DOI:
10.1039/D3SM00560G
(Paper)
Soft Matter, 2023,
19, 5880-5887
Tuning the crosslinking and degradation of hyaluronic acid/gelatin hydrogels using hydrogen peroxide for muscle cell sheet fabrication†
Received
28th April 2023
, Accepted 5th July 2023
First published on 5th July 2023
Abstract
Cell sheets have immense potential for medical and pharmaceutical applications including tissue regeneration, drug testing, and disease modelling. In this study, composite hydrogels were prepared from a mixture of phenolated hyaluronic acid (HA-Ph) and gelatin (Gelatin-Ph), with a controlled degree of polymer crosslinking and degradation, to fabricate muscle cell sheets from myoblasts. These hydrogels were obtained via hydrogen peroxide (H2O2)-mediated crosslinking catalysed by horseradish peroxidase (HRP) and peroxide-mediated cleavage of the polymer chains. The degrees of crosslinking and degradation were modulated by altering the exposure time to air containing H2O2. The results showed that exposing a solution of 2% w/v HA-Ph, 0.75% w/v Gelatin-Ph, and 1 unit mL−1 HRP to air with 16 ppm H2O2 for 60 min yielded a stiffer hydrogel (7.16 kPa Young's modulus) than exposure times of 15 min (0.46 kPa) and 120 min (3.98 kPa). Moreover, mouse myoblast C2C12 cells cultured on a stiff hydrogel and induced to undergo myogenic differentiation formed longer and higher-density myotubes than those on softer hydrogels. The cell sheets were readily detached within 5 min by immersing the HA-Ph/Gelatin-Ph hydrogels covered with a monolayer of cells in a medium containing hyaluronidase. Our findings demonstrate that composite hydrogels with properties tuned by controlling the exposure time to H2O2, show great promise as platforms for muscle cell sheet fabrication.
Introduction
Cell sheet technology is a strategy for fabricating sheets composed of cells with cell-to-cell connections in vitro. The cell sheets can be transplanted into patients to repair damaged tissues or organs, such as the cornea,1 myocardium,2 cartilage,3 and skeletal muscles,4 and they can be used in disease models in drug testing.5 The most common approach for fabricating cell sheets involves growing cells on temperature-responsive surfaces that are modified with poly(N-isopropyl acrylamide) (PNIPAAm). The cells are harvested by decreasing the temperature to below 32 °C,6 which induces a phase transition between PNIPAAm's hydrophilic and hydrophobic states. These transitions occur around 32 °C; therefore, decreasing the temperature below this threshold facilitates cell harvest.6 While temperature-responsive dishes have been widely used, they have limitations, such as cell type dependent detachment and difficulties in cell adhesion and growth.7 Moreover, the surface properties of temperature-responsive dishes, such as stiffness, surface charge, and surface chemistry, which are critical for controlling cell behaviour including functional expression and differentiation, are difficult to tune.8,9
The objective of this study was to address some of the limitations of commonly used temperature-responsive substrates for cell sheet fabrication. Specifically, we aimed to develop hydrogels that could influence the myogenic differentiation of myoblasts into skeletal muscle cells and allow for easy harvesting of cell sheets without temperature control. To achieve this, a hydrogel was prepared from a mixture of phenolated hyaluronic acid (HA-Ph) and type B gelatin (Gelatin-Ph) via horseradish peroxidase (HRP)-mediated crosslinking of the phenol moieties (Fig. 1a) using hydrogen