature-programmed reduction experiments†
Received
31st March 2023
, Accepted 19th August 2023
First published on 22nd August 2023
Abstract
The direct activation of methane to methanol (MTM) proceeds through a chemical-looping process over Cu-oxo sites in zeolites. Herein, we extend the overall understanding of oxidation reactions over metal-oxo sites and C–H activation reactions by pinpointing the evolution of Cu species during reduction. To do so, a set of temperature-programmed reduction experiments were performed with CH4, C2H6, and CO. With a temperature ramp, the Cu reduction could be accelerated to detect changes in Cu speciation that are normally not detected due to the slow CH4 adsorption/interaction during MTM (∼200 °C). To follow the Cu-speciation with the three reductants, X-ray absorption spectroscopy (XAS), UV-vis and FT-IR spectroscopy were applied. Multivariate curve resolution alternating least-square (MCR-ALS) analysis was used to resolve the time-dependent concentration profiles of pure Cu components in the X-ray absorption near edge structure (XANES) spectra. Within the large datasets, as many as six different CuII and CuI components were found. Close correlations were found between the XANES-derived CuII to CuI reduction, CH4 consumption, and CO2 production. A reducibility–activity relationship was also observed for the Cu-MOR zeolites. Extended X-ray absorption fine structure (EXAFS) spectra for the pure Cu components were furthermore obtained with MCR-ALS analysis. With wavelet transform (WT) analysis of the EXAFS spectra, we were able to resolve the atomic speciation at different radial distances from Cu (up to about 4 Å). These results indicate that all the CuII components consist of multimeric CuII-oxo sites, albeit with different Cu–Cu distances.
1 Introduction
Methane, being the principal component of natural gas, is a vastly underutilized raw material for the chemical industry.1 Due to the strong C–H bond in light alkanes, one of the biggest challenges to overcome is the over-oxidation of the desired products. Therefore, extensive research efforts have been devoted to reaction design and material development to find direct reaction routes that can overcome the issue of over-oxidation.2,3 These approaches involve the direct activation of methane-to-methanol. A high selectivity toward methanol has been obtained in a direct reaction route, albeit at the expense of a high yield. One of the more promising methods to obtain a high selectivity has been through a cyclic, stepwise reaction process where both products and reactants are kept separate with the aid of Cu-loaded zeolites that form CuxOy-moieties when exposed to oxygen at high temperatures (∼500 °C).4 In the second step, at lower temperatures (∼200 °C), the CuxOy-moieties are exposed to CH4 leading to the reduction of some of the Cu-oxo sites and the formation of methoxy intermediates. In the final step, steam is introduced to extract methanol as the product. To increase the output of the reaction, while maintaining the selectivity, fine-tuning of reaction protocols and optimization of materials are essential.3,5 Consequently, the effect of Cu speciation, material properties, and reaction mechanism need to be unfolded and understood. Learning more about these properties would also potentially open the doors to other oxidation reactions and C–H activation routes.
Over the years, many zeolite systems have been studied, where the more active ones are MOR,6–11, CHA,12,13 FER,14 FAU,15 and MAZ.16 The mentioned zeolites feature a combination of large and small ring systems, rendering it difficult to determine which zeolite properties are most important for methane activation and methanol formation. However, well-distributed Cu ions,17 as well as an intermediate loading of Cu have been identified as important requirements to achieve the highest methanol yields.10,18 This is linked with the need to have Cu ions in suitable proximity to form both accessible and reducible multimeric species, while at the same time retaining a sufficient number of Brønsted acid sites to effectively aid in stabilizing the intermediates.19 Both monomeric,12,20 dimeric,18,21 and trimeric11,22 copper species have been proposed as the active sites,11,22 however, a redox mechanism involving mixed CuII/CuI sites in dimeric CuxOy-moieties, e.g. mono-μ-oxo dicopper(II), does seem to best meet the criterion of the two-electron process of converting methane to methanol.18,21,23,24
There are still many unanswered questions regarding the changes to the CuxOy-sites upon CH4 activation, H2O extraction, and subsequent reoxidation. Thus far, the literature indicates that CH4 activation proceeds via the formation of methoxy intermediates upon interaction with the Cu-sites that are stabilized on either Cu species or Brønsted acid sites nearby.25,26 Then, for the methanol formation, several groups have recently suggested a mechanism where the role of H2O is not only to displace the products but also to be the main oxygen donor.27–29 It is, however, well established that activation in O2 at high temperature leads to a fully oxidized CuII state of the zeolite. Further, it has been demonstrated that a fraction of the CuII is reduced to CuI when CH4 is introduced at e.g. 200 °C. However, only a fraction of the CuII is reduced, often around 30%.6,7,11,12,21,23 Upon introduction of H2O for the extraction of methanol, hydrolysis of Cu species have been reported, followed by a slow reoxidation of CuI back to CuII.23
Many different experimental and computational methods have been used to clarify the mechanism and pinpoint performance descriptors, as the stepwise nature of the reaction makes it very difficult to obtain kinetically relevant information.19,30 A potentially relevant method for the reaction is temperature-programmed reduction (TPR) with CH4. In a recent work by Sushkevich et al., they showed by comparing different zeolite topologies that FAU zeolites needed a higher CH4-loading temperature for the CuxOy-sites to be active towards methanol formation.15 Similarly, Kvande et al. relied on CH4-TPR to show that SAPO-34 needed a higher C–H activation temperature than the isostructural CHA zeolite, SSZ-13, to increase the methanol yield. This was associated with dissimilar Cu-sites or lattice components in the two CHA-type frameworks.31 As both of these previous studies compare different zeolite topologies, we found it intriguing to determine if CH4-TPR also could elucidate differences within the same zeolite system, but with different composition and MTM activity, to find relationships between structure/reducibility and activity. To that end, we chose two MOR zeolites with different Si/Al and Cu/Al ratio, well-characterized from several previous studies.18,23,32–34 To follow the Cu-speciation throughout the TPR protocol and connect CH4 oxidation to Cu reduction, we have coupled the TPR experiment with in situ X-ray absorption spectroscopy (XAS). Multivariate curve resolution alternating-least-square (MCR-ALS) analysis was performed to obtain the spectral signatures of pure Cu species contributing to the Cu K-edge X-ray absorption near edge structure (XANES) signal as well as their time/temperature-dependent concentration profiles. This is a technique that has given impressive, value-added input to understand the Cu-speciation in zeolites.35,36 Martini et al. used MCR-ALS analysis to identify differences in the Cu-speciation in a Cu-CHA when exposed to O2 or He during activation and was able to connect this with the reducibility of the samples.35 In another work by Pappas et al. on the same Cu-MOR investigated herein, it was shown with MCR-ALS analysis on high energy resolution fluorescence-detected (HERFD) XANES that two different framework-coordinated CuII species form at high temperatures.18 One of these species was linked to the CuII active site in Cu-MOR for MTM. To enhance our understanding of the Cu reducibility and broaden the applicability of our findings, C2H6 and CO were also employed as substrates/reductants in the TPR protocol, while probing the sample response by in situ XAS. C2H6-TPR was performed to deepen and expand our understanding of C–H activation in light hydrocarbons over Cu-zeolites beyond the iconic CH4 case. This is important since ethane, although much less abundant than methane, is the second largest component of natural gas, and there are large repositories of underutilized natural gas, especially from the abundant shale gas reserves. The industrially applied method for converting ethane is via steam cracking, which is a very energy intensive method leading to a high fraction of CO2.37 It is therefore important to find alternative pathways for converting ethane, where some suggested approaches thus far has involved oxidative and non-oxidative dehydrogenation of ethane to ethylene, aromatization, ammoxidation and also partial oxidation.29 In parallel, CO adsorption/desorption experiments on Cu-zeolites have been widely used to shed light on Cu-speciation and accessibility.32,38–41 Notably, CO also represents a possible overoxidation product in MTM schemes, and low-temperature dry CO oxidation to CO2 has been proposed as an effective probe reaction to assess the presence of multimeric CuxOy-moieties in zeolites.42–44 For all these reasons, CO-TPR studies were performed, under comparable in situ conditions.
In addition to XANES, also the longer energy range corresponding to extended X-ray absorption fine structure (EXAFS) was collected and included in the MCR-ALS analysis,45 allowing us to extract and analyze EXAFS spectra of the pure Cu components. An innovative approach, linking each pure EXAFS spectrum to an average temperature based on the MCR concentration profiles is implemented, to account for the inherent temperature dependence of the EXAFS signal in the interpretation of the results. Finally, Wavelet Transform (WT) analysis was used to untangle the different scattering contributions shaping the high-R region of the EXAFS spectra,34 thus providing unambiguous evidence for the nuclearity of the pure Cu-species formed in the MOR lattice. In situ XAS results are correlated with the CO2 formation and reactant consumption profiles obtained from Mass Spectrometry (MS) analysis and combined with complementary insights from Diffuse Reflectance ultraviolet-visible (UV-vis) and Fourier-transform infrared (FT-IR) spectroscopies.
2 Methods
2.1 Material properties
The two Cu-exchanged mordenites investigated herein were prepared from commercially obtained zeolites from Zeolyst. The 018Cu-MOR(7) was prepared from CBV10ADS (Na-MOR, Si/Al = 7) and the 036Cu-MOR(11) from CBV21A (NH4-MOR, Si/Al = 11). The zeolites were exchanged into their proton form before Cu-loading. The preparation and physicochemical characterization (scanning electron microscopy (SEM), energy dispersive X-ray (EDX) analysis, and powder X-ray diffraction (XRD)) of the materials have been described in detail in previous work from our group.18,33 The samples have also been subjected previously to an array of more advanced characterization involving CO-adsorption with FT-IR spectroscopy, XAS, X-ray photoelectron spectroscopy (XPS), and magic angle spinning (MAS) NMR spectroscopy.18,32,33,46 The sample nomenclature is given as [Cu/Al]Cu-MOR([Si/Al]). The Cu/Al, Si/Al, and Cu concentration (in μmol g−1) as measured by EDX are reported in Table 1. Also, the activity of the materials in the methane-to-methanol reaction from fixed-bed laboratory tests under optimized conditions is reported in Table 1. The values were first reported by Dyballa et al.33
Table 1 Relevant elemental composition and MTM performance results of the Cu-MOR samples
Sample |
Cu/Al |
Si/Al |
Cu (μmol g−1) |
MTMa yield (μmolMeOH g−1) |
MTMa productivity (molMeOH molCu−1) |
MTM yield and productivity as previously reported.33
|
018Cu-MOR(7) |
0.18 |
7 |
367 |
172 |
0.47 |
036Cu-MOR(11) |
0.36 |
11 |
500 |
124 |
0.25 |
2.2
In situ XAS temperature-programmed reduction (TPR) experiments with CH4, C2H6, and CO
TPR experiments were conducted in thin capillary reactors simultaneously as X-ray absorption spectra were collected. About 6 mg of sample were pressed and sieved (160–125 μm) and placed into a quartz capillary (1.5 mm i.d. and 0.01 mm thickness) with a quartz wool plug at each end of the bed. The capillary was then glued to a special-designed bracket so that gas could flow through. The capillary was heated with a heat blower placed directly under the reactor bed. The temperature set points were calibrated with a thermocouple placed inside an empty capillary before the experiments. Three mass flow controllers (MFCs) together with automatic 4-way valves (kept at 120 °C to remove traces of moisture) were used to control the flows of He (100%), O2 (100%), and either CH4 (2%/rest. He), C2H6 (3%/rest. He), or CO (5%/rest. He). All flows were set to 5 ml min−1, and the effluent was analyzed with an online MS. The reaction protocol is depicted in Scheme 1. Before the TPR ramp, the samples were heated to 500 °C in O2 and kept in these conditions for 2 h before it was first cooled (10 °C min−1) to 200 °C and subjected to He for 30 min to clean away possible contaminants when switching the automatic 4-way valve between O2 and He. Then the samples were cooled further to 120 °C and kept in inert treatment for 1 h before being exposed to the reducing agent. After reaching a steady trace (∼20 min) in the MS, the samples were heated at 5 °C min−1 up to 550 °C.
