DOI:
10.1039/D3SC02705H
(Edge Article)
Chem. Sci., 2023,
14, 9496-9502
Axially chiral styrene-based organocatalysts and their application in asymmetric cascade Michael/cyclization reaction†
Received
29th May 2023
, Accepted 16th August 2023
First published on 16th August 2023
Abstract
An axially chiral styrene-based organocatalyst, featuring a combination of axially chiral styrene-based structure and a pyrrole ring, has been designed and synthesized. This catalyst demonstrates remarkable capabilities in producing a wide range of densely substituted spirooxindoles that feature an alkyne-substituted quaternary stereogenic center. These spirooxindoles are generated through mild cascade Michael/cyclization reactions, resulting in high conversion rates and exceptional enantioselectivity. Our catalytic model, based on experiments, X-ray structure analysis and DFT calculations suggests that chiral matched π–π interactions and multiple H-bonds between the organocatalyst and substrates play significant roles in controlling the stereoselectivity of the reaction.
Introduction
Catalytic asymmetric synthesis has emerged as a crucial strategy for obtaining enantiomerically pure compounds, and the key to achieving efficient asymmetric catalysis is the development of efficient chiral catalysts and ligands.1 Axially chiral compounds, known for their intriguing atropisomerism, have played an important role in asymmetric synthesis. Binaphthyl and biphenyl axisymmetric skeletons have been extensively employed as catalysts and ligands in recent years, demonstrating decisive impact on asymmetric catalytic reactions.2 Additionally, various axially chiral catalysts with distinct structures3 have also been reported successively with the development of more methodological studies.4 In contrast, the development of asymmetric axially chiral styrene-based catalysts has been delayed due to synthetic challenges. Meanwhile, significant progress has been made in catalytic asymmetric synthesis of axially chiral styrene-based structures,5 making it possible to apply this structure to catalysts and ligands.6 Promising results have been achieved using axially chiral styrene-based catalysts/ligands in asymmetric synthesis, as exemplified by the work of Bin Tan6a and Bing-Feng Shi6b,d in the synthesis of chiral phosphoric, phosphoramidite, and carboxylic acid derivatives and their application in several asymmetric transformations. Recently, Feng Shi group designed and synthesized a novel thiourea catalyst containing axially chiral styrene, which was validated by calculation6c,e (Fig. 1A). These highly innovative studies have significantly broadened the scope of applications for axially chiral styrene and have inspired the development of novel axially chiral catalysts and ligands (Table 1).
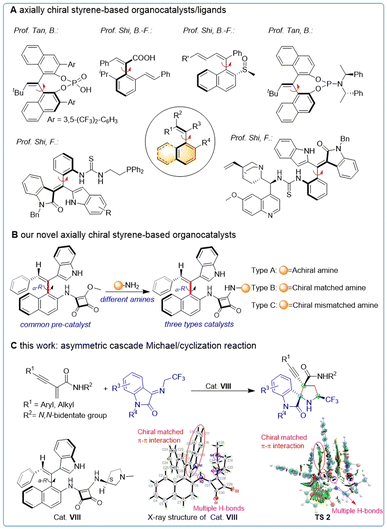 |
| Fig. 1 The developed axially chiral styrene-based organocatalysts/ligands and our work. | |
Table 1 Optimization of organocatalysta
Reaction conditions: 1a (0.1 mmol), 2a (0.15 mmol) and organocatalyst (10 mol%) in Toluene (1.0 mL) stirred at the indicated temperature for 24 h.
The ee value was determined by HPLC analysis.
Isolated yield.
The dr value was determined by 1H NMR analysis.
|
|
Based on our interest in synthesizing axially chirality,5b,7 as well as our experiences in constructing nitrogen-containing compounds,8 we designed and synthesized a series of axially chiral styrene-based organocatalysts with exocyclic double bonds. These catalysts were categorized into racemic amines, chiral matched amines, and chiral mismatched amines based on their different active groups (Fig. 1B).
Furthermore, spiro-[pyrrolidin-3,2-oxindoles] have been recognized as a crucial moiety in numerous natural products and clinical candidates.9 Here, we report the synthesis of a variety of spirooxindoles through asymmetric cascade Michael/cyclization reactions10 of enynamides8b and ketimines utilizing our axially chiral styrene-based organocatalysts. The X-ray structure analysis and DFT calculation of cat. VIII demonstrated its ability to promote stereoselective control of the reaction through π–π interactions and multiple H-bonds (Fig. 1C).
