DOI:
10.1039/D2SD00177B
(Communication)
Sens. Diagn., 2023,
2, 262-267
Discriminative ‘turn-on’ fluorescence sensing of volatile halogenated solvents using a cleft-shaped 4-amino-1,8-naphthalimide Tröger's base fluorophore†‡
Received
8th October 2022
, Accepted 28th December 2022
First published on 29th December 2022
Abstract
A cleft-shaped 2-picolyl-4-amino-1,8-naphthalimide Tröger's base (TBNap) was synthesized and employed as a fluorescent ‘turn-on’ chemosensor for the discriminative sensing of volatile halogenated solvents based on an ICT sensing mechanism. TBNap displayed comparatively high fluorescence emission enhancement for chloroform – a potent anesthetic agent and a major constituent of tobacco smoke. The discriminative fluorescence sensing was also seen through visual color changes and was further validated using computational calculations.
Introduction
The design of easily adaptable sensing methods for detecting volatile organic solvents (VOSs) has attracted widespread research attention recently because of their serious menace to the living system and severe environmental pollution.1–5 Volatile halogenated solvents (VHSs) are one type of VOSs with one or more halogen atoms.6,7 The widespread usage of VHSs in numerous industrial settings and research laboratories increases the risk of serious environmental contamination and workplace explosions owing to their high volatility.8,9 Several health hazards due to excessive exposure to the vapors of VHSs include eye irritation, heart diseases, intensive headaches, kidney failure, and respiratory malfunction, all of which could be fatal, and halogenated solvents are also known to cause cancer.10–13
In general, VHSs are harmful and difficult to decompose; thus, VHSs must be treated or transformed into innocuous products before releasing to the atmosphere.14,15 VHSs can also react in the atmosphere to produce several toxic gaseous species.16 Consequently, the precise detection and differentiation of VHSs are of significant importance and are acknowledged as a prerequisite for their appropriate use and effective treatment. Therefore, developing a suitable sensing method for the discriminative sensing of VHSs is important to mitigate their detrimental effect on human health and the environment. Several analytical techniques, including high-performance chromatography, FT-IR spectroscopy, and gas-chromatography mass-spectrometry (GC-MS), are available to detect VHSs.17–19 However, the tedious and multi-step procedure, high-maintenance cost, requirement of a trained operator, and inoperable on-field analysis restrict the applications of these instrumental techniques for the efficient detection and monitoring of VHSs. Hence, there is an increased interest in developing simple and cost-effective sensor systems for precisely sensing VHSs.
Because of its various advantageous features, such as simple operation, cost-effective instrumentation, unique selectivity, high sensitivity, fast-sensing responses, and easy visualization, fluorescence-based sensing has evolved as an effective detection method.20–25 Many solvatochromic fluorescence probes have been reported for the discriminative sensing of VOSs.26–28 The fluorescence emission of solvatochromic probes highly depends on the solvent polarity, and slight changes in the polarity of the medium can greatly affect their fluorescence emission properties. Until now, a wide variety of solvatochromic fluorescence sensors have been known. Most of them are limited in their practical applications due to their low fluorescence quantum yield, less molar absorption coefficient, and poor photostability.29,30
4-amino-1,8-naphthalimide Tröger's bases (TBNaps) belong to a significant class of ‘V-shaped’ supramolecular scaffolds with tremendous applications in chemosensing, medicinal and materials chemistry.31–34 Owing to their unique cleft structure with a large-hydrophobic cavity and strong fluorescence emission due to internal-charge transfer (ICT) transition, TBNaps have been used as potential hosts in selective complexation of suitable guests and as fluorescence chemosensors for the detection of numerous analytes.1,28,31–34 Herein, we report a new ‘turn-on’ fluorescence sensor, bis-[N-(2-pyridyl)methyl)]-9,18-methano-1,8-naphthalimide-[b,f][1,5]diazocine (TBNap, see Scheme 1), for the discriminative fluorescence sensing of closely-related VHSs. TBNap exhibited a relatively high fluorescence enhancement for CHCl3 over other structurally similar VHSs. TBNap can also be used as a naked-eye fluorescence sensor for discriminative identification of VHSs in the solution phase.