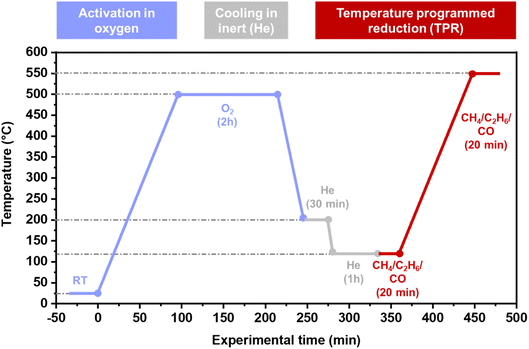 |
| Scheme 1 Experimental protocol for in situ XAS during TPR experiments over Cu-MOR samples. The protocol consists of activation in oxygen at 500 °C, followed by two cooling steps. First, the sample was cooled in oxygen until 200 °C before a second cooling step to 120 °C in inert (He). Then the samples were exposed to a reductive atmosphere (either CH4, C2H6, or CO) while the temperature was ramped up (5 °C min−1) to 550 °C. | |
The Cu K-edge in situ XAS data were collected in transmission mode on the BM31 beamline of the European Synchrotron Radiation Facility (ESRF, Grenoble, France).47 The beamline was equipped with a water-cooled double-crystal monochromator (flat Si[111] pair) and ionization chambers, filled with a He/Ar mixture, were used for the detection of the incident (I0) and transmitted photons (I1).48 All the XAS spectra were collected with an acquisition time of ca. 3 min per scan. The obtained data were normalized to unity edge jump at the Cu K-edge by the Athena software from the Demeter suite.49
2.3
In situ ultraviolet-visible (UV-vis) spectroscopy of CH4-TPR experiments
Supporting CH4-TPR experiments coupled with UV-vis were performed on a purpose-designed test rig for in situ and operando UV-vis gas-phase experiments, where the probe is placed into the middle of an oven, touching the tubular quartz reactor (9 mm i.d.) perpendicular to the reactor bed. About 100 mg of pressed and sieved (500–250 μm) sample was prepared and placed inside the quartz reactor on a glass wool plug. The temperature was controlled by a thermocouple touching the pelletized sample bed. The spectra were recorded in reflectance (%) mode on an Avantes AvaSpec-ULS2048XL-EVO fiber optics spectrometer (100 μm slits) with an Avantes AvaLight-DH-S light source (including both a deuterium and a halogen lamp). For the collection of diffuse reflected light from the sample, a custom high-temperature resistant fiber optic with a high-OH fused silica core (diameter = 100 μm) was used. Polytetrafluoroethylene (PTFE) powder was measured at room temperature (RT) as a 100% reflectance reference before the TPR experiments. The same protocol as reported in Scheme 1 was followed. For the ramp, a flow of 15 ml min−1 CH4 (20%/rest. He) was applied. UV-vis spectra were collected continuously at all steps of the protocol. For the TPR ramp, one spectrum, obtained as the average of 50 scans with 10 ms integration time each, was collected every 50 s.
2.4 Multivariate curve resolution-alternating least squares (MCR-ALS) analysis of in situ XAS data
XAS uniquely gives access to local structural information without any need for long-range order in the Cu sites distribution.50,51 However, in the presence of different Cu species in the zeolite, the technique provides an average signal over all the Cu sites in the mixture. In this regard, Multivariate Curve Resolution-Alternating Least Squares (MCR-ALS) analysis represents a powerful method to deepen the characterization, by resolving individual contributions from pure Cu species. MCR-ALS allows the decomposition of an experimental matrix D into pure contributions, such as spectra S matrix and the corresponding concentration profile C matrix: D = C·ST. Initially, the number of principal components (PCs) must be determined following a critical assessment of the results in terms of spectroscopic and chemical–physical meaningfulness.52–54 The principal results that MCR-ALS returns are concentration profiles and spectra of pure components. The generation of the pure components is performed by initial guesses efficiently managed by specific methods, such as SIMPLISMA.55
In the present work, the whole experimental energy range (8800–9727 eV), including both the XANES and the EXAFS spectral regions, of normalized and energy-aligned XAS spectra was analyzed by MCR-ALS. The normalized μ(E) XAS spectra, starting from the pretreatment step under static conditions in O2 at 500 °C, were organized in three datasets according to the reducing agent used during the TPR step, joining the datasets related to the two investigated Cu-MOR samples. MCR-ALS reconstruction was carried out using the MATLAB-based MCR-ALS Graphical User Interface (GUI) developed by Jaumot et al., using Matlab R2022a.56 For further details on the specific method implementation and the ALS quality control parameters, the interested reader is referred to ESI, Section S2 and S3.†
2.5 Wavelet transform (WT) EXAFS analysis
Fourier-Transform (FT-)EXAFS analysis is traditionally employed to access detailed information about the local structure and coordination environment around a specific metal center.57 However, when different neighboring atoms are localized at similar distances around the absorber, their scattering contributions can overlap in R space, becoming indistinguishable.58,59 In the present case, such overlap issues are especially present in the 2.5–3.5 Å range, involving framework T atoms (Si, Al) and Cu atoms from multimeric Cu-species formed in Cu-MOR at high-temperature treatment in O2. A possible solution to overcome this problem is to associate the conventional FT-EXAFS to its k-space EXAFS signal, thus enhancing the sensitivity to the chemical nature of the scatterer by exploiting the scatterer Z-dependency in the backscattering amplitude functions, F(k).60,61 This task can be performed by an innovative spectral processing technique called Wavelet Transform (WT) analysis. With this technique, a 2D representation of the EXAFS, showing k- and R-space spectral features simultaneously is possible.57 The mother function that is used to obtain the WTs maps and the related power density functions is the so-called Morlet function: | 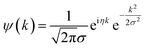 | (1) |
where i denotes the complex unit, and η indicates the frequency of a sine wave convoluted by a Gaussian curve with σ as the standard deviation.34 The selected values of the η and σ parameters regulate the resolution in R and k space, allowing the operator to achieve the best visualization of the desired spectral features.62 In this regard, knowing that the typical Cu–Cu distance in dicopper(II)-oxo species formed in zeolites falls in the 3–4 Å range (average values of 3.5 Å), the best resolution condition at a given distance of interest is obtained choosing σ = 1 and η = 7 for all the WT representations.60,63,64
To more easily compare different spectra, focusing on the presence and properties of Cu–Cu contributions, the so-called power density function ΦR(k) of the WT representation was also calculated.34,65 This function represents integrals of the squared modulus of the WT over the R-space range of interest, according to the following definition:
| 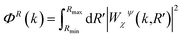 | (2) |
where
Wψ(
k,
R) is the WT representation of the EXAFS signal using the mother function
ψ, and the integral is computed from
Rmin to
Rmax, here set to 2.5 and 4.0 Å respectively.
2.6
In situ Fourier transform infrared (FT-IR) spectroscopy of CO-TPR and desorption experiments
The CO-TPR experiment was conducted on a Bruker Vertex 80 instrument equipped with a Mercury–Cadmium-Telluride (MCT) detector cooled with liquid nitrogen. The material was pressed into a thin, self-supporting wafer (7.5 mg cm−2) and placed inside an AABSPEC cell with low free volume (model #CXX), where both temperature and gas flow can be controlled. For the TPR experiment, the sample was heated to 500 °C (5 °C min−1) in a flow of O2 (100%), before being cooled down to 120 °C (10 °C min−1), where He (100%) was purged on the sample for 1 h. Then, the gas flow was switched to CO (10%/rest. He) and ramped up to 500 °C (10 °C min−1). A spectrum was collected every 40 s. To calculate the CO concentration (in μmol g−1), the following form of Lambert–Beer law was applied:where A (cm−1) is the integrated absorbance defined in a specific spectral region, ρ (mg cm−2) is the pellet density, ε (cm μmol−1) is the molar attenuation coefficient (11.5 cm μmol−1) obtained from Deplano et al.,40 and C (μmol g−1) is the concentration of CO.
3 Results
3.1
In situ XANES of Cu-MOR during CH4, C2H6 and CO-TPR: a qualitative overview
Two MOR zeolites, 036Cu-MOR(11) and 018Cu-MOR(7), with different compositions and MTM activity (Table 1), were subjected to three different TPR experiments. By following the protocol presented in Scheme 1, CH4, C2H6, and CO-TPR were performed. XAS spectra were collected throughout the entire experiment. The Cu K-edge XANES spectra collected during pretreatment in O2 and subsequent cooling step are reported in the ESI, Section S1.† Before O2 activation, in the as-prepared Cu-MOR zeolites, Cu ions mostly occurred as mobile hydrated CuII complexes.11,18 Then, as expected, during the high-temperature activation step in O2, the Cu ions progressively turned into framework-coordinated CuII species with three/four-fold coordination.46,66 When cooling from 500 to 120 °C, the CuII oxidation state is fully preserved. Small modifications in the spectral shape are observed and are suggested to stem from temperature-dependent rearrangements in the coordination motif for a small fraction of framework-coordinated Cu ions. This has already been observed for Cu-MOR under equivalent conditions.23,46Fig. 1 reports the XANES spectra obtained during the TPR protocol for the three different reducing atmospheres (CH4, C2H6, and CO). In all cases, the reduction of Cu is visible. Spectroscopically, this is indicated by the progressive decrease of the pre-edge peak at 8977 eV related to the 1s → 3d transition in CuII ions (see ESI, Section S1† for further details), paralleled by the development of a prominent CuI 1s → 4p rising-edge peak located at ca. 8983 eV.61 The intensity loss in the white line (WL) peak region indicates a decrease in the average coordination number of the absorber.67 This is consistent with the progressive formation of linear or quasi-linear CuI species.23
 |
| Fig. 1 XANES TPR spectra for each sample/reducing agent combination. The temperature was ramped up (5 °C min−1) from 120 °C to 550 °C for 1.5 h. The increased intensity of the rising edge 1s → 4p peak related to CuI observed in all spectra, indicates the progressive formation of CuI species during the TPR experiments. A more complex situation is visible in the case of CO, in which an intermediate CuI species is detected (black boxes). | |
During the reduction in CO, qualitative XAS analysis suggests a more complex reaction pathway. The appearance of an intermediate CuI species is detected in the XANES spectra and highlighted by the black boxes in Fig. 1, right panels. The characteristics of this species will be discussed in the following, based on MCR-ALS results. At the end of the TPR protocols (550 °C), signals related to characteristic CuII transitions are no longer visible in any of the experiments. Based on this, we qualitatively confirm that a virtually pure CuI state was reached at the end of the TPR protocol. Comparing the two Cu-MOR compositions, the reaction led to the development of very similar spectral features in both samples, and it was therefore evident that more advanced analysis was necessary to search for composition-dependent differences in the spectra.
3.2 Finding spectroscopic fingerprints of different Cu-species with MCR-ALS analysis
By employing MCR-ALS analysis on the in situ XAS data, we obtained quantitative information on the Cu-speciation throughout the TPR experiments as this advanced data analysis technique provides detailed insight into the speciation and dynamics of the Cu species inside the zeolite.19,68 The MCR-ALS analysis yielded a total of six principal components. The pure XANES spectra of the six components are reported in Fig. 2a, while their concentration profiles in the different experiments are reported in Fig. 2b. A pictorial representation of possible Cu-species linked with the pure XANES spectra discussed is finally provided in Fig. 2c. Firstly, focusing on the O2 activation step of the protocol, two high-temperature (HT) CuII species, CuII_HT_a and CuII_HT_b, are recognized with very similar XANES signatures. The biggest difference is the shape and intensity of the WL, supporting a conserved coordination motif with only subtle differences in the first-shell bond distances and angles. It is plausible that they could stem from the same Cu-moiety, e.g., a mono-μ-oxo dicopper(II) core, but docked at distinct zeolitic exchange sites, also featuring different accessibility. The third CuII species recognized, CuII_LT, is described as a low-temperature (LT) CuII species, since it increases in concentration with a decrease in temperature. CuII_LT is likely to be more highly coordinated than the high-temperature species, based on the higher WL intensity observed in this case. Interestingly, the growth of CuII_LT appears to correlate with a decrease in the concentration of CuII_HT_a, while CuII_HT_b remains almost stable. We conjecture that the concentration of CuII_LT increases with decreasing temperature due to a dynamic change in some of the CuII_HT_a species, leading to a change in coordination motif from three-fold to four-fold. A minor peak in the typical CuI energy region (8983 eV) is visible in the pure CuII_LT XANES spectrum and is highlighted with an asterisk (*). This spurious spectral feature is an artifact from the MCR-ALS analysis due to a high abundance of CuI in the mix of Cu species, previously documented by Martini et al.69
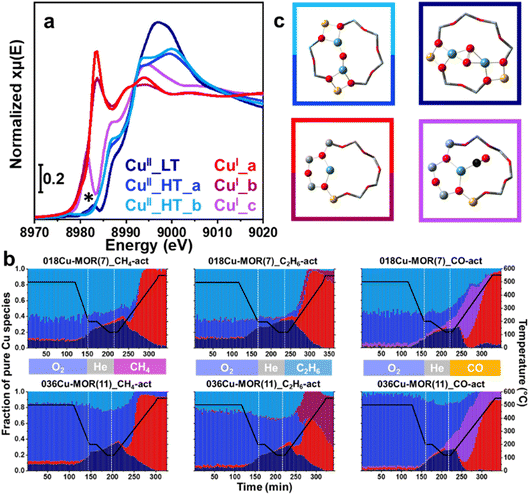 |
| Fig. 2 (a) Global representation of the pure XANES spectra of Cu species obtained by MCR analysis, performed considering the whole dataset except for the ramp from RT to 500 °C during the activation step. The * symbol indicates an MCR reconstruction artifact. (b) Corresponding temperature-dependent concentration profiles for each Cu species, in which the evolution of the common species follow the same trends: CuII_HT_b is more abundant than CuII_HT_a in the 018Cu-MOR(7) sample, while the opposite is observed in 036Cu-MOR(11); CuI_a at the beginning of TPR forms preferentially from the CuII_HT_b species. In the CO case, the CuI_c intermediate species is visible in the first part of the TPR. (c) Pictorial representation of the Cu species proposed to form during the experiment. Atoms color code: Cu, green; O, red; C, black; Si, grey; Al, yellow. | |
When comparing the concentration profile for CuII_HT_a and CuII_HT_b between 018Cu-MOR(7) and 036Cu-MOR(11) during the O2 activation, they behave similarly throughout all experiments, with a higher concentration of CuII_HT_b in 018Cu-MOR(7). Importantly, this is fully consistent with previous results by Pappas et al., where a framework-coordinated CuII species with a Cu K-edge spectral signature consistent with CuII_HT_b, was found to be more abundant in the more productive 018Cu-MOR(7) sample, and tentatively associated with the active site for the MTM reaction.18 Peculiarly, there is some discrepancy in the concentration profiles during the activation step for the CO-TPR experiment, where a higher concentration of CuII_HT_a is observed for both Cu-MOR zeolites. However, we allocate this error to uncertainties in the MCR reconstruction, possibly due to the high spectral similarity between CuII_HT_a and CuII_HT_b.