peroxide (H2O2) as an electron acceptor. H2O2-mediated hydrogelation has been applied to create hydrogels from various polymer solutions for diverse biomedical applications.10–14 Hydrogels are water-swollen networks of polymer chains that are commonly used for in vitro cell culture applications owing to their high biocompatibility, degradability, and similarity to the natural extracellular matrix (ECM).15–18 Hydrogels can be designed with various physical, chemical, and biological properties, such as stiffness, porosity, and specific moieties that interact with cell surface receptors.19,20 Previous studies have reported the successful differentiation of mouse myoblast C2C12 cells into myotubes on substrates with muscle tissue-like stiffness21 and have effectively controlled hydrogel stiffness for the proliferation and differentiation of C2C12 cells and human myoblasts.22
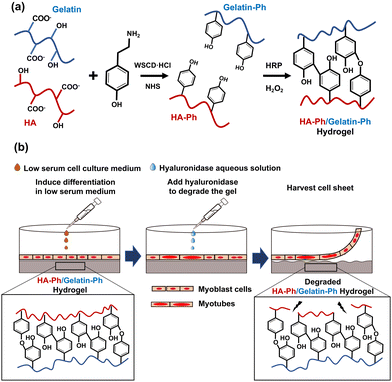 |
| Fig. 1 Schematics of (a) synthesis of HA-Ph and Gelatin-Ph using WSCD·HCl and NHS, followed by HRP-mediated crosslinking of these polymers utilising hydrogen peroxide (H2O2) to yield the hydrogel, and (b) after inducing myogenic differentiation on the hydrogel, the cell sheet is harvested by treating the HA-Ph/Gelatin-Ph hydrogel with hyaluronidase. | |
Hyaluronic acid (HA) is a biocompatible polysaccharide abundant in the ECM and is known to regulate skeletal muscle cell functions,23 and myogenic differentiation.24 HA interacts with the receptors present on the cell surface, i.e., CD44 and receptor for HA-mediated motility (RHAMM).25 However, HA alone does not support cell adhesion; therefore, it must be combined with materials that promote cell adhesion. In a preliminary study, C2C12 cells did not adhere to or grow on hydrogels made of HA-Ph alone (Fig. S1, ESI†). Consequently, in this study, the composite hydrogels prepared from a mixture of HA-Ph and Gelatin-Ph were tested. Gelatin is known to promote cell adhesion because it possesses an arginyl-glycyl-aspartic acid (RGD) peptide, which allows for cell attachment.26 Gelatin is also an attractive choice owing to its biocompatibility, biodegradability, and ability to mimic some aspects of the ECM properties, making it a suitable component for composite hydrogels in tissue engineering applications.14
In this study, the crosslinking density and degradation of these polymers were controlled by changing the H2O2 exposure time during hydrogel preparation. We have reported that H2O2 functions not only as an essential substrate for HRP-catalysed crosslinking of phenolic hydroxyl moieties but also degrades polymer chains in the hydrogels.27–29 Our previous study demonstrated that C2C12 cell attachment and myogenic differentiation could be influenced by adjusting the degree of crosslinking and degradation of Gelatin-Ph molecules in hydrogels prepared from a solution containing Gelatin-Ph alone via H2O2-mediated reactions.28
The hypothesis for this study was that HA-Ph/Gelatin-Ph composite hydrogels with varying degrees of H2O2-mediated crosslinking and degradation would facilitate control over myogenic differentiation of myoblasts on the hydrogels and allow cell sheet harvesting using hyaluronidase (Fig. 1b). The enzyme hyaluronidase degrades hyaluronic acid without the need for temperature control, making it an attractive option for cell sheet preparation.30 In this study, mouse myoblast C2C12 cells, a frequently used myoblast model,31,32 were used to test the potential of HA-Ph/Gelatin-Ph composite hydrogels as platforms for muscle cell sheet fabrication.