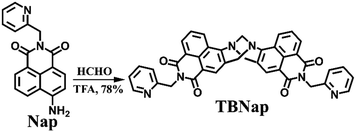 |
| Scheme 1 Synthesis of TBNap from Nap. | |
Experimental
Materials and methods
All reagents, solvents, and starting materials were purchased from Sigma-Aldrich and were used as received. Commercially available reagent-grade chemicals were used for the synthesis of Nap and TBNap. High-purity analytical grade halogenated solvents were purchased from Merck and used as received. Commercially available spectroscopy-grade solvents from Merck were used without further purification. 4-Nitro-1,8-naphthalic anhydride, 2-picolylamine, Pd/C (10%), hydrazine monohydrate (H2N–NH2·H2O), paraformaldehyde, and trifluoroacetic acid (TFA) were purchased from Sigma-Aldrich were reagent grade and used as received. A deuterated solvent, (CD3)2SO, used for NMR analyses was purchased from Sigma-Aldrich. The precursors, N-[(2-pyridyl)methyl]-4-nitro-1,8-naphthalimide and N-[(2-pyridyl)methyl]-4-amino-1,8-naphthalimide (Nap), were synthesized following the procedure reported in the literature.32,35
An electrochemical IA9000 digital melting point apparatus was used for measuring the melting point of TBNap in an unsealed capillary tube. The Shimadzu Scientific Instruments (IR Tracer 100) equipped with an ATR sampler were used for recording the FT-IR spectrum. All NMR spectra were recorded on a Bruker-DPX-Avance spectrometer operating at 400 MHz for 1H NMR and 101 MHz for 13C NMR in a commercially available deuterated solvent. Chemical shifts are reported in parts-per-million (ppm) relative to the internal solvent (CD3)2SO. All NMR data were processed with Bruker Win-NMR 5.0, Topspin, and MestReNova software. Multiplicities were abbreviated as follows: singlet (s), doublet (d), and triplet (t). HRMS was performed on a Shimadzu mass spectrometer using positive ionization and a linear detector. The m/z values were recorded over a range of 100–2000. HPLC-grade CH3CN or CH3OH were used as carrier solvents. The UV-visible absorption spectra were recorded in a 1 cm quartz cuvette on a Thermo Fisher scientific spectrometer, and baseline correction was applied for all spectra. The emission spectra for all the experiments were recorded on a Perkin Elmer FL-8500 fluorescence spectrophotometer equipped with a xenon arc (150 W) light source. The temperature was kept constant throughout the measurements at 298 K. All spectroscopic measurements were recorded in a quartz cuvette with a scan rate of 1200 nm min−1. The pH-dependent emission studies were performed using an OAKLON pH 550 meter. The obtained results were plotted using Origin Pro 8.5 software.