Results and discussion
Screening of the reaction conditions
We initiated our investigation by employing enynamide 1a and ketimine 2a as substrates, using squaramide catalyst I in toluene. Cat. I lacking an active group was found to be ineffective in catalyzing the reaction, hence we attempted to introduce triethylamine for catalysis. The intermolecular synergistic catalysis of cat. I and triethylamine exhibited superior results as compared to pure triethylamine catalysis, and the target product was obtained with a yield of 20%. The introduction of intramolecular basic groups such as pyridine (cat. II) and alkylamine (cat. III) in the catalyst promoted the reaction yield (45–49% yield). However, enantioselectivity control of these catalysts was inadequate (16–30% ee), and achieving chiral control of the reaction via a single axial chirality proved to be challenging. On the basis of these phenomena, we considered replacing the reactive site with an S-configuration chiral amine to create an enantiomeric match with the αR-configuration axially chiral styrene, thereby synergistically catalyzing the reaction. To our delight, catalysts IV–VIII, designed based on this approach, exhibited moderate to good capacity in controlling reactivity (52–78% yield) and stereoselectivity (>6
:
1 dr, 49–90% ee). The spatial structure of the optimal catalyst VIII was confirmed by X-ray crystallography. We speculate that the near-planar conformation of the enynamides may interact with the axially chiral portion of the catalyst structure, potentially enhancing the enantioselectivity of the reaction. The axially chirality of the styrene formed a suitable catalyst pocket with squaramide and pyrrole rings, and we hypothesized that the catalyst could promote chiral control of the reaction via chiral matched π–π interaction and multiple H-bonds. To validate our conjecture, we synthesized enantiomerically mismatched catalysts IX–XI. Cat. IX–X with mismatched chiral amines were unable to control the enantioselectivity (−29% to −37% ee) of the reaction, while cat. XI with mismatched π–π interaction exhibited poor stereoselectivity in terms of enantio- and diastereoselectivities (59% ee, 4
:
1 dr). Moreover, in this reaction, the catalytic performance of our novel organocatalyst was significantly superior to that of conventional commercially available catalysts (see ESI, Table S1†). Then, in the presence of the optimal catalyst VIII, the reaction conditions were further evaluated for solvents, temperature, and additives, revealing that the optimal reaction conditions were as follows: 1a (0.2 mmol), 2a (0.3 mmol), MgSO4 (60 mg), and 10 mol% cat. VIII in DCE (2.0 mL) at 0 °C for 24 h (see ESI, Table S2†).
Substrate scopes
After determining the optimal reaction conditions, we investigated the scope of application of the Michael/cyclization reaction. Frist, we expanded the scope of functionalized benzene rings in order to examine the generality of this asymmetric formal cascade Michael/cyclization reaction. As shown in Table 2, a wide range of enynamide 1 were utilized as competent substrates in the reaction with ketimine 2a. Methyl substitution at various positions on the benzene ring led to high yields (75–83%), ees (91–93%) and >9
:
1 dr (3ba–3da). Different substituents on the aromatic ring such as halogen (3ea–3ja), methoxy (3ka), tert-butyl (3la) and phenyl (3ma) were tolerated and no obvious electronic effect of the substituent was observed. Additionally, the reaction could be successfully extended to 3-thiophen-3-yl, naphthyl, and ferrocene delivering the desired products in 75–85% yield, 91–94% ees and >11
:
1 dr (3na–3pa).
Table 2 Substrate scopesa
Reaction conditions: 1 (0.2 mmol), 2 (0.3 mmol), MgSO4 (60 mg) and cat. VIII (10 mol%) in DCE (2.0 mL) stirred at 0 °C for 24 h.