Materials and methods
Materials
HA-Ph, which contains eight Ph groups per 100 repeat units of HA, was supplied by Nagase ChemteX (Osaka, Japan). Gelatin-Ph (4.1 × 10−4 mol-Ph per g-Gelatin-Ph) was synthesized following a previously reported method,33,34 by conjugating type B gelatin (bovine skin, 226 g Bloom, Sigma-Aldrich, St. Louis, MO, USA) with 3-(4-hydroxyphenyl) propionic acid (HPPA; Tokyo Chemical Industry, Tokyo, Japan) using water-soluble carbodiimide hydrochloride (WSCD·HCl; Peptide Institute, Osaka, Japan) and N-hydroxysuccinimide (NHS; FUJIFILM, Wako Pure Chemical, Osaka, Japan). Aqueous H2O2 (31% w/w), catalase from bovine liver (8000 U mg−1), 4% w/w paraformaldehyde in phosphate-buffered saline (PBS, pH 7.4), collagenase (203 U mg−1), hyaluronidase (890 U mg−1), and HRP (190 U mg−1) were purchased from FUJIFILM Wako Pure Chemical. Propidium iodide (PI) and 4′6-diamidino-2-phenylindole (DAPI) were purchased from Dojindo (Kumamoto, Japan). Calcein-AM and phalloidin-iFluor 647 (ab176759) were purchased from Nacalai Tesque Inc. (Kyoto, Japan) and Abcam (Cambridge, UK), respectively. Dulbecco's modified eagle medium (DMEM) from Nissui (Tokyo, Japan) was used for cell culture. The full list of abbreviations can be found in the footnote‡.
Cell culture
Mouse myoblast C2C12 cells (Riken Bio-Resource Center, Ibaraki, Japan) were cultured in DMEM with 10% v/v FBS in a humidified incubator with 95% air/5% CO2 at 37 °C.
Molecular weight measurement
HA-Ph was dissolved in PBS at 2% w/v, and the polymer solution was exposed to 16 ppm H2O2 in air for 15–120 min. The resulting HA-Ph solutions were analysed using HPLC (LC-20AD, Shimadzu, Kyoto, Japan). Unexposed polymer solutions were used as controls. A calibration curve for the molecular weight determination was prepared using the Pullulan standard.
Composite hydrogel (HA-Ph/Gelatin-Ph) preparation
Hydrogels were prepared from 2% w/v HA-Ph, 0.75% w/v Gelatin-Ph, and 1 U mL−1 HRP dissolved in PBS by varying the exposure time to 16 ppm H2O2 in air from 15 to 120 min.
Measurement of mechanical properties
HA-Ph/Gelatin-Ph hydrogels with a diameter of 22.1 mm and a height of 4 mm were prepared by pouring 1 mL of the polymer solution containing HA-Ph, Gelatin-Ph, and 1 U mL−1 HRP into a 12-well plate followed by exposure to H2O2-containing air. Young's moduli of the prepared hydrogels were determined utilising a material tester (EZ-SX, Shimadzu, Kyoto, Japan) working at a compressing rate of 6.0 mm min−1 with a 5 mm diameter probe at 37 °C. The stress-strain curve in the linear range of 2–8% compression was used to calculate the values. For each hydrogel, three measurements were performed, and the average value was used in the calculations.
Enzymatic degradation
The degradability of the HA-Ph/Gelatin-Ph hydrogels by hyaluronidase was evaluated by preparing three hydrogels for each condition (8 mm in diameter and 2 mm in height). The hydrogels were immersed in 1 mL DMEM containing 0.1% w/v hyaluronidase at 37 °C, and the morphological changes of the hydrogels were observed for 2 h. The degradation time reported in the results is the average of all three hydrogels under each condition.
Cell adhesion and viability analysis
The hydrogels were formed in the wells of a 6-well plate. Before cell seeding, residual H2O2 was removed from the hydrogels by overnight immersion in 1 mL of DMEM containing 0.1% w/v catalase and thorough washing in PBS thrice. After replacing the medium with fresh DMEM, the C2C12 cells were cultured at an initial density of 5 × 103 cells per well. Two days post seeding, the cells were stained with Calcein-AM for further analysis. The adhesion area and aspect ratios of individual cells were determined from micrographs (six random positions on each hydrogel) obtained using a fluorescence microscope (BZ-9000, Keyence, Osaka, Japan) and ImageJ software (2.1.0/1.53c, NIH, USA). The aspect ratio was calculated as the ratio of the major and minor axes of the fitted ellipses. The cell circularity was calculated using the formula 4π × (area/perimeter2).