Synthesis of bis-[N-(2-pyridyl)methyl)]-9,18-methano-1,8-naphthalimide-[b,f][1,5]diazocine (TBNap)
Compound Nap (100 mg, 0.33 mmol, 1 equiv.) and paraformaldehyde (15 mg, 0.49 mmol, 1.5 equiv.) were taken in a 25 mL RB flask and flushed with nitrogen. Trifluoroacetic acid (3 mL) was added at 0 °C, and the solution was stirred at room temperature for 12 h under a nitrogen atmosphere. The reaction mixture was then added dropwise to aqueous ammonium hydroxide (30 mL) at 0 °C, and ammonia solution was added until a pH > 10 was achieved. The residue was extracted in dichloromethane (2 × 100 mL), and the organic layer was washed successively with saturated NaHCO3 (2 × 30 mL), brine (2 × 30 mL) and H2O (2 × 30 mL). The solution was dried over MgSO4, and the solvents were removed under reduced pressure to isolate compound TBNap (167 mg, 0.26 mmol, 78%) as a bright-yellow powder after trituration with cold-diethyl ether. Melting point 280–283 °C (decomp.). HRMS m/z: calcd for C39H27N6O4 [M + H+] 643.21, found 643.25; 1H NMR (400 MHz, DMSO-d6) δ 8.77–8.75 (2H, d, J = 8.0 Hz, Naph-H), 8.48–8.46 (2H, d, J = 8.0 Hz, Naph-H), 8.31–8.30 (2H, d, J = 4.0 Hz, picolyl-H), 8.12 (2H, s, Naph-H), 7.99–7.97 (2H, t, J = 8.0 Hz, Naph-H), 7.69–7.65 (2H, t, J = 16 Hz, picolyl-H), 7.28–7.26 (2H, d, J = 8 Hz, picolyl-H), 7.19–7.17 (2H, t, J = 8 Hz, picolyl-H), 5.29 (4H, s, CH2–H), 5.20–5.16 (2H, J = 16 Hz, NCH2–H), 4.74–4.70 (1H (s), 2H (d), J = 16 Hz, NCH2–H); 13C NMR (101 MHz, DMSO-d6) δ 163.93, 163.35, 156.28, 149.75, 149.30, 137.11, 131.42, 131.00, 130.90, 129.86, 128.09, 127.72, 127.32, 126.75, 122.81, 122.51, 121.20, 118.02, 67.59, 57.25, 44.51; FT-IR νmax (ATR, cm−1) 3554, 3068, 2953, 1697, 1654, 1618, 1591, 1570, 1510, 1475, 1460, 1435, 1404, 1377, 1332, 1294, 1259, 1234, 1168, 1153, 1130, 1089, 1051, 1028, 1012, 962, 945, 914, 869, 837, 804, 785, 754, 686, 661, 630, 609, 574, 555, 518, 476.
Fluorescence sensing studies
The stock solution (0.15 mM) of TBNap in the selected volatile halogenated solvents was prepared and utilized for spectroscopic titration studies. The emission spectra were recorded by diluting 200 μL stock solution of TBNap in the respective VHS with 1800 μL of the corresponding VHS in a quartz cell of 1 cm width. A similar titration procedure was carried out for all the VHSs separately, and changes in the emission pattern were measured. For the titration experiment, 100 μL of TBNap stock solution in CCl4 was diluted to 1000 μL using fresh CCl4, and emission changes were recorded. Chloroform was incrementally added to the above solution (10 μL each), and the spectra were recorded at an excitation wavelength (λex) of 380 nm, an excitation slit width of 10 nm, and an emission slit width of 5 nm.
pH-dependent emission studies
To the 0.01 mM solution of TBNap, 1.0 mM HCl solution was added gradually to prepare acidic solutions, and the pH of the solution was determined, followed by the recording of fluorescence emission spectra at different pHs. The basic solution was prepared by adding 1.0 mM NaOH solution to the 0.01 mM solution of TBNap, and the fluorescence emission was measured at different pHs of the solution. All the measurements were performed at room temperature.
Results and discussion
Synthesis and characterization of TBNap
As shown in Scheme 1, the chemosensor TBNap was synthesized from its corresponding amine precursor, N-(2-pyridyl)methyl-4-amino-1,8-naphthalimide (Nap), which was readily obtained from N-(2-pyridyl)methyl-4-nitro-1,8-naphthalimide via Pd/C mediated catalytic hydrogenation reaction.33,35 The cyclization of freshly synthesized Nap with paraformaldehyde (1.5 equiv.) in trifluoroacetic acid yielded the desired product TBNap as a bright-yellow powder in a 78% isolated yield (for details, see the Experimental section). TBNap was soluble in most common solvents and was fully analyzed using various standard spectroscopic techniques, such as FT-IR, multinuclear (1H and 13C) NMR, and HRMS. Further, the structure of TBNap was modeled using computational calculations.