|
|
Encouraged by these results, this methodology was applied to alkyl-substituted starting materials. Fortunately, these substrates (3qa–3ta) afforded the desired products with excellent stereoselectivity (91–97% ee, >5
:
1 dr), except for the tert-butyl substrate which displayed lower reactivity (27% yield). Notably, the silyl substituent in the chiral 3ua product allowed for a wide range of further transformations, leading to the construction of a synthetically useful skeleton in 62% yield, 92% ee and 9
:
1 dr, which could be readily converted into a terminal alkyne. In addition, other enynamides containing intramolecular hydrogen bonds could also efficiently participated in the reaction (3va–3xa). We also examined the influence of substituents on ketimine 2 on the reaction. Methyl-(3aa) and benzyl-protected (3ab) ketimines as well as unprotected ketimine (3ac) at the 1-position were also good 1,3-dipoles, resulting in good to high yields and excellent enantioselectivities and diastereoselectivities. Substituents with either electron-withdrawing or electron-donating groups residing at varying positions on the isatin ring were well tolerated (3ad–3ag), affording the desired products with high enantioselectivities (93–96% ee).
Synthetic application
To showcase the synthetic utility of this protocol, gram–scale reaction and derivatization reactions were briefly implemented (Fig. 2A). Substrate 1a (2 mmol) underwent the formal cascade Michael/cyclization reaction with 2a (3 mmol) at standard conditions, affording 3aa (886 mg) in 82% yield without erosion of optical purity. The estradiol derivative 1y was well tolerated and gave 3,2′-pyrrolidinyl spirooxindole 3ya in 76% yield with excellent stereochemical integrity and high diastereoselectivity (19
:
1 dr). These results indicated the utility of the reaction for late-stage modification of macromolecules. For spiro[pyrrolidin-3,2-oxindoles] 3aa, the quinoline moiety could be removed by treatment with 2-iodoxybenzoic acid (IBX), affording primary amide 4aa.11 Moreover, further treatment of 4aa with LiAlH4 at 0 °C in THF provided lactam 5aa containing a polyheterocyclic scaffold in 41% yield without compromising ee (Fig. 2C). The present protocol enables convenient access to various synthetically challenging stereochemically defined nitrogenous heterocycles bearing an alkyne-substituted quaternary carbon centre as important chiral building blocks in organic synthesis.
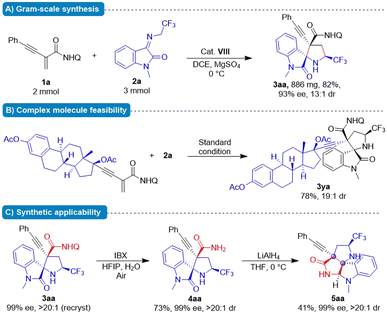 |
| Fig. 2 Studies on gram-scale reaction, applicability of complex molecules and further synthesis application. | |
Proposed mechanistic DFT calculations
In order to reveal the key role of the catalyst in the enantioselectivity control of the cascade Michael/cyclization reaction, we performed density functional theory (DFT) calculations at the M06-2X/6-311++G(d,p) (solvent = DCE, SMD)//M06-2X/6-31G(d,p) (vacuum) level to investigate the reaction pathway12 (see ESI, Fig. S4†). The computational analysis demonstrated that catalyst VIII efficiently activated both enynamide 1a and ketimine 2a by means of chiral matched π–π interactions and multiple H-bonds, leading to the formation of Int D. Subsequently, carbon nucleophile attacks the double bond, initiating asymmetric Michael addition and generating Int E, which possesses a newly formed chiral center. This is followed by intramolecular asymmetric Mannich reaction, resulting in the formation of Int F. Finally, the desired chiral spiro product 3aa is produced as it dissociates, effectively regenerating the catalyst (Fig. 3).