Upon shifting our focus to the TPR step of the protocol, it can be noted that new Cu-species develop, as CuI species form at the expense of CuII.17 Depending on the experiment, three distinct principal components were detected, where CuI_b and CuI_c formed exclusively in C2H6 and CO, respectively. The two species denoted as CuI_a and CuI_b show similar spectral features, with a typical rising edge peak assigned to the 1s → 4p transition of CuI, and no trace of the d9 1s → 3d pre-edge peak (Fig. 2a). The WL intensity is significantly lower than for the CuII species, indicating Cu sites with lower coordination number. Indeed, the spectral features are very similar to (quasi) linear Cu sites coordinated with two lattice O atoms.70 The minor modifications in the XANES spectral shape between CuI_a and CuI_b are likely related to small induced differences in bond length and/or minor rearrangements when the sites are perturbed by the different gaseous species (CH4vs. C2H6). The XANES spectrum of the third CuI species, CuI_c, has very different spectral features from the other two, where the 1s → 4p peak is shifted towards lower energy values. This spectral fingerprint, clearly distinguishable in the experimental data, initially suggested the presence of an intermediate species during the CO-TPR ramp (Fig. 1). This implies that the coordination geometry and environment of this species is considerably different. When studying the concentration profile, Fig. 2b, we observe how the species reaches a maximum at about 260 °C and then slowly diminishes up until ∼400 °C. Due to the change in the coordination environment of this species compared to CuI_a and CuI_b, we conjecture the species is related to mono- or dicarbonyl complexes forming in the presence of CO, as CO is known to form stable adducts with CuI sites in zeolites.39,40 A small fraction of CuI_c species is still detected above 400 °C for 036Cu-MOR(11), however, this quantity falls within the uncertainty typically associated with MCR-ALS reconstruction (∼10% of total Cu), and can therefore not be ascertained as a stable species.69
Lastly, it should be noted that when the CuI species start forming in the CH4- and C2H6-TPR experiments, we see the consumption of CuII_HT_b slightly before the consumption of CuII_HT_a. This observation indicates that CuII_HT_b is more easily reduced upon alkane activation, and as this is the dominant CuII species in the most MTM active 018Cu-MOR(7) sample, we hypothesize the Cu reducibility to be linked with the activity of the samples. This hypothesis is considered even more closely in the following section, as we attempt to look more meticulously at the overall reduction trends during the TPR ramp.
3.3 Coupling CuII to CuI reduction with reactant consumption and product formation
To elucidate more easily the minor differences in the TPR protocol step between the two MOR compositions and the different substrates, the total CuI fraction during the TPR ramp is plotted in Fig. 3. The data points are derived by summing the fractions of all the pure CuI species as determined by the MCR-ALS analysis above for each XANES spectrum collected. The CuI fraction in 018Cu-MOR(7) and 036Cu-MOR(11) as a function of temperature during TPR is compared for all three reactants, CH4, C2H6, and CO. In the bottom panel of Fig. 3, we report the first derivative of the trace (d(CuI tot.)/dE) to emphasize the reduction rate. Several interesting differences may be observed. Foremost, we observe that the onset of Cu reduction varies depending on the substrate in the order of CO < C2H6 < CH4, where the CH4 atmosphere leads to the highest reduction temperature. This is quite natural given the stronger bond dissociation energy of CH4 (439 kJ mol−1) compared to C2H6 (422 kJ mol−1).71 As the behavior of Cu in CO is markedly different, we will discuss further the CO-TPR event at the end of the section, and for now, focus on the results obtained with the two alkanes as reducing agents.
 |
| Fig. 3 Comparison of MCR-derived total CuI fraction as a function of temperature for each sample/reactant combination (top panel) in the CH4-, C2H6-, and CO-TPR steps, and corresponding first derivative (bottom panel) to better identify inflection points in the CuI total fraction profile. | |
At first glance, the two Cu-MOR samples are behaving very similarly. Nevertheless, upon close examination, some small, but consistent differences can be observed. First, 036Cu-MOR(11) has a higher maximum reduction rate. This is specifically evident in the derivative plotted in the bottom panel of Fig. 3. Secondly, we observe that 018Cu-MOR(7) appears to have a marginal temperature region initially, where Cu is reduced faster than for 036Cu-MOR(11). This is consistent with the CuII_HT_b species reducing faster than the other CuII species, as observed in the previous section. This earlier reduction behavior in 018Cu-MOR(7) could be sought-after evidence of more readily reducible species in the highly active Cu-MOR material.18,33 A high “self-reduction” potential has been linked directly to the materials' methanol productivity in other framework topologies like Cu-exchanged FER and CHA.12,14 This however, has not been observed for the Cu-MOR zeolites presented herein, as previously reported results have indicated that 018Cu-MOR(7) has more “self-reduction” resistant species, which then were coupled with well-distributed Cu-oxo species with high coordination and uniform bond lengths.18,32 It should be noted, however, that the above-mentioned studies concern the reducibility of Cu-zeolites in either vacuum or inert atmosphere. Conversely, herein we are linking the high methanol productivity of 018Cu-MOR(7) directly to the reducibility of the Cu species in a methane atmosphere. This elucidates the importance of working with as relevant conditions as possible. Moreover, the higher reducibility observed for 018Cu-MOR(7) herein is in full agreement with Lomachenko et al. who found that more CuII reduced to CuI after methane loading for the 018Cu-MOR(7) material compared to 036Cu-MOR(11), when investigating the samples with XAS in a full MTM cycle.23
Upon comparing the reduction rate of CuII to the substrate consumption and CO2 production obtained simultaneously from MS analysis of the reactor outlet (Fig. 4), interesting features are found. First, during CH4-TPR, both CO2, from over-oxidation of CH4, as well as the consumption of CH4, appear in the same temperature window as the CuI formation. This direct link between CH4 consumption, CO2 production, and CuI formation contrasts with previously reported results by Sushkevich et al.15 They observed a temperature region where CH4 was consumed before CO2 production over Cu-MOR (200 > 325 °C) and signified this window to be linked with oxidation processes of partially oxidized methane intermediates. However, as we observe the complete oxidation occurring simultaneously with CuI formation and CH4 consumption, we speculate that with a continuous temperature ramp, the over-oxidation occurs too fast to be singled out from the CH4 consumption and CuII reduction in a meaningful way. Nevertheless, about 10% of the total CuI fraction is forming before the onset of CH4 consumption (below 250 °C). We postulate this minor CuI fraction, yet very close to the uncertainty of the MCR-ALS method, to possibly come from a “self-reduction” of dimeric CuII-oxo sites to CuI, as made possible by an electron-rich environment after O2 activation, where O2− ions have remained stabilized in the framework.72 We must point out that the results also show small traces of CO2 produced in this low-temperature window. However, we allocate this to an effect of the low signal resolution caused by the small amounts of material and gas flows used in the experiment. Also due to this, we cannot exclude the possibility that some CH4 (not visible in the CH4 trace) is being consumed already from around 200 °C when Cu is being reduced. Regardless, some CuI is forming before consumption during C2H6-TPR, especially discernible for 018Cu-MOR(7), supporting the “self-reduction” event suggested above. Another intriguing observation during C2H6-TPR is that there is a secondary region, where no more ethane is being consumed, but there is still a reduction of Cu, as well as continued production of CO2. This behavior indicates that when ethane acts as a substrate, there are secondary oxidation processes with fragments from ethane oxidation, that are less potent reducing agents, occurring on remaining CuII sites that did not react directly with ethane or were somehow not accessible to ethane.
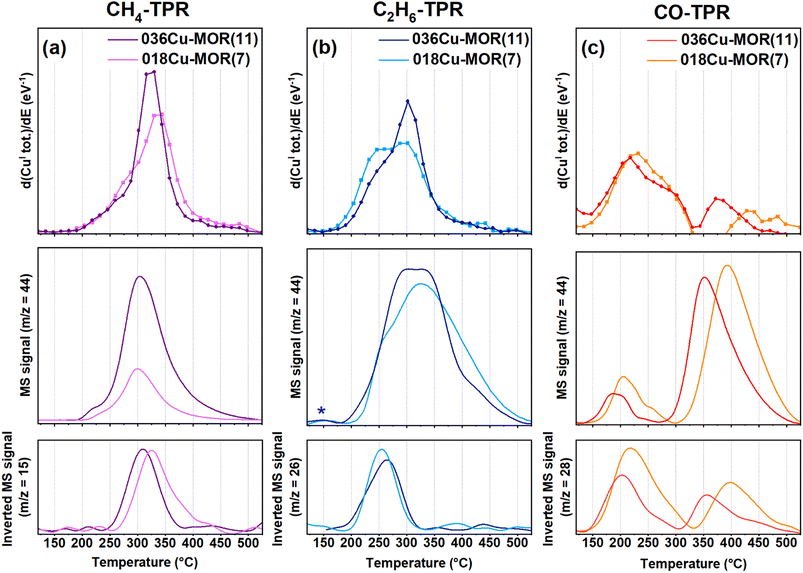 |
| Fig. 4 (a–c) Comparison of the first derivative of the CuI fraction (top panel) to the CO2 formation (middle panel) and reactant consumption (bottom panel) during the TPR protocol with (a) CH4, (b) C2H6 and (c) CO. The MS trace used for CO2 is m/z = 44, while m/z = 15, 26, and 28 is used for CH4, -C2H6 and CO, respectively. The MS traces have been normalized to the sample weight and Cu content. *The CO2 trace for 0.36Cu,H-MOR(11) during C2H6-TPR has been collected in a parallel experiment in the home laboratory, due to technical problems with the trace obtained during the XAS experiment. | |
Coming back to the CO-TPR protocol, two reduction regions are observed. The primary reduction starts directly upon contact with CO and leads to ca. 80% of reduced CuII. Then as the temperature increases, a secondary reduction event happens at higher temperatures, involving the residual fraction of ca. 20% of total Cu. For 018Cu-MOR(7), an apparent transient reoxidation involving <10% CuI can be observed at high temperatures. However, given the unlikely nature of this event, we assign this peculiar behavior to an error in the reconstruction of the XANES spectra. In contrast to the behavior of the Cu-zeolites in the alkane atmosphere, it appears the Cu-sites in 036Cu-MOR(11) reduce in a lower temperature range. Focusing on Fig. 4, we surmise that the first, broad reduction peak is linked with the above-suggested formation of CuI–(CO)x (x = 1 or 2). This is also well supported by a clear consumption of CO, and a smaller CO2 production coming from the oxidation at the Cu-oxo sites. Then, during the high-temperature reduction event, we do see a much larger fraction of CO2 being produced in combination with a smaller consumption of CO. As most Cu already has been reduced to CuI at this point, we postulate that the production of CO2 is linked with trapped CO2 being released as the temperature increases, or possibly the release and oxidation of CO from CuI–carbonyls. This could proceed via a reaction with residual O2− present, or by a disproportionation reaction between two CO molecules in a Boudouard reaction.73 As we do not have any evidence for the formation of elemental C, the latter reaction pathway is only speculations by the authors at this point.