The FT-IR spectrum of TBNap displays the characteristic C
Oimide, C
Npyridyl, and C–NTröger’s base functional group stretching vibrational bands at ν = 1697, 1652 and 1232 cm−1, respectively (Fig. S1, ESI‡). In the 1H NMR spectrum of TBNap recorded in DMSO-d6, the presence of two-well resolved doublets in the range of δ = 5.20–4.69 ppm corresponds to the methylene (–CH2N–) proton resonances of the bridge-head diazocine unit. The aromatic proton signals for the 2-picolyl and 1,8-naphthalimide moieties were observed between δ = 8.77 and 7.17 ppm. The 2-picolyl methylene (CH2) proton resonance appeared at δ = 5.29 ppm (Fig. 1A). The high-resolution mass-spectrometry (HRMS) analysis showed an intense peak at m/z = 643.2074 corresponding to the [M + H]+ molecular ion (Fig. S3, ESI‡). The energy-minimized structure of TBNap demonstrates its unique cleft-shaped conformation, in which the bridge-head diazocine unit with an angle of 95.86° places the two 1,8-naphthalimide moieties perpendicular to each other at an inter-planar distance of 7.29 Å. Notably, the two 2-picolyl moieties are flanked outwards from the hydrophobic cavity, possibly to avoid the steric hindrance (Fig. 1B). TBNap is a typical push–pull type of fluorophore, which is evidenced by the electron-density distribution. The HOMO electron density is more localized on the Tröger's base moiety. At the same time, the LUMO is primarily distributed at the imide unit (Fig. 1C). Therefore, on excitation, there is an internal-charge transfer transition from the electron-rich Tröger's base moiety to the electron-deficient imide sites, which makes TBNap a highly emissive fluorophore.
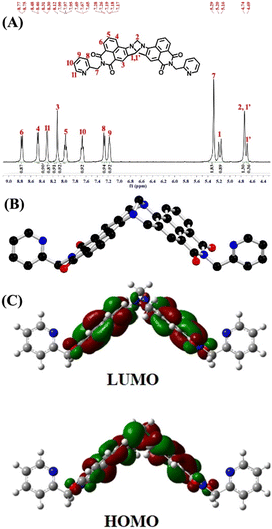 |
| Fig. 1 (A) 1H NMR spectrum of TBNap (400 MHz, DMSO-d6) with peak assignment. (B) Energy-minimized structure of TBNap (colour code: black C, red O, and blue N). Hydrogen atoms are omitted for clarity. (C) The frontier molecular orbitals of TBNap. | |
Fluorescence sensing studies
After the successful synthesis and characterization, we next analyzed the photophysical properties of TBNap in various organic solvents. TBNap was dissolved/well-dispersed in different solvents, namely, chloroacetyl chloride (CAC), tetrachloromethane (TCM), chloroform (CFM), cyclohexyl bromide (CHB), dichloromethane (DCM), dichloroethane (DCE) and epichlorohydrin (ECH), bromobenzene (BrB), butyl-benzene (BuB), propyl-benzene (PrB), tetrahydrofuran (THF) and acetonitrile (ACN). The UV-vis absorption spectra of TBNap in different organic solvents display the characteristic bands of a high-energy absorption band at λ = 340–355 nm corresponding to the π–π* transition and a low-energy broad band centered at λ = 348–385 nm ascribed to the internal charge transfer (ICT) transition (Fig. S4, ESI‡). It is worth mentioning that no significant shift in the absorption maxima (λmax) was observed in the electronic absorption spectra of TBNap recorded in different organic solvents (except in chloroacetyl chloride), and this indicates that the solvent effect in the ground state was inconsequential. However, the notable differences in the absorption intensity in different solvents were presumably due to their solubility difference in the selected solvents (Fig. S4, ESI‡).