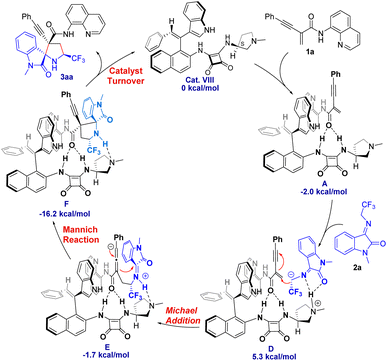 |
| Fig. 3 Proposed catalytic cycle. | |
To visualize the interaction between catalyst and substrates, we performed NCI analysis on noncovalent key interactions in TS2. As shown in Fig. 4, the substrate 1a, which is nearly planar,8b formed a more apparent chiral matched π–π interaction by being parallel and in close proximity to the axially chiral indole (Fig. 4). On the other hand, multiple N–H bonds in pyrrole and squaramide form multiple hydrogen bonds with the intermediate. These weak interactions fix the intermediate in the catalyst pocket and synergistically control the stereoselectivity of the reaction. Interestingly, similar catalytic phenomena had also occurred in the synthesis of nonbiaryl naphthalene-1,2-diamine reported previously by our group.7a
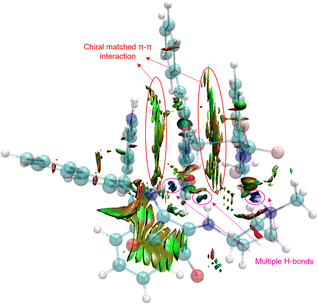 |
| Fig. 4 Visualization of noncovalent interactions in TS2. | |
The asymmetric addition of carbon anion to double bonds was a key step to control the enantioselectivity of the reaction. The conformations of the key transition states TS2 optimized by DFT along different attack directions were shown in Fig. 5. Compared to TS2′, TS2 has a lower relative Gibbs free energy barrier (ΔΔG = 2.0 kcal mol−1) due to the dual H-bond interaction between isatin and pyrrole.
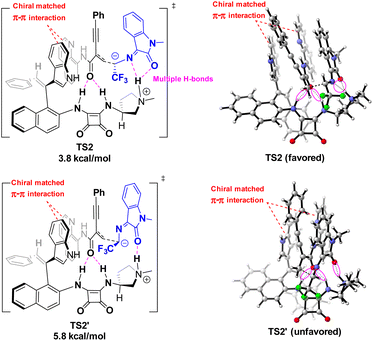 |
| Fig. 5 The structures of key nucleophilic attack transition states TS-2 at M06-2X/6-311++G(d,p) (solvent = DCE, SMD)//M06-2X/6-31G(d,p) (vacuum) level. | |
Conclusions
In summary, we have successfully developed a novel axially chiral styrene-based squaramide organocatalyst that combines axial chirality and a pyrrole structure to effectively control the stereoselectivity of the reaction. More importantly, based on experimental results and X-ray structural analysis, we established a catalytic model through DFT calculations, revealing that the catalytic pocket effectively regulates stereoselectivity via chiral matched π–π interactions and multiple H-bonds. In addition, we have achieved the synthesis of a diverse range of densely substituted spirooxindole compounds featuring alkynyl substituent quaternary stereogenic centers, with impressive outcomes such as high yields (up to 85%), excellent enantioselectivity (up to 96% ee), and high diastereomeric ratios (>20
:
1 dr). These findings contribute to a more comprehensive understanding of the catalyst and facilitate the design and development of axially styrene-based chiral catalysts and ligands in the future.
Data availability
All detailed procedures, characterization data, and spectra are available in the ESI.†
Author contributions
Y. Hao performed the experiments and analyzed the data. Z.-H. Li perform the theoretical calculations. Y. Hao, Z.-G. Ma, R.-X. Liu, R.-T. Ge, Q.-Z. Li, T.-M. Ding and Shu-Yu Zhang supervised the research and co-wrote the manuscript. S.-Y. Zhang conceived the project.
Conflicts of interest
The authors declare no competing financial interests.
Acknowledgements
This work was supported by the National Key Research and Development Program of China (2021YFF0701800 and 2022YFC2703400), and NSFC (no. 22071147).
Notes and references
- For selected reviews, see:
(a) S. Akutagawa, Appl. Catal., A, 1995, 128, 171–207 CrossRef CAS;
(b) X. Liu, L. Lin and X. Feng, Acc. Chem. Res., 2011, 44, 574–587 CrossRef CAS PubMed;
(c) A. H. Hoveyda, Y. Zhou, Y. Shi, M. K. Brown, H. Wu and S. Torker, Angew. Chem., Int. Ed., 2020, 59, 21304–21359 CrossRef CAS PubMed;
(d) A. F. Zahrt, S. V. Athavale and S. E. Denmark, Chem. Rev., 2020, 120, 1620–1689 CrossRef CAS PubMed;
(e) L. Wu, H. Wei, J. Shen, J. Chen and W. Zhang, Acta Chim. Sin., 2021, 79, 1331–1344 CrossRef CAS;
(f) X. Wang, Z. Han, Z. Wang and K. Ding, Acc. Chem. Res., 2021, 54, 668–684 CrossRef CAS PubMed;
(g) L. Süsse and B. M. Stoltz, Chem. Rev., 2021, 121, 4084–4099 CrossRef PubMed;
(h) H. Wang, J. Wen and X. Zhang, Chem. Rev., 2021, 121, 7530–7567 CrossRef CAS PubMed;
(i) X.-M. Zhang, B.-S. Li, S.-H. Wang, K. Zhang, F.-M. Zhang and Y.-Q. Tu, Chem. Sci., 2021, 12, 9262–9274 RSC ; for selected examples, see:;
(j) R. Zhang, S. Ge and J. Sun, J. Am. Chem. Soc., 2021, 143, 12445–12449 CrossRef CAS PubMed;
(k) B. Su and J. F. Hartwig, Angew. Chem., Int. Ed., 2022, 61, e202113343 CrossRef CAS PubMed.