3.4 Complementary in situ UV-vis TPR experiments
To support the observations presented in the previous sections, a complementary experiment was performed in-house with a purpose-designed in situ UV-vis setup. The UV-vis spectra were obtained in diffuse reflectance mode during a CH4-TPR experiment for both 018Cu-MOR(7) and 036Cu-MOR(11) and are reported in Fig. 5. Even though we here operated with a different sample amount and gas flow, the CH4 consumption and CO2 production followed closely the same temperature window (∼250–400 °C) as obtained during the in situ XAS experiments. The MS traces for CH4 and CO2 are reported in the ESI, Section S6.† Interestingly, we are also seeing the biggest changes in the UV-vis spectra in the same temperature window. We observe that at about 250 °C, three bands at 20
250, 16
850, and 13
000 cm−1 grow, reaching a maximum at about 325 °C. This is also approximately the temperature at which a maximum is observed for CH4 consumption and CuI formation rate during the XAS experiment. At 325 °C, the bands decrease again, giving the biggest evidence of reduction from CuII to CuI, as this is what one would expect when the Cu ion goes from a d9 configuration to a d10 configuration, where the d–d electron transfer is forbidden. When the temperature reaches about 400 °C, the changes slow down, and mostly temperature-induced shifts in the ligand to metal charge transfer (LMCT) region (25
000–45000 cm−1) are observed from 450–550 °C.74 The final state reached at 550 °C shows for both samples a rather flat profile in the d–d spectral range, consistent with the virtually pure CuI state revealed by XAS. The weak negative features still observed at the end of the TPR around 16
000 cm−1 most likely stem from a combination of high-temperature background fluctuations and, possibly, residual traces of highly absorbing CuII species (vide infra), with abundance below the XAS detection limit.
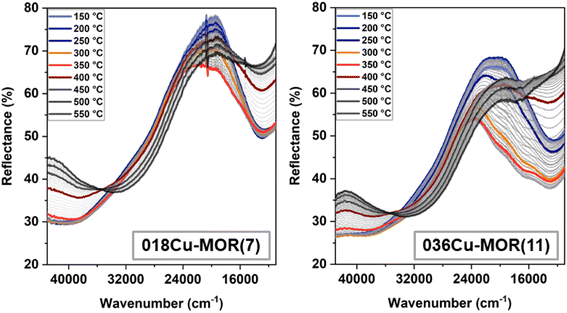 |
| Fig. 5
In situ UV-vis spectra were obtained during a TPR protocol with CH4 for the two Cu-MOR samples. The spectra are overlayed, with a thick line, and different colors are used for every 50 °C to clarify the change in the spectra throughout the protocol. Blue colors are for low temperatures, red for intermediate temperatures, and dark grey for high temperatures. | |
The growth of the bands in the d–d region between 250 and 325 °C, especially visible in 036Cu-MOR(11), is counter-intuitive, as it could be at first glance interpreted as an increase in the abundance of CuII species. However, the combination of these three bands shares similarities with the so-called “multiplet” formation also observed previously in Cu-CHA during O2 activation.75 The literature suggests that the multiplet formation is linked with a diluted CuII species with a very strong extinction coefficient.76 Interestingly, it is evident from the band at 38
500 cm−1 that the species must also affect the LMCT region, as there is no evidence of reduction here before the temperature reaches 325 °C. Nevertheless, we conjecture that even though this species is forming, it is likely to involve a minor fraction of total Cu ions, and there is still a reduction process occurring before T = 325 °C, as evidenced by the XAS experiments. To the best of our knowledge, the observation of a multiplet has not previously been reported for Cu-MOR. The CuII species is temperature dependent, and forms, contrary to Cu-CHA, in a CH4 atmosphere. Why this species is much more prominent in 036Cu-MOR(11) than in 018Cu-MOR(7) would only be subject to speculation at this point, but it is alluring to suggest it is linked with the CuII_HT_b, as this is much more abundant in the 036Cu-MOR(11) sample.
3.5 MCR-ALS-derived EXAFS spectra
By analyzing the EXAFS region, we get further information on the local environment of Cu ions after key steps in the TPR protocols (Scheme 1). Since the results thus far have shown the same Cu species in 018Cu-MOR(7) and 036Cu-MOR(11), we decided to combine data obtained over both zeolites to optimize the signal quality in the EXAFS region. As we had performed an MCR reconstruction on the whole data set, including the EXAFS energy range, the EXAFS spectra could be obtained directly from the MCR-derived μ(E) spectra by following a recently reported approach.45 This allowed us to get EXAFS signals of the pure Cu species dominating Cu-speciation in the key experimental steps. The EXAFS signals were then extracted with standard procedures and visualized in both k- and R-space (Fig. 6). To guarantee signal reproducibility while avoiding as much as possible high-k noise, the Fourier Transform was performed in a narrow k-range from 2.5–9.0 Å−1 (grey box in Fig. 6a), see ESI, Section S5† for more details. The collection temperature is known to strongly influence the EXAFS oscillation intensity, being increasingly dampened as temperature increases.77 This is due to an effect of the Debye–Waller factor in the EXAFS equation. As the MCR-ALS derived EXAFS spectra are obtained from the collective analysis of a temperature-dependent dataset, we have defined an average temperature associated with each spectrum to guide the interpretation of the EXAFS spectra. In particular, for each ith pure species, a reference average temperature, Tavgi, representing the weighted average temperature using the MCR-derived concentration of each scan in the dataset as weights, was calculated (ESI, Section S4†). The temperature dependence is evident in the k2χ(k) spectra reported in Fig. 6a. All HT CuII (CuII_HT_a and CuII_HT_b) species show dampened oscillations compared to CuII_LT. This is consistent with the latter having a significantly lower Tavgi value (254 °C vs. 385 and 326 °C, respectively for the HT CuII species). Then, compared to the CuII species, the k2χ(k) curves of all CuI species present lower signal amplitudes and detectable frequency differences, regardless of temperature. Hence, we point out that the oxidation state of the absorbing atom, although not directly monitored by EXAFS, is relevant for further discussion, as it influences the average number and distribution of atomic neighbors and, to some extent, their interatomic distance from the Cu centers. Finally, we note that the EXAFS spectra in k-space of CuI_a and CuI_b, as well as CuII_HT_a and CuII_HT_b, are very similar, again pointing to the structural similarities between these species as suggested above.
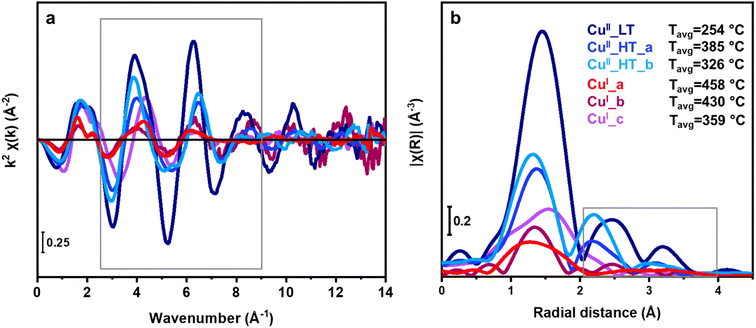 |
| Fig. 6 (a) MCR-ALS derived k-space EXAFS spectra for the pure Cu species identified. The k range used to calculate the FT, 2.5–9.0 Å−1, is highlighted by the grey box. (b) Corresponding FT-EXAFS spectra in R-space, obtained by Fourier transforming the k2χ(k) curves reported in (a). According to the structure of the zeolite, the peaks highlighted by the grey box could be related to Al, Si. and the farthest O atoms from the MOR lattice or to other extra framework copper atoms participating in multimeric Cu species. | |
By analyzing the FT-EXAFS spectra shown in Fig. 6b, we can get more information on the Cu environment of the different pure components. The first-shell peak corresponds to single-scattering (SS) contributions involving framework (Of) and extra-framework oxygen (Oef) atoms.78 A higher intensity in the first shell should be linked with higher Cu coordination and a lower Tavgi temperature, which fit well with the proposed CuI and CuII speciation, based on the MCR-ALS derived pure XANES spectra (Fig. 3b). In addition to CuI_c having a higher first-shell peak than the other CuI species (indicating higher coordination), the structured shape of the first-shell peak suggests the presence of another scattering contribution in addition to Of and Oef. The observed shift of the first shell maximum to higher R is also consistent with an earlier study of CO interacting with CuI-ZSM-5.79 Combined with the XANES results indicating a CuI–CO formation, we accredit this contribution to the carbon atom in a CO ligand.
A well-defined second shell peak is visible in the CuII species. This we attribute, based on previous studies, to a framework bound Al near the Cu atoms (ca. 2.8 Å).80 Importantly, the presence of this signal for the CuII_LT FT-EXAFS eliminates the possibility of CuII_LT being a mobilized, hydrated CuII species, as hydration of Cu would dampen this signal. In CuI_a and CuI_b, the second-shell region is strongly dampened. This is consistent with previous studies on “self-reduced” Cu-MOR, causing a higher degree of structural disorder in the CuI cation local environment, which ultimately led to destructive interference between the EXAFS scattering paths.34,70,80
The third shell peak (3.0–3.3 Å), although, only visible for CuII species, could stem from several types of scattering contributions with similar radial distances like framework Si/Al/O atoms or Cu atoms in multimeric moieties. To unravel the contributions in the third shell, we performed Wavelet Transform (WT) analysis on the EXAFS data.
3.6 Shedding light on the nuclearity of Cu-species with WT-EXAFS analysis
The limitations of conventional, FT-EXAFS analysis in the assignment of the third shell EXAFS contributions can be solved by performing a WT-EXAFS analysis, where information on interatomic distances from the R-space is combined with element-specific information obtained from k-space.57–59 In k-space, Cu–Cu scattering paths can be discriminated from those involving framework Si/Al/O atoms due to a different EXAFS backscattering amplitude function (F(k)) of the elements, leading to a k-value that is element-specific. The typical Cu–Cu distances of dicopper(II)-oxo species in zeolites is ∼3.5 Å.34,80 An example of the resulting WT-EXAFS density maps is depicted in Fig. 7. We remind that this and all the WT-EXAFS maps reported herein are obtained by applying a Morlet Wavelet Transform (see Section 2.5) to the MCR-derived k2χ(k) pure EXAFS spectra reported in Fig. 6a. In part (a), the full-range WT-EXAFS map for a representative pure Cu species, CuII_HT_a, is given. The first lobe located at Δk (0–12.5) Å−1 × ΔR (0.5–2.0) Å is from Of and Oef first shell SS contributions. The elongation effect of this low-R first lobe towards higher k-values is due to spectral broadening, well explained by Timoshenko et al.59 The more interesting high-R EXAFS contributions are magnified in Fig. 7b for better visualization. The F(k) functions for the relevant atoms (O, Al, Si, and Cu) are presented above in Fig. 7b to aid in the sub-lobe assignment. Two sub-lobes are detected. The low-k sub-lobe (∼3 Å−1) indicates the contributions of lighter elements (O, Al, and Si), while the second, high-k sub-lobe at ∼7 Å−1, fit well with the maximum of the Cu backscattering function and can undoubtedly be assigned to Cu–Cu scattering contributions.60,61
 |
| Fig. 7 (a) Full-range WT representation of the EXAFS signal for a representative pure Cu species, namely CuII_HT_a, formed at high temperature during the pre-treatment step. (b) Magnification of the high-R WT region highlighted by the red dashed box in (a), in the ranges Δk (0–9) Å−1 and ΔR (2.5–4.5) Å. On the top is reported a plot of the relevant backscattering amplitude functions, F(k), calculated from FEFF6.0 considering SS paths involving O, Si, Al, and Cu scatterers.34 | |
To compare the WT-EXAFS maps of the six pure Cu species more easily, the maps were integrated in the relevant R-space range, giving the power density functions of the Cu species. The power density functions are reported in Fig. 8, together with the WT-EXAFS maps of the high-R EXAFS region for all Cu species. In both representations, the position of the sub-lobes provides valuable information on the elemental identity of the atomic neighbors surrounding the Cu absorber in the radial distance of interest. When comparing the power density functions of the CuII species, they all have detectable Cu–Cu contributions, visible from the high-k sub-lobe, in the accessible R-space range, leading us to describe these species as dicopper(II) moieties (or proximal CuII monomers), also in line with previous literature proposals.3,18,60,61 Consistently with previous studies on the use of CO dry oxidation to titrate dicopper(II) species in zeolites,42,43 having most of the Cu organized into dimeric species at the end of the oxidative pre-treatment falls in line with the effective reduction observed during CO-TPR, already in the 150–300 °C range.