C2C12 cell differentiation
Cells were cultured on the hydrogels at an initial density of 1 × 105 cells per well in DMEM supplemented with 10% v/v FBS. Upon reaching confluence, the cells were cultured in a differentiation medium (DMEM containing 2% v/v horse serum) following daily replenishment of the medium to support cell growth. After culturing for six days in the differentiation medium, the cells were fixed by immersion in 4% paraformaldehyde in PBS for 30 min and permeabilised with 50 mM 4-(2-hydroxyethyl)-1-piperazineethanesulfonic acid (HEPES)-buffered solution containing bovine serum albumin (pH 7.4). The cells were then stained with phalloidin-iFluor 647 (F-actin) and DAPI (nucleus). The number of cells that differentiated into multinucleated myotubes and the length of the myotubes were determined from fluorescent micrographs taken at six random positions by fluorescence microscopy using ImageJ software.
Cell sheet fabrication
Once confluence was reached, hyaluronidase was added to the medium at a final concentration of 0.1% w/v. After 5 min, cell sheet detachment was examined using an inverted microscope (IX71; Olympus, Tokyo, Japan). The detached cell sheets were transferred to fresh 6-well plates using a pipette to assess the ability of the cell sheets to reattach to other substrates.
Statistical analysis
Microsoft Excel 2019, version 1808 (Microsoft Corp., Redmond, WA, USA) was used for data analysis. One-way analysis of variance (ANOVA) was used for statistical analyses. A post-hoc t-test was conducted using Tukey's HSD, with a p-value of <0.05 considered significantly different.
Results
Characterisation of the composite hydrogels
First, the mechanical properties of the composite hydrogels composed of 2% w/v HA-Ph and 0.75% w/v Gelatin-Ph prepared by exposure to H2O2-containing air for 15, 60, and 120 min was assessed. As depicted in Fig. 2a, increasing the exposure time from 15 to 60 min led to a significant increase in the Young's modulus from 0.5 ± 0.1 to 7.1 ± 0.2 kPa. However, increasing the exposure time further, from 60 to 120 min, caused a decrease in the Young's modulus from 7.1 ± 0.2 to 3.9 ± 0.1 kPa. We also investigated the impact of the H2O2-exposure time on the hyaluronidase-mediated degradation of the hydrogels in a cell culture medium containing hyaluronidase at a final concentration of 0.1% w/v. As shown in Fig. 2b, the 15 min-H2O2-exposure-hydrogels were completely degraded within 48 ± 4 min. The time required for degradation increased to 115 ± 7 min with an increase in the H2O2-exposure time to 60 min. A further increase in the H2O2-exposure time to 120 min led to a decrease in the time required for degradation of the hydrogels to 83 ± 4 min.
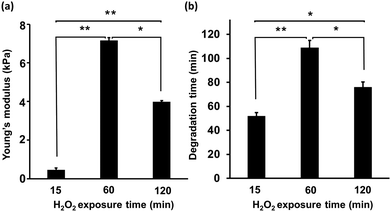 |
| Fig. 2 Effect of H2O2 exposure time during hydrogel preparation on (a) Young's modulus of the composite hydrogels (n = 3), and (b) the time until complete degradation of the composite hydrogels in medium containing 0.1% w/v hyaluronidases (n = 5). Error bars in columns: S.D. *p < 0.05, **p < 0.01, Tukey HSD. | |
Cell adhesion and hydrogel morphology
Initially, the suitability of the HA-Ph/Gelatin-Ph hydrogels for the C2C12 cells was confirmed by measuring the cell viability the day after seeding; the viabilities of cells grown on HA-Ph/Gelatin-Ph hydrogels prepared at 15, 60, and 120 min of H2O2 exposure were 89%, 96%, and 95%, respectively. These results demonstrate the limited toxicity of H2O2 in this hydrogelation system. Although H2O2 is known to exert cytotoxic effects on cells, overnight incubation with catalase appears to be sufficient to degrade the remaining H2O2. As shown in Fig. 3a and b, the cells cultured on the stiff hydrogel, obtained after 60 min of exposure to H2O2-air, showed a 2.8 and 1.5-fold increase in area compared to those cultured on the hydrogels obtained after 15 and 120 min of exposure, respectively. This was 73% of the area of those grown on cell culture dishes. In addition, cells on the hydrogel prepared with 60 min of exposure showed the highest elongation, marked by the highest aspect ratio (Fig. 3c) and the lowest circularity (Fig. 3d), compared to those on other hydrogels and culture dishes.