- For selected reviews, see:
(a) K. Ding, H. Guo, X. Li, Y. Yuan and Y. Wang, Top. Catal., 2005, 35, 105–116 CrossRef CAS;
(b) T. Akiyama, Chem. Rev., 2007, 107, 5744–5758 CrossRef CAS PubMed;
(c) M. Terada, Chem. Commun., 2008, 4097–4112 RSC;
(d) M. Terada, Synthesis, 2010, 1929–1982 CrossRef CAS;
(e) D. Parmar, E. Sugiono, S. Raja and M. Rueping, Chem. Rev., 2014, 114, 9047–9153 CrossRef CAS PubMed;
(f) T. Akiyama and K. Mori, Chem. Rev., 2015, 115, 9277–9306 CrossRef CAS PubMed;
(g) Y.-B. Wang and B. Tan, Acc. Chem. Res., 2018, 51, 534–547 CrossRef CAS PubMed;
(h) G. Liao, T. Zhou, Q.-J. Yao and B.-F. Shi, Chem. Commun., 2019, 55, 8514–8523 RSC;
(i) J. A. Carmona, C. Rodríguez-Franco, R. Fernández, V. Hornillos and J. M. Lassaletta, Chem. Soc. Rev., 2021, 50, 2968–2983 RSC ; for selected examples, see:;
(j) B.-C. Da, S.-H. Xiang, S. Li and B. Tan, Chin. J. Chem., 2021, 39, 1787–1796 CrossRef CAS;
(k) K. Mori, T. Itakura and T. Akiyama, Angew. Chem., Int. Ed., 2016, 55, 11642–11646 CrossRef CAS PubMed;
(l) J.-M. Tian, A.-F. Wang, J.-S. Yang, X.-J. Zhao, Y.-Q. Tu, S.-Y. Zhang and Z.-M. Chen, Angew. Chem., Int. Ed., 2019, 58, 11023–11027 CrossRef CAS PubMed;
(m) O. M. Beleh, E. Miller, F. D. Toste and S. J. Miller, J. Am. Chem. Soc., 2020, 142, 16461–16470 CrossRef CAS PubMed;
(n) X.-J. Zhao, Z.-H. Li, T.-M. Ding, J.-M. Tian, Y.-Q. Tu, A.-F. Wang and Y.-Y. Xie, Angew. Chem., Int. Ed., 2021, 60, 7061–7065 CrossRef CAS PubMed;
(o) Z. Bai, H. Zhang, H. Wang, H. Yu, G. Chen and G. He, J. Am. Chem. Soc., 2021, 143, 1195–1202 CrossRef CAS PubMed.
- For selected examples, see:
(a) T. Mino, K. Nishikawa, M. Asano, Y. Shima, T. Ebisawa, Y. Yoshida and M. Sakamoto, Org. Biomol. Chem., 2016, 14, 7509–7519 RSC;
(b) G. Liao, H.-M. Chen, Y.-N. Xia, B. Li, Q.-J. Yao and B.-F. Shi, Angew. Chem., Int. Ed., 2019, 58, 11464–11468 CrossRef CAS PubMed;
(c) T. He, L. Peng, S. Li, F. Hu, C. Xie, S. Huang, S. Jia, W. Qin and H. Yan, Org. Lett., 2020, 22, 6966–6971 CrossRef CAS PubMed;
(d) Q.-J. Yao, P.-P. Xie, Y.-J. Wu, Y.-L. Feng, M.-Y. Teng, X. Hong and B.-F. Shi, J. Am. Chem. Soc., 2020, 142, 18266–18276 CrossRef CAS PubMed;
(e) Q.-J. An, W. Xia, W.-Y. Ding, H.-H. Liu, S.-H. Xiang, Y.-B. Wang, G. Zhong and B. Tan, Angew. Chem., Int. Ed., 2021, 60, 24888–24893 CrossRef CAS PubMed.