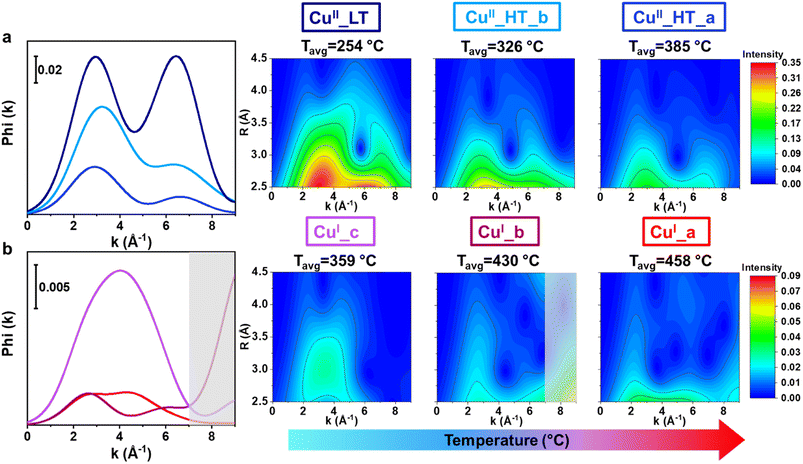 |
| Fig. 8 (a and b) Power density functions (left) and WT maps (right) obtained by WT-EXAFS analysis for (a) CuII species and (b) CuI species. For each ith species, the average temperature (Tavgi) is reported. The shadowed grey boxes in (b) highlight the high-k range affected by possible artifacts due to poor S/N ratio in the MCR-derived k2χ(k) curves for CuI_b. | |
We observe that the signal intensity of the CuII species decreases with increasing temperature (Tavgi) in the whole k-range, which is consistent with the temperature dependence of EXAFS spectra and cannot be directly linked with a reduction in the coordination number of specific species. However, the two sub-lobes distinctly have the same intensity in CuII_LT which is not the case for the HT CuII species, where the high-k sub-lobe has a lower intensity. Dealing with pure-species WT-EXAFS, this observation can solely be linked to differences in the Cu–Cu interatomic distance. Indeed, considering the inherent dampening of the EXAFS contributions as the interatomic distance increases, the observed differences in the relative intensities of the low- and high-k peaks point to a longer Cu–Cu distance in the two HT CuII species compared to CuII_LT. Looking at Fig. 8, is possible to identify differences in the signal distribution of the second sub-lobe in Cu_HT species, suggesting a shorter Cu–Cu interatomic distance in CuII_HT_b with respect to CuII_HT_a. Indeed, the former species shows a distinctly higher intensity in the 2.5–3.0 Å R-space range, consistent with the slight shift towards lower R-values visible by comparing the FT-EXAFS spectra of the two species in Fig. 6b.
Focusing on the CuI species (Fig. 8b), the low-k WT-EXAFS signals associated with CuI_a and CuI_b are overall weaker and broader concerning what is observed for the CuII species. This is consistent with the FT-EXAFS results and is due to the already-mentioned structural disorder in the second-shell region and the relatively high temperature at which the CuI species form. Conversely, the power density function and WT-EXAFS map of CuI_c present a dominating peak/sub-lobe shifted towards higher k values (k = 4 Å−1) compared to the sub-lobe detected in the WT-EXAFS map of the CuII species (k = 3 Å−1). Based on the above-mentioned observations, we assign this sub-lobe to the presence of additional single and multiple scattering contributions from Cu–C/O in CuI–carbonyl adducts, reinforcing the assignment of CuI_c to CuI–(CO)x complexes (vide infra). Focusing on the high-k region for the CuI species, it is impossible to detect a well-defined sub-lobe at ca. 7 Å−1, as it was for the CuII moieties. We note an intensity increase above ca. 8 Å−1 for the CuI_b species (shadowed grey boxes in Fig. 8). However, due to poorer S/N ratio in the MCR-derived k2χ(k) curve for this species, likely also due to the high TCu(I)_bavg (430 °C), we allocate the intensity increase to an artifact that causes a spurious signal increase in the high-k portion of the WT map that cannot be safely interpreted. Accordingly, we recognize that the absence of a high-k sub-lobe in the CuI species corroborates previous studies suggesting that multimeric copper-oxo moieties are cleaved during reduction, forming isolated CuI ions that have Cu–Cu interatomic distances higher than the EXAFS-accessible R-space range.80
3.7 Confirming the formation of CuI–CO with in situ FT-IR spectroscopy measurements
To verify that the CuI_c component is indeed from CuI–(CO)x formation, and whether it is linked with mono- or dicarbonyl species, we performed in situ CO-TPR experiments coupled with FT-IR spectroscopy on a pre-oxidized 018Cu-MOR(7) sample. The results are reported in Fig. 9a. As CO atmosphere is introduced to the sample, a band at 2158 cm−1 evolves slowly, confirming the formation of some CuI species that is then available to form CuI–CO adducts on Cu-MOR zeolites.81 Additionally, we observe the presence of the gaseous phase of CO, contributing with the presence of rotational-vibrational branches on both sides of the CuI–CO band. The maximum intensity of these branches is marked with an asterisk (*). Then, as the temperature increases, the monocarbonyl band increases, until a maximum is reached at about 350 °C. The intensity of the band at 2158 cm−1 reflects both the amount of CuI (growing according to the reducing effect of CO) and the stability of the CuI–CO adducts. For this reason, it is not directly coinciding with the evolution of the CuI_c component, which is found to have a maximum at about 300 °C (Fig. 2b), however, clearly present up to at least 400 °C. The formation of a CuI–CO band on a Cu zeolite with no CuI as evidenced by XAS, suggests that at 120 °C, CO is instantaneously able to reduce some CuII species to CuI, that are then able to form the CuI–CO adduct and form CO2. This is consistent with the reduction of CuII, the early consumption of CO, and the subsequent formation of CO2, as observed above when performing the parallel CO-TPR/XAS experiment. Due to the strong presence of rotational-vibrational features, CO-TPD was also performed to verify that only monocarbonyl species form when exposed to CO and that the prolonged presence of the 2158 cm−1 band was indeed due to a strong interaction with the framework-coordinated CuI ions, and not due to the presence of gaseous CO. The results are reported in ESI, Section S7,† and show undoubtedly the CuI–CO band at 2158 cm−1, with no presence of the symmetric or asymmetric stretches of dicarbonyl species.81 The band decreases in intensity with temperature but is not completely lost until T = 300 °C. This suggests that even without a constant atmosphere of CO, the monocarbonyl has a strong interaction with the Cu sites, confirming that CuI–CO is the intermediate species observed during CO-TPR.
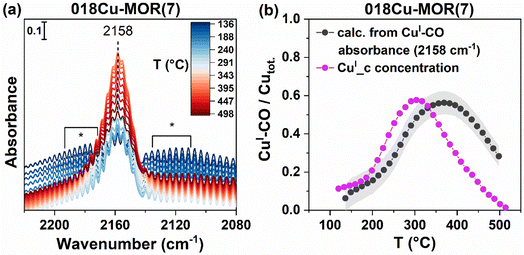 |
| Fig. 9 (a) FT-IR spectra obtained during CO-TPR. Spectra are background subtracted. (b) A comparison of the fraction of CuI–CO species found with XAS throughout the TPR protocol (CuI_c), and the corresponding CuI–monocarbonyl fraction calculated from Lambert–Beer law by integrating the band at 2158 cm−1. The error bars (grey band) are given as the standard deviation determined from three different baseline positions on the rotational–vibrational features when obtaining the integrated area. | |
To more quantitatively compare the temperature-dependent evolution of the 2158 cm−1 band from FT-IR with the evolution of the CuI_c component, we calculated the CO concentration from the integrated feature at 2158 cm−1 by applying the Lambert–Beer law given in eqn (3). Deplano et al. recently determined the molar attenuation coefficient, ε, for monocarbonyl species forming on Cu-ZSM-5 zeolites,40 and as long as the band shape of the CuI–CO band is similar, this value should be somewhat comparable for other zeolite systems. We therefore used this value for our calculations herein. By dividing the calculated CO concentration by the total Cu concentration of the system we indirectly find the fraction of CuI–CO species with FT-IR. The results are reported in Fig. 9b, and lead to a strong correspondence with the CuI_c concentration determined by XAS. Both traces give a maximum CuI–CO formation (∼300 °C) of about 55% of the total Cu concentration in 018Cu-MOR(7). At this temperature, almost 80% of the Cu in the sample has become CuI (Fig. 3), indicating that some CO species have already desorbed, leaving bare CuI sites. The CO reduction on CuII sites is a two-electron process, and should therefore only be possible on Cu-dimers, or possibly two isolated CuII species nearby.44 Overall, these results, combined with the WT-EXAFS results described above, suggest that a very large fraction of the Cu-species are forming dimeric species already after O2 activation, and do not need to be made mobile with, e.g., an NH3 molecule to form the dimers, as suggested for other zeolite systems, like CHA.43,82 We note that there is a small shift in the temperature profile for the CO/Cu trace (calculated from absorbance) compared to CuI_c; nonetheless, we allocate this to the different setups utilized, causing small variations in the measured temperature. Additionally, we explain the slower CO desorption in the FT-IR setup to the vast difference in the sample environment. FT-IR was measured on a thin wafer, while XAS was performed on a pelletized sample in a capillary reactor. The different pellet morphology and sample environment likely leads to a slower diffusion through the sample in the FT-IR cell compared to the capillary.
4 Discussion
In the previous sections we have combined advanced spectroscopy methods in different spectral ranges with qualitative MS analysis to accurately determine the chemical nature, local structure and abundance of Cu species formed in two representative Cu-MOR samples after pretreatment in O2 and subsequent TPR using CH4, C2H6 and CO as reducing agents/substrates. The two studied Cu-MOR samples were selected based on their significantly different performance in the direct MTM conversion,18,23 and the results obtained herein unlocked novel insights on the Cu active site for this iconic reaction.
In this section, we critically discuss the obtained results in view of previous literature findings, to underline how the innovative approaches implemented in this work could contribute to deepen the atomic-scale understanding of Cu-zeolite based materials towards selective C–H bond activation. To this aim, Scheme 2 summarizes the main Cu-species identified throughout our experiments, illustrating their dependency on temperature, gas feed and compositional characteristics of the samples. We will examine separately the two key steps addressed in this work, namely the CuII speciation after pretreatment in O2 and reduction dynamics/CuI speciation under TPR conditions.
 |
| Scheme 2 Summary of the identified Cu species and their evolution as a function of temperature, gas feed and Cu-MOR composition. (a) CuII speciation after pre-treatment in O2; pie charts illustrate the fractions of Cu-species determined by XANES MCR analysis at 500 °C in O2 and at 120 °C in He just before exposing to the selected reducing agent (Fig. 2b, averaged values over all the available datasets). (b) Reduction behavior and CuI speciation under TPR conditions with CH4, C2H6 and CO; reduction onsets are estimated based on the temperature values corresponding to the first maximum in the first derivative curve of the CuI tot. (T) profiles (Fig. 3, bottom panel). In all cases, pictorial models of the identified Cu species are reported; in part (a) the main assignments are displayed in the top boxes, while plausible alternatives, also consistent with our spectroscopic findings, are shown in the bottom boxes. Atoms color code: Cu, green; H, white; O, red; C, black; Si, grey; Al, yellow. | |
4.1 CuII speciation after pre-treatment in O2
Resolving the Cu speciation in zeolites after high-temperature thermal treatment in O2 represents an essential, yet challenging, step before further analysis of Cu reactivity/reducibility is undertaken. A number of possible species has been proposed in literature, depending on zeolite topology, compositional characteristics, and synthesis/pre-treatment conditions.19,30,83 The experimental findings obtained here, including for the first time MCR-ALS-based extraction of pure EXAFS spectra and their visual interpretation by WT, points to mono-μ-oxo dicopper(II) cores as dominant configurations at 500 °C in O2. Consistently with previous HERFD-XANES analysis of the same samples,18 two closely related components are found at 500 °C, namely CuII_HT_a and CuII_HT_b, with sample-dependent relative abundance (see pie charts in Scheme 2a) and shorter Cu–Cu interatomic distance in the latter component. According to recent literature,63,64,84 different Cu–O–Cu angles (and thus Cu–Cu internuclear separation) in mono-μ-oxo dicopper species can influence the selectivity and reactivity of dicopper sites for MTM. In particular, according to Artsiusheuski et al.,84 dicopper cores featuring a smaller Cu–O–Cu angle and a shorter Cu–Cu distance are characterized by a higher selectivity towards methanol and promoted at low Cu loading. This is fully consistent with both the MCR-ALS analysis and testing results reported previously for 018Cu-MOR(7) and novel structural insights obtained here, where WT-EXAFS indicate a shorter Cu–Cu distance in CuII_HT_b, which is the more abundant species in 018Cu-MOR(7). In parallel, the clear Cu–Cu scattering signal in WT-EXAFS for both HT CuII species, allows ruling out trans-μ-1,2-peroxo-dicopper(II) species, which would imply an excessively long internuclear separation (typically > 4 Å (ref. 85)), not consistent with the observed signal. In contrast, “coupled” [CuOH]+ monomers remain a plausible alternative for CuII_HT_a. The coupled monomers, also recently considered among the viable MTM active sites,86 would conserve the same tridentate coordination motif as mono-μ-oxo dicopper and, assuming Cu–Cu distances up to ca. 3.3 Å range, would fit with the Cu–Cu scattering observed in WT-EXAFS.