The fluorescence emission spectra of TBNap were measured in various organic solvents. As expected, TBNap in different solvent media exhibited various fluorescence emission profiles. The calculated quantum yield for TBNap in different solvents was in the range of ϕ = 0.05 to 4.15 (see Table S1, ESI‡). The pH-dependent fluorescence emission studies revealed that at basic pH > 8, TBNap was highly emissive, but at acidic pH < 4, the emission intensity decreased due to the protonation of the NH2 group (Fig. S5, ESI‡). Furthermore, the concentration-dependent fluorescence emission study showed that the emission intensity of TBNap was enhanced upon gradually increasing its concentration (Fig. S6, ESI‡). Among various organic solvents, interestingly, TBNap in chloroform (CFM) medium displayed the highest fluorescence emission intensity, which is accompanied by significant redshifts in emission maxima (λmax) (Fig. 2A and B). From the emission profile, the relative number-fold emission enhancement was calculated by taking TBNap in tetrachloromethane (TCM) as the reference medium. Among the chosen solvents, TBNap in CFM solution displayed the highest number-fold emission change of about 9.3. In contrast, the CAC solution displayed the least value of 0.3 (Fig. 2C). The fluorescence emission enhancement order was as follows: CFM > BrB > PrB > DCM > BuB > CHB > DCE > THF > ECH > ACN > CAC. These variations in the emission intensity pattern in different solvents demonstrate that TBNap can clearly distinguish the closely related VHSs and sense a particular solvent system. Notably, the observed difference in fluorescence enhancement among the chosen chlorinated solvents indicates that no photodissociation occurs in the solution, and the discriminative fluorescence sensing of VHSs is primarily due to the difference in the solvent polarity.
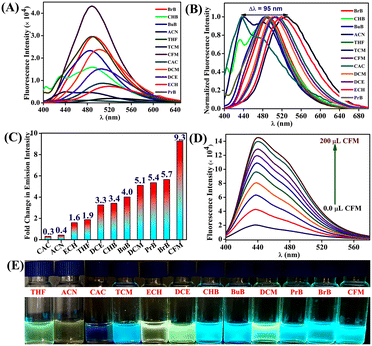 |
| Fig. 2 (A) The measured relative changes in the fluorescence emission intensity of TBNap in different organic solvents and (B) the corresponding normalized emission spectra showing the bathochromic shift in the emission maxima. (C) Number-fold changes in the emission intensity of TBNap concerning its emission in TCM. (D) The observed increases in the fluorescence emission of TBNap dissolved in CCl4 upon increasing the volume percentage of CFM. (E) The photograph of the visual color change was imaged in different organic solvents under UV-light irradiation. | |
To get further insight into the fluorescence sensing properties of TBNap, we performed fluorescence titration studies in a CFM medium. Upon incremental volume addition of CFM to TBNap solution in TCM, we observed a gradual increase in the fluorescence emission intensity at λmax = 440 nm (Fig. 2D). From the titration profile, the plot between the fluorescence emission enhancements and the volume percentage of CFM displays a linear relationship with a slope of 1.8 × 104. This linear curve indicates the potential of TBNap to quantitatively determine the volume percentage of the CFM content in other organic solvent media (Fig. S7, ESI‡). In addition, the normalized fluorescence emission spectra of TBNap in the chosen solvents display a perfect bathochromic shift (Δλ = 95 nm) from the blue to the red region (Fig. 2B). Solvents with a high dielectric constant exhibited emission at a higher wavelength, and solvents with a low dielectric constant emitted in the low-wavelength region. For example, TBNap in TCM with a dielectric constant of 2.24 exhibited the emission maxima at λ = 436 nm. In contrast, TBNap in ACN, having a ten times higher dielectric constant (37.5), displayed the emission maxima at λ = 530 nm (see Table S1, ESI‡). The redshift was due to the stabilization of the excited-state dipole of TBNap in the solvent medium with a high dielectric constant that decreases the HOMO–LUMO energy difference and enhances the possibility of strong ICT transition leading to an increase in the wavelength.7 On top of this, TBNap can effectively discriminate the chosen closely-related VHSs via naked-eye visualization (Fig. 2E). The shift in color of TBNap from blue to yellow as a function of an increase in the solvent dielectric constant was observed under a UV lamp. In particular, the commonly used volatile halogenated solvents TCE, CFM, and DCM, with a slight difference in their structure, displayed a remarkable shift in the color from blue to green, respectively, which is even observable with the naked eye (Fig. S8, ESI‡). Thus, the developed sensor TBNap can be used as a practically useful colorimetric probe for discriminative sensing of volatile halogenated solvents.