- For selected reviews, see:
(a) R. Song, Y. Xie, Z. Jin and Y. R. Chi, Angew. Chem., Int. Ed., 2021, 60, 26026–26037 CrossRef CAS PubMed;
(b) J. K. Cheng, S.-H. Xiang, S. Li, L. Ye and B. Tan, Chem. Rev., 2021, 121, 4805–4902 CrossRef CAS PubMed;
(c) H.-H. Zhang and F. Shi, Acc. Chem. Res., 2022, 55, 2562–2580 CrossRef CAS PubMed;
(d) W. Qin, Y. Liu and H. Yan, Acc. Chem. Res., 2022, 55, 2780–2795 CrossRef CAS PubMed ; for selected examples, see:;
(e) K. T. Barrett, A. J. Metrano, P. R. Rablen and S. J. Miller, Nature, 2014, 509, 71–75 CrossRef CAS PubMed;
(f) Y. Kwon, J. Li, J. P. Reid, J. M. Crawford, R. Jacob, M. S. Sigman, F. D. Toste and S. J. Miller, J. Am. Chem. Soc., 2019, 141, 6698–6705 CrossRef CAS PubMed;
(g) Q.-H. Nguyen, S.-M. Guo, T. Royal, O. Baudoin and N. Cramer, J. Am. Chem. Soc., 2020, 142, 2161–2167 CrossRef CAS PubMed;
(h) T. Li, C. Mou, P. Qi, X. Peng, S. Jiang, G. Hao, W. Xue, S. Yang, L. Hao, Y. R. Chi and Z. Jin, Angew. Chem., Int. Ed., 2021, 60, 9362–9367 CrossRef CAS PubMed;
(i) J. Yang, J.-W. Zhang, W. Bao, S.-Q. Qiu, S. Li, S.-H. Xiang, J. Song, J. Zhang and B. Tan, J. Am. Chem. Soc., 2021, 143, 12924–12929 CrossRef CAS PubMed;
(j) G. Liao, T. Zhang, L. Jin, B.-J. Wang, C.-K. Xu, Y. Lan, Y. Zhao and B.-F. Shi, Angew. Chem., Int. Ed., 2022, 61, e202115221 CrossRef CAS PubMed;
(k) K.-W. Chen, Z.-H. Chen, S. Yang, S.-F. Wu, Y.-C. Zhang and F. Shi, Angew. Chem., Int. Ed., 2022, 61, e202116829 CAS;
(l) R. R. Surgenor, X. Liu, M. J. H. Keenlyside, W. Myers and M. D. Smith, Nat. Chem., 2023, 15, 357–365 CrossRef CAS PubMed;
(m) R. Mi, Z. Ding, S. Yu, R. H. Crabtree and X. Li, J. Am. Chem. Soc., 2023, 145, 8150–8162 CrossRef CAS PubMed.