Importantly, we observe changes in CuII speciation while cooling down from 500 to 120 °C, under O2/He flow, mostly connected with an increase in the concentration of CuII_LT, preferentially forming at the expense of CuII_HT_a. A similar behavior was already reported for both Cu-CHA and Cu-MOR zeolites,46 but was not quantitatively addressed by MCR analysis. Both the XANES and the EXAFS features of CuII_LT points to a four-fold coordinated, multimeric CuII species. The species increase in abundance also when the feed is switched from O2 to He in the final cooling step before TPR. A plausible assignment starting from a mono-μ-oxo dicopper(II) core could entail bis-μ-hydroxo dicopper(II) formed upon interaction with residual H2O traces, favored as temperature decreases. This hydroxo-bridged dicopper(II) species is specifically discussed in enzymatic systems and complex formation,87,88 but a similar hydration event forming trimeric Cu species in zeolites has also been suggested.89 In a recent theoretical study on Cu-CHA,90 Göltl et al. indicated such hydroxylated dicopper(II) species as the most stable around the typical CH4 exposure conditions, and plausibly active towards MTM. Alternatively, μ-η2,η2-peroxo-dicopper(II) species could be proposed as a way for the system to prevent self-reduction by using O2 molecules supplied in the first part of the cooling step. A resonance structure between the latter species and a bis-(μ-oxo) dicopper cluster has been recently proposed to describe MTM-active cores in Cu-FER.91
4.2 Reactivity and CuI speciation under TPR conditions
MCR-ALS analysis of XAS data obtained under consistent TPR conditions revealed a common linear CuI_a species for all the three reducing agents employed. Its spectroscopic signature is fully consistent with bare CuI ions coordinated to the MOR framework.34,70 Overall, the XAS results obtained under CH4-TPR well align with the recent report by Artsiusheuski et al.,92 confirming for Cu-MOR the absence of either metallic or oxidic aggregates at the end of the TPR step. Nonetheless, during both C2H6- and CO-TPR, additional CuI species were found. CuI_b, observed at T > 300 °C during C2H6-TPR is again a quasi-linear CuI, likely with a slightly different geometry/siting with respect to CuI_a. Considering the recent findings about ethane-to-ethylene conversion over Cu-MOR,93 both the reactant and the formed hydrocarbons could trigger long-range interactions leading to certain distortion in the CuI centers local coordination, especially in the high-temperature portion of the TPR. The CuI_c species found during CO-TPR was established herein as a chemisorbed CuI–CO intermediate by the combination of results from XAS and FT-IR. We were also able to verify the number of CuI–CO species formed throughout the TPR protocol by quantifying the absorbance observed with FT-IR. By exploiting CO oxidation as a probe reaction to titrate dimeric Cu species in zeolites,42,43 CO-TPR results also showed, in agreement with WT-EXAFS, that dicopper(II) species are largely dominant in both Cu-MOR samples before reductant exposure.
By coupling the transformation of CuII to CuI, observed with XAS and UV-vis spectroscopy, with the evolvement of the CO2 and CH4 MS fragments, we found an unambiguous connection between the three traces. Additionally, a small but consistent difference was observed between the two different MOR compositions. The 018Cu-MOR(7) sample, with the highest methane activity in MTM18 as well as the highest abundance of CuII_HT_b after pre-treatment, showed a faster reduction at low temperatures compared to 036Cu-MOR(11) (see Scheme 2b). This observation offers an additional piece of evidence in connecting the CuII_HT_b, described as a mono-μ-oxo dicopper(II) core with Cu–Cu internuclear separation around 3 Å, with the MTM active site in the investigated Cu-MOR samples.
Notably, in situ UV-vis during CH4-TPR evidenced the evolution of a complex “multiplet” band in the d–d region of 036Cu-MOR(11). This band, previously observed in Cu-CHA75,76 and reported here for the first time in Cu-MOR, was loosely assigned to a high-temperature, three-fold coordinated CuII species, much more abundant in 036Cu-MOR(11) and thus likely related to the CuII_HT_a component.
5 Conclusions
In this work, detailed insight into the dynamic changes of the Cu-speciation during light alkane selective oxidation has been obtained. We collected in situ XAS spectra on two compositionally different Cu-MOR zeolites during temperature programmed reduction experiments with CH4, C2H6, and CO. Upon coupling the relevant MS traces with MCR-ALS analysis of the XANES data, we reveal clear behavioral differences among the reducing agents and trends related both to local structure and Cu reducibility. The relevant experimental conditions allowed us to connect reducibility to the MTM activity for mordenites, which has previously been dubious due to “self-reduction” resistant Cu species in the highly active sample, 018Cu-MOR(7). Additional in situ UV-vis and FT-IR spectroscopy experiments allowed for confirmative and additional information related to the Cu-speciation. We also applied a leading-edge method using the MCR-ALS derived spectra to extract EXAFS signals for all pure Cu species. Wavelet transform (WT) analysis on the pure EXAFS spectra provided confirmative information on Cu–Cu single scattering contributions in the 3.0–3.5 Å−1 interatomic distance range for all three CuII species, which confirmed the hypothesis of these species being related to dimeric copper species. This study has pushed the boundaries for how MCR-ALS analysis and EXAFS-WT mapping can be used. We anticipate that the experimental procedure and data analysis strategy presented herein, especially when combined with complementary techniques like UV-vis and FT-IR spectroscopy, will have great impact on our understanding of other systems. In particular, we anticipate that such research will be very important in the advancing our understanding of other transition metal loaded zeolites in selective oxidation, and also to shed further light on the reactivity of substrates other than methane. Finally, the approach can further be transferred to many other reactions where the complex superimposition of various phenomena has led to inconclusive results thus far.
Data availability
ESI† is available in the additional files and further supporting data is available from the authors upon request.
Author contributions
K. K.: conceptualization, investigation, formal analysis, writing – original draft, visualization. B. G.: investigation, formal analysis, writing – original draft, visualization. M. S., B. G. S., G. D., S. P.: investigation, writing – review & editing. U. O., P. B., S. B.: supervision, validation, writing – review & editing. S. S.: conceptualization, supervision, funding acquisition, writing – review & editing. E. B.: formal analysis, methodology, writing – original draft, supervision.
Conflicts of interest
There are no conflicts to declare.
Acknowledgements
The authors acknowledge the iCSI (industrial Catalysis Science and Innovation) Centre for Research-based Innovation, which receives financial support from the Research Council of Norway under contract no. 237922. The authors also acknowledge funding from Horizon 2020 Excellence Science ERC-Synergy program 2019-CUBE: “Unravelling the secrets of Cu-based catalysts for C–H activation” (grant agreement no. 856446). B. G., G. D., M. S., S. B. and E. B. acknowledge support from the Project CH4.0 under the MUR program “Dipartimenti di Eccellenza 2023-2027” (CUP: D13C22003520001). We acknowledge the European Synchrotron Radiation Facility (ESRF) for the provision of synchrotron radiation facilities, and we would like to thank W. van Beek, D. Stoian, and K. Marshall for assistance and support in using beamline BM31. We also would like to acknowledge MAX IV Laboratory for time on Beamline Balder under Proposal 20190347, which laid the foundation for developing the experiment performed at BM31. Research conducted at MAX IV, a Swedish national user facility, is supported by the Swedish Research Council under contract 2018–07152, the Swedish Governmental Agency for Innovation Systems under contract 2018-04969, and Formas under contract 2019-02496. We thank the staff, J. Just, M. Ciambezi, and K. Klementiev for help and guidance at the beamline. We are grateful to A. Martini for programming and making available the script employed for WT-EXAFS analysis and to D. K. Pappas for the initial conceptualization of the TPR-XAS study as well as contribution to our background knowledge on the investigated systems.
References
- J. H. Lunsford, Catalytic conversion of methane to more useful chemicals and fuels: a challenge for the 21st century, Catal. Today, 2000, 63, 165–174 CrossRef CAS.
- R. Horn and R. Schlögl, Methane Activation by Heterogeneous Catalysis, Catal. Lett., 2015, 145, 23–39 CrossRef CAS.
- M. Ravi, M. Ranocchiari and J. A. van Bokhoven, The Direct Catalytic Oxidation of Methane to Methanol-A Critical Assessment, Angew. Chem., Int. Ed., 2017, 56, 16464–16483 CrossRef CAS PubMed.
- M. H. Groothaert, P. J. Smeets, B. F. Sels, P. A. Jacobs and R. A. Schoonheydt, Selective oxidation of methane by the bis(μ-oxo)dicopper core stabilized on ZSM-5 and mordenite zeolites, J. Am. Chem. Soc., 2005, 127, 1394–1395 CrossRef CAS PubMed.
- A. A. Latimer, A. Kakekhani, A. R. Kulkarni and J. K. Nørskov, Direct Methane to Methanol: The Selectivity–Conversion Limit and Design Strategies, ACS Catal., 2018, 8, 6894–6907 CrossRef CAS.
- V. L. Sushkevich, D. Palagin, M. Ranocchiari and J. A. van Bokhoven, Selective Anaerobic Oxidation of Methane Enables Direct Synthesis of Methanol, Science, 2017, 356, 523–527 CrossRef CAS PubMed.
- E. M. Alayon, M. Nachtegaal, M. Ranocchiari and J. A. van Bokhoven, Catalytic conversion of methane to methanol over Cu-mordenite, Chem. Commun., 2012, 48, 404–406 RSC.
- S. Grundner, W. Luo, M. Sanchez-Sanchez and J. A. Lercher, Synthesis of Single-Site Copper Catalysts for Methane Partial Oxidation, Chem. Commun., 2016, 52, 2553–2556 RSC.
- P. Tomkins, A. Mansouri, S. E. Bozbag, F. Krumeich, M. B. Park, E. M. Alayon, M. Ranocchiari and J. A. van Bokhoven, Isothermal Cyclic Conversion of Methane into Methanol over Copper-Exchanged Zeolite at Low Temperature, Angew. Chem., Int. Ed., 2016, 55, 5467–5471 CrossRef CAS PubMed.
- S. Prodinger, K. Kvande, B. Arstad, E. Borfecchia, P. Beato and S. Svelle, Synthesis–Structure–Activity Relationship in Cu-MOR for Partial Methane Oxidation: Al Siting via Inorganic Structure-Directing Agents, ACS Catal., 2022, 12, 2166–2177 CrossRef CAS.
- S. Grundner, M. A. C. Markovits, G. Li, M. Tromp, E. A. Pidko, E. J. M. Hensen, A. Jentys, M. Sanchez-Sanchez and J. A. Lercher, Single-site trinuclear copper oxygen clusters in mordenite for selective conversion of methane to methanol, Nat. Commun., 2015, 6, 7546 CrossRef PubMed.
- D. K. Pappas, E. Borfecchia, M. Dyballa, I. A. Pankin, K. A. Lomachenko, A. Martini, M. Signorile, S. Teketel, B. Arstad, G. Berlier, C. Lamberti, S. Bordiga, U. Olsbye, K. P. Lillerud, S. Svelle and P. Beato, Methane to Methanol: Structure-Activity Relationships for Cu-CHA, J. Am. Chem. Soc., 2017, 139, 14961–14975 CrossRef CAS PubMed.
- M. J. Wulfers, S. Teketel, B. Ipek and R. F. Lobo, Conversion of Methane to Methanol on Copper-Containing Small-Pore Zeolites and Zeotypes, Chem. Commun., 2015, 51, 4447–4450 RSC.
- D. K. Pappas, E. Borfecchia, M. Dyballa, K. A. Lomachenko, A. Martini, G. Berlier, B. Arstad, C. Lamberti, S. Bordiga, U. Olsbye, S. Svelle and P. Beato, Understanding and Optimizing the Performance of Cu-FER for The Direct CH4 to CH3OH Conversion, ChemCatChem, 2019, 11, 621–627 CrossRef CAS.