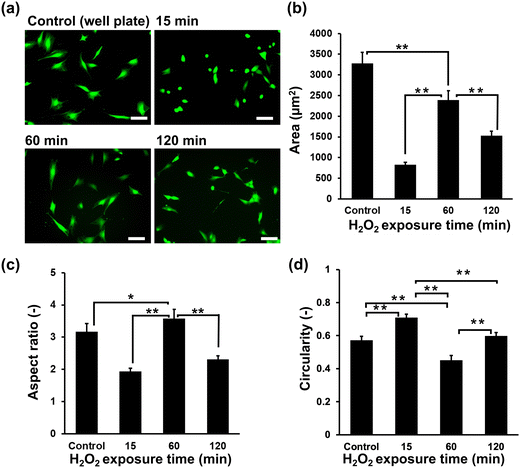 |
| Fig. 3 Effect of H2O2 exposure time during hydrogel preparation on C2C12 cell morphology after two days of culture. (a) Fluorescence micrographs of C2C12 cells on hydrogels stained with Calcein-AM. Scale bars: 100 μm. (b) Area, (c) aspect ratio, and (d) circularity of cells on hydrogels. Error bars in columns: S.D (n ≥ 50). (a)–(d) Control: On 6-well plate. *p < 0.05, **p < 0.01, Tukey HSD. | |
Cell differentiation on hydrogels
The myogenic differentiation of C2C12 cells on HA-Ph/Gelatin-Ph hydrogels prepared by exposure to H2O2-containing air for 15 to 120 min was analysed after six days of culture in differentiation medium. Fig. 4a shows cells stained with phalloidin-iFluor 647 (red: F-actin) and DAPI (blue: nucleus). Comparing the density of myotubes with multinucleate in the cells, those grown on the hydrogels obtained through 60 min exposure time showed more than two times higher densities than those grown on the other hydrogels (Fig. 4a and b). In addition, the cells on the 60 min-hydrogel had the longest myotubes (Fig. 4c). Both, myotube density and length were 1.7-fold and 2.9-fold greater in cells grown on the 60 min-hydrogel than in those cultured on cell culture dishes, respectively.
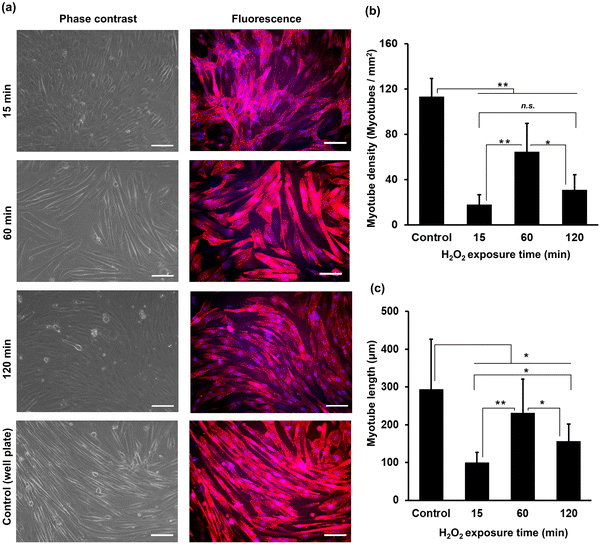 |
| Fig. 4 Effect of the H2O2 exposure time during hydrogel preparation on myogenic differentiation of C2C12 cells. The studies were conducted after six days of culture in a differentiation medium. (a) Representative phase contrast and fluorescence micrographs of C2C12 cells stained with phalloidin-iFluor 647 (red: F-actin) and DAPI (blue: nucleus), respectively. Scale bars: 100 μm. (b) The number of myotubes and (c) myotube length. Error bars in columns: S.D. (n ≥ 50). (a)–(c) Control: On 6-well plate. *p < 0.05, **p < 0.01, n.s.: p > 0.05, Tukey HSD. | |
Cell sheet harvesting
To harvest the cell sheets, hyaluronidase was added to the medium at a final concentration of 0.1% w/v after the induction of myogenic differentiation. As shown in Fig. 5a–c, the cells detached from the hydrogels after 5 min of hyaluronidase addition, while preserving cell-to-cell connections; that is, the cells detached as cell sheets. In contrast, the cells detached by collagenase treatment lost their cell-to-cell connections and broke into small pieces (Fig. 5h). In addition, the cells did not detach from the hydrogel composed solely of Gelatin-Ph upon hyaluronidase addition to the medium (Fig. 5g). These results indicate that the detachment of cell sheets from the HA-Ph/Gelatin-Ph hydrogels was caused by the degradation of HA-Ph by hyaluronidase. All detached cell sheets were re-adhered to the cell culture dishes within 2 h (Fig. 5d–f).
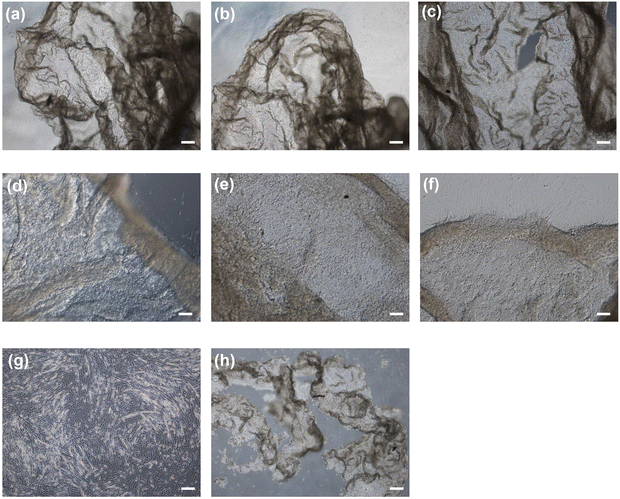 |