- For selected reviews, see:
(a) J. Feng and Z. Gu, SynOpen, 2021, 05, 68–85 CrossRef CAS;
(b) Z.-H. Li, Q.-Z. Li, H.-Y. Bai and S.-Y. Zhang, Chem. Catal., 2023, 3, 100594 CrossRef CAS ; for selected examples, see:;
(c) S.-C. Zheng, S. Wu, Q. Zhou, L. W. Chung, L. Ye and B. Tan, Nat. Commun., 2017, 8, 15238 CrossRef PubMed;
(d) J. D. Jolliffe, R. J. Armstrong and M. D. Smith, Nat. Chem., 2017, 9, 558–562 CrossRef CAS PubMed;
(e) S. Jia, Z. Chen, N. Zhang, Y. Tan, Y. Liu, J. Deng and H. Yan, J. Am. Chem. Soc., 2018, 140, 7056–7060 CrossRef CAS PubMed;
(f) S. Zhu, Y.-H. Chen, Y.-B. Wang, P. Yu, S.-Y. Li, S.-H. Xiang, J.-Q. Wang, J. Xiao and B. Tan, Nat. Commun., 2019, 10, 4268 CrossRef PubMed;
(g) L. Jin, Q.-J. Yao, P.-P. Xie, Y. Li, B.-B. Zhan, Y.-Q. Han, X. Hong and B.-F. Shi, Chem, 2020, 6, 497–511 CrossRef CAS;
(h) Y. Liang, J. Ji, X. Zhang, Q. Jiang, J. Luo and X. Zhao, Angew. Chem., Int. Ed., 2020, 59, 4959–4964 CrossRef CAS PubMed;
(i) J. Wang, X. Qi, X.-L. Min, W. Yi, P. Liu and Y. He, J. Am. Chem. Soc., 2021, 143, 10686–10694 CrossRef CAS PubMed;
(j) C. Yang, T.-R. Wu, Y. Li, B.-B. Wu, R.-X. Jin, D.-D. Hu, Y.-B. Li, K.-J. Bian and X.-S. Wang, Chem. Sci., 2021, 12, 3726–3732 RSC;
(k) C. Zhang, Z. Tang, Y. Qiu, J. Tang, S. Ye, Z. Li and J. Wu, Chem. Catal., 2022, 2, 164–177 CrossRef CAS;
(l) S.-Q. Qiu, Y. Chen, X.-J. Peng, S.-J. He, J. K. Cheng, Y.-B. Wang, S.-H. Xiang, J. Song, P. Yu, J. Zhang and B. Tan, Angew. Chem., Int. Ed., 2022, 61, e202211211 CrossRef CAS PubMed;
(m) J.-L. Yan, R. Maiti, S.-C. Ren, W. Tian, T. Li, J. Xu, B. Mondal, Z. Jin and Y. R. Chi, Nat. Commun., 2022, 13, 84 CrossRef CAS PubMed;
(n) B. Cai, Y. Cui, J. Zhou, Y.-B. Wang, L. Yang, B. Tan and J. Wang, Angew. Chem., Int. Ed., 2023, 62, e202215820 CrossRef CAS PubMed;
(o) D. Xu, Y. Chang, Y. Liu, W. Qin and H. Yan, ACS Catal., 2023, 13, 2957–2967 CrossRef CAS.
-
(a) Y.-B. Wang, P. Yu, Z.-P. Zhou, J. Zhang, J. Wang, S.-H. Luo, Q.-S. Gu, K. N. Houk and B. Tan, Nat. Catal., 2019, 2, 504–513 CrossRef CAS;
(b) H. Song, Y. Li, Q.-J. Yao, L. Jin, L. Liu, Y.-H. Liu and B.-F. Shi, Angew. Chem., Int. Ed., 2020, 59, 6576–6580 CrossRef CAS PubMed;
(c) C. Ma, F.-T. Sheng, H.-Q. Wang, S. Deng, Y.-C. Zhang, Y. Jiao, W. Tan and F. Shi, J. Am. Chem. Soc., 2020, 142, 15686–15696 CrossRef CAS PubMed;
(d) L. Jin, P. Zhang, Y. Li, X. Yu and B.-F. Shi, J. Am. Chem. Soc., 2021, 143, 12335–12344 CrossRef CAS PubMed;
(e) S.-J. Liu, Z.-H. Chen, J.-Y. Chen, S.-F. Ni, Y.-C. Zhang and F. Shi, Angew. Chem., Int. Ed., 2022, 61, e202112226 CAS.
-
(a) H.-Y. Bai, F.-X. Tan, T.-Q. Liu, G.-D. Zhu, J.-M. Tian, T.-M. Ding, Z.-M. Chen and S.-Y. Zhang, Nat. Commun., 2019, 10, 3063 CrossRef PubMed;
(b) Q.-Z. Li, Z.-H. Li, J.-C. Kang, T.-M. Ding and S.-Y. Zhang, Chem. Catal., 2022, 2, 3185–3195 CrossRef CAS.