The observed discriminative ‘turn-on’ fluorescence sensing of TBNap in different VHSs was further validated by computational analysis using density functional theory (DFT) calculations. All the calculations were done at the B3LYP/6-311++G**//B3LYP/6-31G* level of theory by using the Gaussian 16 program.36 We calculated the HOMO and LUMO energies of all the chosen VHSs and compared them with TBNap frontier molecular orbitals. As given in Table 1, the calculated LUMO energy of all the chosen solvents was found to be higher in energy than the LUMO energy of TBNap, which favors the possibility of energy transfer from VHSs to TBNap and, thus, results in the fluorescence emission enhancement. The calculated energy difference between the LUMO of CFM and TBNap was comparatively low, which indicates the enhanced energy transfer from CFM to TBNap and, therefore, a greater fluorescence enhancement. The order of emission enhancement is in good agreement with the calculated energy differences and dielectric constants of various VHSs, which supports that the observed discriminative fluorescence ‘turn-on’ sensing of VHSs follows an ICT-based sensing mechanism.
Table 1 The HOMO and LUMO energies of TBNap and various VHSs compared to the number-fold emission enhancement
Compounds |
HOMO (eV) |
LUMO (eV) |
Fold-changes in emission intensity |
TBNap
|
−6.298 |
−2.694 |
— |
CFM |
−8.752 |
−1.595 |
9.3 |
BrB |
−6.906 |
−0.887 |
5.7 |
PrB |
−7.574 |
−0.701 |
5.4 |
DCM |
−8.594 |
−0.913 |
5.1 |
BuB |
−7.557 |
−0.621 |
4.0 |
CHB |
−7.442 |
−0.653 |
3.4 |
DCE |
−8.322 |
−0.607 |
3.3 |
ECH |
−7.972 |
−0.483 |
1.6 |
Conclusions
In conclusion, we have carefully designed and developed a novel 2-picolyl-4-amino-1,8-naphthalimide Tröger's base (TBNap) and demonstrated its application as a turn-on fluorescence sensor for the discriminative sensing of volatile halogenated solvents. The “N” containing picolyl and Tröger's base moieties improve the utilization of TBNap for halogenated solvent sensing. The sensor TBNap showed a higher fluorescence enhancement in the CFM solvent compared to the other solvent medium. The enhancement behavior was validated using computational analysis. In addition to sensing, TBNap has also been used for the trace quantification of volatile solvents. The discriminative fluorescence sensing was also evident from the visual color changes, thereby upgrading the practical applicability of TBNap as a colorimetric probe. Further studies are in progress to develop a practically useful TBNap-based sensor system with improved selectivity, specificity, and sensitivity for detecting particular volatile solvents.
Author contributions
Binduja Mohan: data curation, formal analysis, investigation, methodology, software, validation, and writing – original draft. Deivasigamani Umadevi: computational investigation and validation. Sankarasekaran Shanmugaraju: conceptualization, funding acquisition, project administration, resources, supervision, validation, visualization, and writing – original draft.
Conflicts of interest
There are no conflicts to declare.
Acknowledgements
The authors thank the Science and Engineering Research Board (EMEQ Award EEQ/2018/000799 to SS) for the financial support. We thank the Central Instrumentation Facility (CIF) at IIT Palakkad for the research facilities.
References
- S. Shanmugaraju, D. Umadevi, L. M. González-Barcia, J. M. Delente, K. Byrne, W. Schmitt, G. W. Watson and T. Gunnlaugsson, Chem. Commun., 2019, 55, 12140 RSC.
- H. Liu, X. Xu, Z. Shi, K. Liu and Y. Fang, Anal. Chem., 2016, 88, 10167 CrossRef CAS.
-
L. Vittozzi and E. Testai, Handbook of Hazardous Materials, Academic Press, 1993, pp. 119–125 Search PubMed.
- R. F. Landis, M. Yazdani, B. Creran, X. Yu, V. Nandwana, G. Cooke and V. M. Rotello, Chem. Commun., 2014, 50, 4579 RSC.