| Fig. 5 Photomicrographs of detached cell sheets by adding 0.1 w/v% hyaluronidase to the hydrogel prepared through (a) 15, (b) 60, and (c) 120 min of exposure to air containing H2O2, and (d)–(f) re-attachment of the cell sheets to wells of a 6-well plate, respectively. Photomicrographs of the cells on hydrogel prepared from the solution containing 2 w/v% Gelatin-Ph through 60 min of exposure to air containing H2O2 after soaking in medium containing (g) 0.1 w/v% hyaluronidase, and (h) 0.1 w/v% collagenase for 5 min. Scale bars: 100 μm. | |
Discussion
The primary objective of this study was to assess the potential of hyaluronidase-degradable HA-Ph/Gelatin-Ph hydrogels as platforms for cell sheets production from myoblasts. These HA-composite hydrogels present advantages over conventionally used temperature-responsive PNIPAAm surfaces, such as the ability to easily control polymer content and modulate crosslinking density and degradation, which in turn influence hydrogel stiffness and interaction with cell surface receptors. The selected HA-Ph (2% w/v) and Gelatin-Ph (0.75% w/v) concentrations were based on preliminary solution viscosity studies to ensure ease of handling and complete hydrogel degradation by hyaluronidase. Solutions containing more than 2% w/v HA-Ph were too viscous for pipetting and hydrogels with a Gelatin-Ph content higher than 0.75% w/v required both hyaluronidase and protease for complete degradation. The chosen HRP concentration (1 U mL−1) facilitated the investigation of the correlations between HRP-catalysed crosslink formation and polymer chain degradation by H2O2.27–29,35
To modulate polymer crosslinking and degradation in the hydrogels with H2O2, we varied the exposure times of the HA-Ph, Gelatin-Ph, and HRP mixtures to air containing 16 ppm H2O2. The measurement of the mechanical properties has direct implications for the contradictory effects of H2O2. The changes observed in the Young's modulus with increasing exposure time to H2O2-containing air (Fig. 2a) can be attributed to alterations in the phenol moiety crosslinking and polymer chain degradation. These trends are consistent with our previous findings,27,29 despite the different polymer contents of the hydrogels. The degradation of polymers by oxidative cleavage by H2O2 is well known36 and H2O2-induced degradation of Gelatin-Ph28,35 and HA-Ph has been reported.27,29 Those observations were confirmed in this study (Fig. S2, ESI†). The shorter degradation time of the less-rigid hydrogels (Fig. 2a and b) may be attributed to the looser polymer network, which allows easier hyaluronidase penetration. Although we used air with 16 ppm H2O2, higher concentrations could potentially reduce the time required to achieve comparable crosslinking and degradation. Although not investigated in this study, hydrogel properties could be controlled by altering the H2O2 concentration. HA-Ph, Gelatin-Ph, and HRP concentrations also affect the hydrogel properties and should be considered.