- V. L. Sushkevich and J. A. van Bokhoven, Methane-to-Methanol: Activity Descriptors in Copper-Exchanged Zeolites for the Rational Design of Materials, ACS Catal., 2019, 9, 6293–6304 CrossRef CAS.
- A. J. Knorpp, A. B. Pinar, M. A. Newton, V. L. Sushkevich and J. A. van Bokhoven, Copper-Exchanged Omega (MAZ) Zeolite: Copper-Concentration Dependent Active Sites and its Unprecedented Methane to Methanol Conversion, ChemCatChem, 2018, 10, 5593–5596 CrossRef CAS.
- K. Kvande, S. Prodinger, F. Schlimpen, P. Beato, P. Pale, S. Chassaing and S. Svelle, Copper-zeolites Prepared by Solid-state Ion Exchange - Characterization and Evaluation for the Direct Conversion of Methane to Methanol, Top. Catal., 2022 DOI:10.1007/s11244-022-01763-7.
- D. K. Pappas, A. Martini, M. Dyballa, K. Kvande, S. Teketel, K. A. Lomachenko, R. Baran, P. Glatzel, B. Arstad, G. Berlier, C. Lamberti, S. Bordiga, U. Olsbye, S. Svelle, P. Beato and E. Borfecchia, The Nuclearity of the Active Site for Methane to Methanol Conversion in Cu-Mordenite: A Quantitative Assessment, J. Am. Chem. Soc., 2018, 140, 15270–15278 CrossRef CAS PubMed.
- K. Kvande, D. K. Pappas, E. Borfecchia and K. A. Lomachenko, Advanced X-ray Absorption Spectroscopy Analysis to Determine Structure-Activity Relationships for Cu-Zeolites in the Direct Conversion of Methane to Methanol, ChemCatChem, 2020, 12, 2385–2405 CrossRef CAS.
- V. L. Sushkevich, M. Artsiusheuski, D. Klose, G. Jeschke and J. A. van Bokhoven, Identification of Kinetic and Spectroscopic Signatures of Copper Sites for Direct Oxidation of Methane to Methanol, Angew. Chem., Int. Ed., 2021, 60, 15944–15953 CrossRef CAS PubMed.
- M. A. Newton, A. J. Knorpp, A. B. Pinar, V. L. Sushkevich, D. Palagin and J. A. van Bokhoven, On the Mechanism Underlying the Direct Conversion of Methane to Methanol by Copper Hosted in Zeolites; Braiding Cu K-Edge XANES and Reactivity Studies, J. Am. Chem. Soc., 2018, 140, 10090–10093 CrossRef CAS PubMed.
- J. Zheng, I. Lee, E. Khramenkova, M. Wang, B. Peng, O. Y. Gutierrez, J. L. Fulton, D. M. Camaioni, R. Khare, A. Jentys, G. L. Haller, E. A. Pidko, M. Sanchez-Sanchez and J. A. Lercher, Importance of Methane Chemical Potential for Its Conversion to Methanol on Cu-Exchanged Mordenite, Chem.–Eur. J., 2020, 26, 7563–7567 CrossRef CAS PubMed.
- K. A. Lomachenko, A. Martini, D. K. Pappas, C. Negri, M. Dyballa, G. Berlier, S. Bordiga, C. Lamberti, U. Olsbye, S. Svelle, P. Beato and E. Borfecchia, The impact of reaction conditions and material composition on the stepwise methane to methanol conversion over Cu-MOR: an operando XAS study, Catal. Today, 2019, 336, 99–108 CrossRef CAS.
- D. Plessers, A. J. Heyer, H. M. Rhoda, M. L. Bols, E. I. Solomon, R. A. Schoonheydt and B. F. Sels, Tuning Copper Active Site Composition in Cu-MOR through Co-Cation Modification for Methane Activation, ACS Catal., 2023, 13, 1906–1915 CrossRef CAS PubMed.
- M. Dyballa, K. Thorshaug, D. K. Pappas, E. Borfecchia, K. Kvande, S. Bordiga, G. Berlier, A. Lazzarini, U. Olsbye, P. Beato, S. Svelle and B. Arstad, Zeolite Surface Methoxy Groups as Key Intermediates in the Stepwise Conversion of Methane to Methanol, ChemCatChem, 2019, 11, 5022–5026 CrossRef CAS.
- V. L. Sushkevich, J. A. van Bokhoven and R. Verel, Pathways of Methane Transformation over Copper-Exchanged Mordenite as Revealed by In Situ NMR and IR Spectroscopy, Angew. Chem., Int. Ed., 2020, 59, 910–918 CrossRef CAS PubMed.
- L. Sun, Y. Wang, C. Wang, Z. Xie, N. Guan and L. Li, Water-involved methane-selective catalytic oxidation by dioxygen over copper zeolites, Chem, 2021, 7, 1557–1568 CAS.
- A. Koishybay and D. F. Shantz, Water Is the Oxygen Source for Methanol Produced in Partial Oxidation of Methane in a Flow Reactor over Cu-SSZ-13, J. Am. Chem. Soc., 2020, 142, 11962–11966 CrossRef CAS PubMed.
- X. Liu, T. Liang, R. Barbosa, G. Chen, H. Toghiani and Y. Xiang, Ammoxidation of Ethane to Acetonitrile and Ethylene: Reaction Transient Analysis for the Co/HZSM-5 Catalyst, ACS Omega, 2020, 5, 1669–1678 CrossRef CAS PubMed.
- M. A. Newton, A. J. Knorpp, V. L. Sushkevich, D. Palagin and J. A. van Bokhoven, Active sites and mechanisms in the direct conversion of methane to methanol using Cu in zeolitic hosts: a critical examination, Chem. Soc. Rev., 2020, 49, 1449–1486 RSC.
- K. Kvande, D. K. Pappas, M. Dyballa, C. Buono, M. Signorile, E. Borfecchia, K. A. Lomachenko, B. Arstad, S. Bordiga, G. Berlier, U. Olsbye, P. Beato and S. Svelle, Comparing the Nature of Active Sites in Cu-loaded SAPO-34 and SSZ-13 for the Direct Conversion of Methane to Methanol, Catalysts, 2020, 10, 191 CrossRef CAS.
- D. K. Pappas, K. Kvande, M. Kalyva, M. Dyballa, K. A. Lomachenko, B. Arstad, E. Borfecchia, S. Bordiga, U. Olsbye, P. Beato and S. Svelle, Influence of Cu-speciation in mordenite on direct methane to methanol conversion: Multi-Technique characterization and comparison with NH3 selective catalytic reduction of NOx, Catal. Today, 2021, 369, 105–111 CrossRef CAS.
- M. Dyballa, D. K. Pappas, K. Kvande, E. Borfecchia, B. Arstad, P. Beato, U. Olsbye and S. Svelle, On How Copper Mordenite Properties Govern the Framework Stability and Activity in the Methane-to-Methanol Conversion, ACS Catal., 2019, 9, 365–375 CrossRef CAS.
- A. Martini, M. Signorile, C. Negri, K. Kvande, K. A. Lomachenko, S. Svelle, P. Beato, G. Berlier, E. Borfecchia and S. Bordiga, EXAFS wavelet transform analysis of Cu-MOR zeolites for the direct methane to methanol conversion, Phys. Chem. Chem. Phys., 2020, 22, 18950–18963 RSC.
- A. Martini, E. Borfecchia, K. A. Lomachenko, I. A. Pankin, C. Negri, G. Berlier, P. Beato, H. Falsig, S. Bordiga and C. Lamberti, Composition-Driven Cu-Speciation and Reducibility in Cu-CHA Zeolite Catalysts: a Multivariate XAS/FTIR Approach to Complexity, Chem. Sci., 2017, 8, 6836–6851 RSC.
- A. Martini, E. Alladio and E. Borfecchia, Determining Cu–Speciation in the Cu–CHA Zeolite Catalyst: The Potential of Multivariate Curve Resolution Analysis of In Situ XAS Data, Top. Catal., 2018, 61, 1396–1407 CrossRef CAS.
- J. S. Valente, R. Quintana-Solórzano, H. Armendáriz-Herrera and J.-M. M. Millet, Decarbonizing Petrochemical Processes: Contribution and Perspectives of the Selective Oxidation of C1–C3 Paraffins, ACS Catal., 2023, 13, 1693–1716 CrossRef CAS.
- K. I. Hadjiivanov, M. M. Kantcheva and D. G. Klissurski, IR study of CO adsorption on Cu-ZSM-5 and CuO/SiO2 catalysts: σ and π components of the Cu+—CO bond, J. Chem. Soc., Faraday Trans., 1996, 92, 4595–4600 RSC.
- V. M. Rakić, R. V. Hercigonja and V. T. Dondur, CO interaction with zeolites studied by TPD and FTIR: transition-metal ion-exchanged FAU-type zeolites, Microporous Mesoporous Mater., 1999, 27, 27–39 CrossRef.
- G. Deplano, M. Signorile, V. Crocellà, N. G. Porcaro, C. Atzori, B. G. Solemsli, S. Svelle and S. Bordiga, Titration of Cu(I) Sites in Cu-ZSM-5 by Volumetric CO Adsorption, ACS Appl. Mater. Interfaces, 2022, 14, 21059–21068 CrossRef CAS PubMed.
- R. Bulánek, B. Wichterlová, Z. Sobalík and J. Tichý, Reducibility and oxidation activity of Cu ions in zeolites - Effect of Cu ion coordination and zeolite framework composition, Appl. Catal. B Environ., 2001, 31, 13–25 CrossRef.
- H. Li, C. Paolucci, I. Khurana, L. N. Wilcox, F. Göltl, J. D. Albarracin-Caballero, A. J. Shih, F. H. Ribeiro, R. Gounder and W. F. Schneider, Consequences of exchange-site heterogeneity and dynamics on the UV-visible spectrum of Cu-exchanged SSZ-13, Chem. Sci., 2019, 10, 2373–2384 RSC.
- R. Villamaina, U. Iacobone, I. Nova, M. P. Ruggeri, J. Collier, D. Thompsett and E. Tronconi, Low-T CO Oxidation over Cu−CHA Catalysts in Presence of NH3: Probing the Mobility of CuII Ions and the Role of Multinuclear CuII Species, ChemCatChem, 2020, 12, 3843–3848 CrossRef CAS.
- P. Da Costa, B. Modén, G. D. Meitzner, D. K. Lee and E. Iglesia, Spectroscopic and chemical characterization of active and inactive Cu species in NO decomposition catalysts based on Cu-ZSM5, Phys. Chem. Chem. Phys., 2002, 4, 4590–4601 RSC.
- W. H. Cassinelli, L. Martins, A. R. Passos, S. H. Pulcinelli, C. V. Santilli, A. Rochet and V. Briois, Multivariate curve resolution analysis applied to time-resolved synchrotron X-ray Absorption Spectroscopy monitoring of the activation of copper alumina catalyst, Catal. Today, 2014, 229, 114–122 CrossRef CAS.
- E. Borfecchia, D. K. Pappas, M. Dyballa, K. A. Lomachenko, C. Negri, M. Signorile and G. Berlier, Evolution of Active Sites During Selective Oxidation of Methane to Methanol over Cu-CHA and Cu-MOR Zeolites as Monitored by Operando XAS, Catal. Today, 2019, 333, 17–27 CrossRef CAS.
- P. M. Abdala, O. V. Safonova, G. Wiker, W. van Beek, H. Emerich, J. A. van Bokhoven, J. Sa, J. Szlachetko and M. Nachtegaal, Scientific Opportunities for Heterogeneous Catalysis Research at the SuperXAS and SNBL Beam Lines, Chimia, 2012, 66, 699–705 CrossRef CAS PubMed.
- W. van Beek, O. V. Safonova, G. Wiker and H. Emerich, SNBL, a dedicated beamline for combined in situ X-ray diffraction, X-ray absorption and Raman scattering experiments, Phase Transitions, 2011, 84, 726–732 CrossRef CAS.
- B. Ravel and M. Newville, ATHENA, ARTEMIS, HEPHAESTUS: data analysis for X-ray absorption spectroscopy using IFEFFIT, J. Synchrotron Radiat., 2005, 12, 537–541 CrossRef CAS PubMed.
- J. E. Penner-Hahn, X-ray absorption spectroscopy in coordination chemistry, Coord. Chem. Rev., 1999, 190, 1101–1123 CrossRef.
- S. Bordiga, E. Groppo, G. Agostini, J. A. van Bokhoven and C. Lamberti, Reactivity of Surface Species in Heterogeneous Catalysts Probed by In Situ X-ray Absorption Techniques, Chem. Rev., 2013, 113, 1736–1850 CrossRef CAS PubMed.