- A. Bergen, C. Bohne, D. Fuentealba, H. Ihmels, T. Pace, M. Waidelich, C. Yihwa and J. W. Bats, Photochem. Photobiol. Sci., 2012, 11, 752 CrossRef CAS.
- C. Bohne, H. Ihmels, M. Waidelich and C. Yihwa, J. Am. Chem. Soc., 2005, 127, 17158 CrossRef CAS.
- L. Dai, D. Wu, Q. Qiao, W. Yin, J. Yin and Z. Xu, Chem. Commun., 2016, 52, 2095 RSC.
- R. Parui, N. Meher and P. K. Iyer, Mater. Adv., 2022, 3, 5980 RSC.
- R. Gao and D. Yan, Chem. Commun., 2017, 53, 5408 RSC.
- J. K. Wickliffe, T. H. Stock, J. L. Howard, E. Frahm, B. R. SimonFriedt, K. Montgomery, M. J. Wilson, M. Y. Lichtveld and E. Harville, Sci. Rep., 2020, 10, 21649 CrossRef CAS.
- B. Huang, C. Lei, C. Wei and G. Zeng, Environ. Int., 2014, 71, 118 CrossRef CAS PubMed.
- C. Barragán-Martínez, C. A. Speck-Hernández, G. Montoya-Ortiz, R. D. Mantilla, J.-M. Anaya and A. Rojas-Villarraga, PLoS One, 2012, 7, e51506 CrossRef PubMed.
- N. Guha, D. Loomis, Y. Grosse, B. Lauby-Secretan, F. E. Ghissassi, V. Bouvard, L. Benbrahim-Tallaa, R. Baan, H. Mattock and K. Straif, Lancet Oncol., 2012, 13, 1192 CrossRef PubMed.
- W. I. S. Galpothdeniya, B. P. Regmi, K. S. McCarter, S. L. de Rooy, N. Siraj and I. M. Warner, Anal. Chem., 2015, 87, 4464 CrossRef CAS PubMed.
- J. Lee, H. T. Chang, H. An, S. Ahn, J. Shim and J.-M. Kim, Nat. Commun., 2013, 4, 2461 CrossRef PubMed.
- W. R. Simpson, S. S. Brown, A. Saiz-Lopez, J. A. Thornton and R. V. Glasow, Chem. Rev., 2015, 115, 4035 CrossRef CAS PubMed.
- W. Buchberger and U. Huebauer, Microchim. Acta, 1989, 99, 137 CrossRef.
- M. I. Cervera, J. Beltran, F. J. Lopez and F. Hernandez, Anal. Chim. Acta, 2011, 704, 87 CrossRef CAS.
- R. Lu, W.-W. Li, B. Mizaikoff, A. Katzir, Y. Raichlin, G.-P. Sheng and H.-Q. Yu, Nat. Protoc., 2016, 11, 377 CrossRef CAS.
- X. Zhou, S. Lee, Z. Xu and J. Yoon, Chem. Rev., 2015, 115, 7944 CrossRef CAS.
- A. P. de Silva, H. Q. N. Gunaratne, T. Gunnlaugsson, A. J. M. Huxley, C. P. McCoy, J. T. Rademacher and T. E. Rice, Chem. Rev., 1997, 97, 1515 CrossRef CAS.
- S. Erbas-Cakmak, S. Kolemen, A. C. Sedgwick, T. Gunnlaugsson, T. D. James, J. Yoon and E. U. Akkaya, Chem. Soc. Rev., 2018, 47, 2228 RSC.
- G. Sivaraman, B. Vidya and D. Chellappa, RSC Adv., 2014, 4, 30828 RSC.
- T. Shen, D. Tan, M. Shanmugham and X. Liu, Sens. Diagn., 2022, 1, 714 RSC.
- S. Shanmugaraju and P. S. Mukherjee, Chem. Commun., 2015, 51, 16014 RSC.
- E. Yamaguchi, C. Wang, A. Fukazawa, M. Taki, Y. Sato, T. Sasaki, M. Ueda, N. Sasaki, T. Higashiyama and S. Yamaguchi, Angew. Chem., Int. Ed., 2015, 127, 4622 CrossRef.