The observed trend of larger cell area and aspect ratio for C2C12 cells on stiffer HA-Ph/Gelatin-Ph composite hydrogels (Fig. 3a and b) is consistent with our previous results on C2C12 cells on Gelatin-Ph hydrogels.28 Similar stiffness-dependent changes in myoblast morphology have been reported for other substrates.21 Stiff substrates have been shown to facilitate actin polymerisation and assembly, leading to cell elongation and cytoskeletal tension maintenance.37 In contrast, softer substrates hinder F-actin bundle and stress fibre formation, resulting in smaller cell area, higher circularity and aspect ratio values.27,38,39 Additionally, low molecular weight HA-Ph generated through the cleavage of the polymer chain by H2O2 might also affected the morphology of C2C12 cells. It has been reported that the interaction between low molecular weight HA and CD44 and the RHAMM receptor triggered a signalling pathway cascade that alters cell behaviour.40,41
Differences in myotube formation among C2C12 cells on hydrogels with varying stiffness can also be attributed to the hydrogel stiffness, as the highest myotube formation with the largest length was observed on the stiffest hydrogel fabricated at 60 min of H2O2 exposure (Fig. 4). Several studies have reported higher myoblast differentiation on stiffer hydrogels.22,42 Stiffer substrates can better withstand the forces generated by contracting cells, thereby allowing for improved myotube formation.43 A possible explanation for the longer myotube formation in stiffer hydrogels is that there is a correlation between stiffness and adhesion properties of myoblasts. This can be explained by the fact that ECM stiffness affects the tension and force generated by cells. On a soft substrate, cells generate less tension because of the lack of resistance, resulting in shorter myotubes. In contrast, on a stiff substrate, cells generate more tension, resulting in longer myotubes. A previous study by Bettadapur et al. (2016) showed the effect of substrate stiffness on myotube length on a gelatin hydrogels, in which longer myotubes were formed on stiffer substrates compared to softer substrates.44 The low myotube formation observed on the hydrogel fabricated at 15 min of H2O2 exposure could be due to substrate deformation or collapse under cell contraction forces, inhibiting myotube formation.43 Interestingly, lower myotube formation was observed in cells cultured on hydrogel fabricated by 120 min of H2O2 exposure (Fig. 4). This phenomenon may have been caused by the presence of low molecular weight HA-Ph fragments resulting from prolonged H2O2 exposure (Fig. S2, ESI†). Earlier investigations have found that the molecular weight of HA has varying effects on muscle cell differentiation, with low molecular weight HA exhibiting an inhibitory effect.45 Low molecular weight HA-Ph fragments may interact with HA cell receptors (CD44 and RHAMM), leading to the inhibition of myoblast behaviour, including myogenesis.25 Indeed, HA binding to cells is reported to inhibit myogenesis.24 Overall, the complex interplay between substrate stiffness and molecular weight plays a role in C2C12 differentiation.