-
(a) J.-W. Dong, T. Ding, S.-Y. Zhang, Z.-M. Chen and Y.-Q. Tu, Angew. Chem., Int. Ed., 2018, 57, 13192–13196 CrossRef CAS PubMed;
(b) Z.-G. Ma, J.-L. Wei, J.-B. Lin, G.-J. Wang, J. Zhou, K. Chen, C.-A. Fan and S.-Y. Zhang, Org. Lett., 2019, 21, 2468–2472 CrossRef CAS PubMed;
(c) H. Shao, K. Fang, Y.-P. Wang, X.-M. Zhang, T.-M. Ding, S.-Y. Zhang, Z.-M. Chen and Y.-Q. Tu, Org. Lett., 2020, 22, 3775–3779 CrossRef CAS PubMed;
(d) Q.-Z. Li, S.-H. Hou, J.-C. Kang, P.-F. Lian, Y. Hao, C. Chen, J. Zhou, T.-M. Ding and S.-Y. Zhang, Angew. Chem., Int. Ed., 2022, 61, e202207088 CrossRef CAS PubMed;
(e) X.-Y. Qiu, Z.-H. Li, J. Zhou, P.-F. Lian, L.-K. Dong, T.-M. Ding, H.-Y. Bai and S.-Y. Zhang, ACS Catal., 2022, 12, 7511–7516 CrossRef CAS.
- For selected reviews, see:
(a) C. V. Galliford and K. A. Scheidt, Angew. Chem., Int. Ed., 2007, 46, 8748–8758 CrossRef CAS PubMed;
(b) R. Dalpozzo, G. Bartoli and G. Bencivenni, Chem. Soc. Rev., 2012, 41, 7247–7290 RSC;
(c) D. Cheng, Y. Ishihara, B. Tan and C. F. Barbas III, ACS Catal., 2014, 4, 743–762 CrossRef CAS.
- For selected reviews, see:
(a) L. Klier, F. Tur, P. H. Poulsen and K. A. Jørgensen, Chem. Soc. Rev., 2017, 46, 1080–1102 RSC;
(b) A. Ding, M. Meazza, H. Guo, J. W. Yang and R. Rios, Chem. Soc. Rev., 2018, 47, 5946–5996 RSC;
(c) M. Ma, Y. Zhu, Q. Sun, X. Li, J. Su, L. Zhao, Y. Zhao, S. Qiu, W. Yan, K. Wang and R. Wang, Chem. Commun., 2015, 51, 8789–8792 RSC;
(d) Y. You, W.-Y. Lu, Z.-H. Wang, Y.-Z. Chen, X.-Y. Xu, X.-M. Zhang and W.-C. Yuan, Org. Lett., 2018, 20, 4453–4457 CrossRef CAS PubMed;
(e) B. Niu, X.-Y. Wu, Y. Wei and M. Shi, Org. Lett., 2019, 21, 4859–4863 CrossRef CAS PubMed;
(f) W. C. Jang, M. Jung and H. M. Ko, Org. Lett., 2021, 23, 1510–1515 CrossRef CAS PubMed;
(g) L. Wei and C.-J. Wang, Chem. Commun., 2021, 57, 10469–10483 RSC;
(h) J.-A. Xiao, X.-L. Cheng, H. Peng, J.-S. Liang and W. Su, Chem.–Asian J., 2021, 16, 2435–2438 CrossRef CAS PubMed.
- Z. Zhang, X. Li, M. Song, Y. Wan, D. Zheng, G. Zhang and G. Chen, J. Org. Chem., 2019, 84, 12792–12799 CrossRef CAS PubMed.
-
(a) N. M. Rezayee, V. J. Enemærke, S. T. Linde, J. N. Lamhauge, G. J. Reyes-Rodríguez, K. A. Jørgensen, C. Lu and K. N. Houk, J. Am. Chem. Soc., 2021, 143, 7509–7520 CrossRef CAS PubMed;
(b) Q. Yan, M. Duan, C. Chen, Z. Deng, M. Wu, P. Yu, M.-L. He, G. Zhu, K. N. Houk and J. Sun, Chem. Sci., 2022, 13, 5767–5773 RSC;
(c) M. A. Maskeri, A. J. Fernandes, G. Di Mauro, N. Maulide and K. N. Houk, J. Am. Chem. Soc., 2022, 144, 23358–23367 CrossRef CAS PubMed.
|
This journal is © The Royal Society of Chemistry 2023 |
Click here to see how this site uses Cookies. View our privacy policy here.