-
C. Ruckebusch, Resolving spectral mixtures: with applications from ultrafast time-resolved spectroscopy to super-resolution imaging, Elsevier, 2016 Search PubMed.
- C. Ruckebusch and L. Blanchet, Multivariate curve resolution: a review of advanced and tailored applications and challenges, Anal. Chim. Acta, 2013, 765, 28–36 CrossRef CAS PubMed.
- A. de Juan and R. Tauler, Chemometrics applied to unravel multicomponent processes and mixtures: revisiting latest trends in multivariate resolution, Anal. Chim. Acta, 2003, 500, 195–210 CrossRef CAS.
- W. Windig and J. Guilment, Interactive self-modeling mixture analysis, Anal. Chem., 1991, 63, 1425–1432 CrossRef CAS.
- J. Jaumot, R. Gargallo, A. De Juan and R. Tauler, A graphical user-friendly interface for MCR-ALS: a new tool for multivariate curve resolution in MATLAB, Chemom. Intell. Lab. Syst., 2005, 76, 101–110 CrossRef CAS.
- H. Funke, A. C. Scheinost and M. Chukalina, Wavelet Analysis of Extended X-Ray Absorption Fine Structure Data, Phys. Rev. B: Condens. Matter Mater. Phys., 2005, 71, 094110 CrossRef.
- M. Muñoz, P. Argoul and F. Farges, Continuous Cauchy wavelet transform analyses of EXAFS spectra: a qualitative approach, Am. Mineral., 2003, 88, 694–700 CrossRef.
- J. Timoshenko and A. Kuzmin, Wavelet Data Analysis of EXAFS Spectra, Comput. Phys. Commun., 2009, 180, 920–925 CrossRef CAS.
- I. A. Pankin, A. Martini, K. A. Lomachenko, A. V. Soldatov, S. Bordiga and E. Borfecchia, Identifying Cu-Oxo Species In Cu-Zeolites By XAS: A Theoretical Survey by DFT-Assisted XANES Simulation and EXAFS Wavelet Transform, Catal. Today, 2020, 345, 125–135 CrossRef CAS.
- V. L. Sushkevich, O. V. Safonova, D. Palagin, M. A. Newton and J. A. van Bokhoven, Structure of Copper Sites in Zeolites Examined by Fourier and Wavelet Transform Analysis of EXAFS, Chem. Sci., 2020, 11, 5299–5312 RSC.
- T. J. Penfold, I. Tavernelli, C. J. Milne, M. Reinhard, A. El Nahhas, R. Abela, U. Rothlisberger and M. Chergui, A wavelet analysis for the X-ray absorption spectra of molecules, J. Chem. Phys., 2013, 138, 014104 CrossRef CAS PubMed.
- B. E. R. Snyder, P. Vanelderen, R. A. Schoonheydt, B. F. Sels and E. I. Solomon, Second-Sphere Effects on Methane Hydroxylation in Cu-Zeolites, J. Am. Chem. Soc., 2018, 140, 9236–9243 CrossRef CAS PubMed.
- P. Vanelderen, B. E. R. Snyder, M.-L. Tsai, R. G. Hadt, J. Vancauwenbergh, O. Coussens, R. A. Schoonheydt, B. F. Sels and E. I. Solomon, Spectroscopic definition of the copper active sites in mordenite: selective methane oxidation, J. Am. Chem. Soc., 2015, 137, 6383–6392 CrossRef CAS PubMed.
- H. Funke, M. Chukalina and A. C. Scheinost, A new FEFF-based wavelet for EXAFS data analysis, J. Synchrotron Radiat., 2007, 14, 426–432 CrossRef CAS PubMed.
- I. A. Pankin, H. I. Hamoud, K. A. Lomachenko, S. B. Rasmussen, A. Martini, P. Bazin, V. Valtchev, M. Daturi, C. Lamberti and S. Bordiga, Cu-and Fe-speciation in a composite zeolite catalyst for selective catalytic reduction of NOx: insights from operando XAS, Catal. Sci. Technol., 2021, 11, 846–860 RSC.
- G. Brezicki, J. D. Kammert, T. B. Gunnoe, C. Paolucci and R. J. Davis, Insights into the Speciation of Cu in the Cu-H-Mordenite Catalyst for the Oxidation of Methane to Methanol, ACS Catal., 2019, 9, 5308–5319 CrossRef CAS.
- A. Voronov, A. Urakawa, W. van Beek, N. E. Tsakoumis, H. Emerich and M. Ronning, Multivariate curve resolution applied to in situ X-ray absorption spectroscopy data: an efficient tool for data processing and analysis, Anal. Chim. Acta, 2014, 840, 20–27 CrossRef CAS PubMed.
- A. Martini and E. Borfecchia, Spectral Decomposition of X-ray Absorption Spectroscopy Datasets: Methods and Applications, Crystals, 2020, 10, 664 CrossRef CAS.
- C. Buono, A. Martini, I. A. Pankin, D. K. Pappas, C. Negri, K. Kvande, K. A. Lomachenko and E. Borfecchia, Local structure of Cu(I) ions in the MOR zeolite: A DFT-assisted XAS study, Radiat. Phys. Chem., 2020, 175, 108111 CrossRef CAS.
- B. Ruscic, Active Thermochemical Tables: Sequential Bond Dissociation Enthalpies of Methane, Ethane, and Methanol and the Related Thermochemistry, J. Phys. Chem. A, 2015, 119, 7810–7837 CrossRef CAS PubMed.
- P. J. Smeets, R. G. Hadt, J. S. Woertink, P. Vanelderen, R. A. Schoonheydt, B. F. Sels and E. I. Solomon, Oxygen Precursor to the Reactive Intermediate in Methanol Synthesis by Cu-ZSM-5, J. Am. Chem. Soc., 2010, 132, 14736–14738 CrossRef CAS PubMed.
- J. Hunt, A. Ferrari, A. Lita, M. Crosswhite, B. Ashley and A. E. Stiegman, Microwave-Specific Enhancement of the Carbon–Carbon Dioxide (Boudouard) Reaction, J. Phys. Chem. C, 2013, 117, 26871–26880 CrossRef CAS.
- P. J. Smeets, M. H. Groothaert and R. A. Schoonheydt, Cu Based Zeolites: A UV-Vis Study of the Active Site in the Selective Methane Oxidation at Low Temperatures, Catal. Today, 2005, 110, 303–309 CrossRef CAS.
- F. Giordanino, P. N. R. Vennestrom, L. F. Lundegaard, F. N. Stappen, S. Mossin, P. Beato, S. Bordiga and C. Lamberti, Characterization of Cu-Exchanged SSZ-13: a Comparative FTIR, UV-Vis, and EPR Study with Cu-ZSM-5 and Cu-beta with Similar Si/Al and Cu/Al Ratios, Dalton Trans., 2013, 42, 12741–12761 RSC.
- C. Negri, T. Selleri, E. Borfecchia, A. Martini, K. A. Lomachenko, T. V. W. Janssens, M. Cutini, S. Bordiga and G. Berlier, Structure and Reactivity of Oxygen-Bridged Diamino Dicopper(II) Complexes in Cu-Ion-Exchanged Chabazite Catalyst for NH3-Mediated Selective Catalytic Reduction, J. Am. Chem. Soc., 2020, 142, 15884–15896 CrossRef CAS PubMed.
- G. T. Palomino, P. Fisicaro, S. Bordiga, A. Zecchina, E. Giamello and C. Lamberti, Oxidation states of copper ions in ZSM-5 zeolites. A multitechnique investigation, J. Phys. Chem. B, 2000, 104, 4064–4073 CrossRef CAS PubMed.
- G. Brezicki, J. Zheng, C. Paolucci, R. Schlögl and R. J. Davis, Effect of the Co-cation on Cu Speciation in Cu-Exchanged Mordenite and ZSM-5 Catalysts for the Oxidation of Methane to Methanol, ACS Catal., 2021, 11, 4973–4987 CrossRef CAS.
- C. Lamberti, S. Bordiga, M. Salvalaggio, G. Spoto, A. Zecchina, F. Geobaldo, G. Vlaic and M. Bellatreccia, XAFS, IR, and UV-Vis Study of the CuI Environment in CuI-ZSM-5, J. Phys. Chem. B, 1997, 101, 344–360 CrossRef CAS.
- G. Deplano, A. Martini, M. Signorile, E. Borfecchia, V. Crocellà, S. Svelle and S. Bordiga, Copper Pairing in the Mordenite Framework as a Function of the CuI/CuII Speciation, Angew. Chem., Int. Ed., 2021, 60, 25891–25896 CrossRef CAS PubMed.
- S. Bordiga, C. Lamberti, F. Bonino, A. Travert and F. Thibault-Starzyk, Probing zeolites by vibrational spectroscopies, Chem. Soc. Rev., 2015, 44, 7262–7341 RSC.
- U. Iacobone, I. Nova, E. Tronconi, R. Villamaina, M. P. Ruggeri, J. Collier and D. Thompsett, Appraising Multinuclear Cu2+ Structure Formation in Cu-CHA SCR Catalysts via Low-T Dry CO Oxidation with Modulated NH3 Solvation, ChemistryOpen, 2022, 11, e202200186 CrossRef CAS PubMed.
- E. Borfecchia, P. Beato, S. Svelle, U. Olsbye, C. Lamberti and S. Bordiga, Cu-CHA – a model system for applied selective redox catalysis, Chem. Soc. Rev., 2018, 47, 8097–8133 RSC.
- M. A. Artsiusheuski, J. A. van Bokhoven and V. L. Sushkevich, Structure of Selective and Nonselective Dicopper (II) Sites in CuMFI for Methane Oxidation to Methanol, ACS Catal., 2022, 12, 15626–15637 CrossRef CAS.
- L. Vilella and F. Studt, The Stability of Copper Oxo Species in Zeolite Frameworks, Eur. J. Inorg. Chem., 2016, 2016, 1514–1520 CrossRef CAS.
- V. L. Sushkevich, D. Palagin and J. A. van Bokhoven, The Effect of the Active-Site Structure on the Activity of Copper Mordenite in the Aerobic and Anaerobic Conversion of Methane into Methanol, Angew. Chem., Int. Ed., 2018, 57, 8906–8910 CrossRef CAS PubMed.
- R. Haase, T. Beschnitt, U. Flörke and S. Herres-Pawlis, Bidentate guanidine ligands with ethylene spacer in copper-dioxygen chemistry: Structural characterization of bis(μ-hydroxo) dicopper complexes, Inorg. Chim. Acta, 2011, 374, 546–557 CrossRef CAS.
- M. R. Halvagar, P. V. Solntsev, H. Lim, B. Hedman, K. O. Hodgson, E. I. Solomon, C. J. Cramer and W. B. Tolman, Hydroxo-Bridged Dicopper(II,III) and -(III,III) Complexes: Models for Putative Intermediates in Oxidation Catalysis, J. Am. Chem. Soc., 2014, 136, 7269–7272 CrossRef CAS PubMed.
- G. Li, P. Vassilev, M. Sanchez-Sanchez, J. A. Lercher, E. J. M. Hensen and E. A. Pidko, Stability and reactivity of copper oxo-clusters in ZSM-5 zeolite for selective methane oxidation to methanol, J. Catal., 2016, 338, 305–312 CrossRef CAS.
- F. Göltl, S. Bhandari and M. Mavrikakis, Thermodynamics Perspective on the Stepwise Conversion of Methane to Methanol over Cu-Exchanged SSZ-13, ACS Catal., 2021, 11, 7719–7734 CrossRef.
- L. Tao, I. Lee, R. Khare, A. Jentys, J. L. Fulton, M. Sanchez-Sanchez and J. A. Lercher, Speciation of Cu-Oxo Clusters in Ferrierite for Selective Oxidation of Methane to Methanol, Chem. Mater., 2022, 34, 4355–4363 CrossRef CAS.
- M. A. Artsiusheuski, O. Safonova, D. Palagin, J. A. van Bokhoven and V. L. Sushkevich, Structural Evolution of Copper-Oxo Sites in Zeolites upon the Reaction with Methane Investigated by Means of Cu K-edge X-ray Absorption Spectroscopy, J. Phys. Chem. C, 2023, 127, 9603–9615 CrossRef CAS.
- K. Kvande, S. Prodinger, B. G. Solemsli, S. Bordiga, E. Borfecchia, U. Olsbye, P. Beato and S. Svelle, Cu-loaded zeolites enable the selective activation of ethane to ethylene at low temperatures and pressure, Chem. Commun., 2023, 59, 6052–6055 RSC.
|
This journal is © The Royal Society of Chemistry 2023 |
Click here to see how this site uses Cookies. View our privacy policy here.