- L. Giordano, V. V. Shvadchak, J. A. Fauerbach, E. A. Jares-Erijman and T. M. Jovin, J. Phys. Chem. Lett., 2012, 3, 1011 CrossRef CAS.
- S. Shanmugaraju, C. Dabadie, K. Byrne, A. J. Savyasachi, D. Umadevi, W. Schmitt, J. A. Kitchen and T. Gunnlaugsson, Chem. Sci., 2017, 8, 1535 RSC.
- R. F. Landis, M. Yazdani, B. Creran, X. Yu, V. Nandwana, G. Cooke and V. M. Rotello, Chem. Commun., 2014, 50, 4579 RSC.
- J. Yoon, Y. Jung and J. Kim, Adv. Funct. Mater., 2009, 19, 209 CrossRef CAS.
- E. B. Veale and T. Gunnlaugsson, J. Org. Chem., 2010, 75, 5513 CrossRef CAS.
- S. Shanmugaraju, C. S. Hawes, A. J. Savyasachi, S. Blasco, J. A. Kitchen and T. Gunnlaugsson, Chem. Commun., 2017, 53, 12512 CAS.
- M. Binduja, E.-A. Sandra, D. Umadevi, B. C. Poulsen, S. Blasco, G. J. McManus, T. Gunnlaugsson and S. Shanmugaraju, Inorg. Chem., 2022, 61, 11592 CrossRef PubMed.
- J. M. Delente, D. Umadevi, S. Shanmugaraju, O. Kotova, G. W. Watson and T. Gunnlaugsson, Chem. Commun., 2020, 56, 2562 RSC.
- J. I. Lovitt, D. Umadevi, P. Raja Lakshmi, B. Twamley, T. Gunnlaugsson and S. Shanmugaraju, Supramol. Chem., 2020, 32, 620–633 CrossRef CAS.
-
M. J. Frisch, G. W. Trucks, H. B. Schlegel, G. E. Scuseria, M. A. Robb, J. R. Cheeseman, G. Scalmani, V. Barone, G. A. Petersson, H. Nakatsuji, X. Li, M. Caricato, A. V. Marenich, J. Bloino, B. G. Janesko, R. Gomperts, B. Mennucci, H. P. Hratchian, J. V. Ortiz, A. F. Izmaylov, J. L. Sonnenberg, D. Williams-Young, F. Ding, F. Lipparini, F. Egidi, J. Goings, B. Peng, A. Petrone, T. Henderson, D. Ranasinghe, V. G. Zakrzewski, J. Gao, N. Rega, G. Zheng, W. Liang, M. Hada, M. Ehara, K. Toyota, R. Fukuda, J. Hasegawa, M. Ishida, T. Nakajima, Y. Honda, O. Kitao, H. Nakai, T. Vreven, K. Throssell, J. A. Montgomery, Jr., J. E. Peralta, F. Ogliaro, M. J. Bearpark, J. J. Heyd, E. N. Brothers, K. N. Kudin, V. N. Staroverov, T. A. Keith, R. Kobayashi, J. Normand, K. Raghavachari, A. P. Rendell, J. C. Burant, S. S. Iyengar, J. Tomasi, M. Cossi, J. M. Millam, M. Klene, C. Adamo, R. Cammi, J. W. Ochterski, R. L. Martin, K. Morokuma, O. Farkas, J. B. Foresman and D. J. Fox, Gaussian 16, Revision C.01, Gaussian, Inc., Wallingford CT, 2016 Search PubMed.
Footnotes |
† We dedicate this article to Prof. Thorfinnur Gunnlaugsson on the occasion of his 55th birthday. |
‡ Electronic supplementary information (ESI) available: FTIR, 13C NMR, and HRMS of TBNap. Electronic absorption and fluorescence emission spectral data of TBNap are available. See DOI: https://doi.org/10.1039/d2sd00177b |
|
This journal is © The Royal Society of Chemistry 2023 |
Click here to see how this site uses Cookies. View our privacy policy here.