In this study, cell sheets capable of reattaching to the substrates were successfully harvested by treatment with hyaluronidase (Fig. 5). All cell sheets detached within 5 min and there were no notable differences depending on exposure time to air containing H2O2 for cell detachment, despite significant differences in the time necessary for complete hydrogel degradation (Fig. 2b). This is because cell sheet detachment resulted from the degradation of the hydrogel surface in contact with the cells, indicating that the crosslinking density of the polymers had little effect on the time required for complete degradation. There are few safety concerns for the use of hyaluronidase to harvest cell sheets for clinical applications, because hyaluronidase is already used in clinical applications, including ophthalmic and dermatologic surgery.46 Using alginate and cellulose derivatives instead of HA-Ph is another option. Cell sheets have been obtained by treatment with alginate lyase47,48 and cellulase30 without using protease. However, to the best of our knowledge, these enzymes have not been applied clinically, making hyaluronidase-degradable substrates more attractive than those degradable by alginate lyase or cellulase.
As shown in Fig. 4, the degree of myogenic differentiation and the cell shape within each cell sheet were not uniform. Cell sheet functionality beyond the reattachment ability was not investigated in this study. The reattached cell sheets proliferated on the new surface without detachment, demonstrating the long-term viability of the cell sheets as reported in the previous studies that obtained cell sheet using other substrate such as PNIPAAm.6 The functions required for cell sheets vary depending on their applications in tissue regeneration, drug testing, and disease modelling. The crucial finding of this study was the potential to fabricate cell sheets from myoblasts with different qualities using solutions containing HA-Ph, Gelatin-Ph, and HRP by controlling the exposure time to air containing H2O2. Further studies on the detailed functionality of cell sheets will be conducted in the future, considering their applications for specific purposes.
Conclusions
In this study, we demonstrated the potential of HA-Ph/Gelatin-Ph hydrogels as platforms for muscle cell sheet fabrication by modulating their physicochemical properties through control of the H2O2 exposure time. Our findings show that stiffer hydrogels facilitated a higher density of myotube formation, whereas softer and intermediately stiff hydrogels led to a lower density of myotube formation. Furthermore, we successfully harvested cell sheets with different myotube densities within 5 min of hyaluronidase addition. The harvested cell sheets maintained their morphology and were reattached to the cell culture well plates within 2 h. These results demonstrate the versatility and potential of HA-Ph/Gelatin-Ph hydrogels obtained by controlling H2O2-mediated crosslinking and degradation for the fabrication of muscle cell sheets from myoblasts. Continued investigation and optimisation of this platform will be crucial to unlock its full potential for advancing the fields of tissue engineering, regenerative medicine, and disease modelling.
Author contributions
K. C. M. L. E.: conceptualisation, methodology, investigation, data curation, formal analysis, writing – original draft, writing – review & editing, and visualisation. W. M.: investigation, writing, review & editing. S. S.: conceptualisation, methodology, resources, funding acquisition, writing – original draft, writing – review & editing, supervision. All the authors approved the final version of the manuscript.
Conflicts of interest
There are no conflicts to declare.
Acknowledgements
This study was supported by Toyota Physical and Chemical Research Institute, and the Japan Society for the Promotion of Science (JSPS) Fostering Joint International Research (B) [grant number 20KK0112] and a Grant-in-Aid for JSPS Fellows No. 22F22373. W. M. was supported by a JSPS Postdoctoral Fellowship in Japan. We would like to thank Editage (http://www.editage.com) for English language editing.
References
- K. Nishida, M. Yamato, Y. Hayashida, K. Watanabe, K. Yamamoto, E. Adachi, S. Nagai, A. Kikuchi, N. Maeda, H. Watanabe, T. Okano and Y. Tano, N. Engl. J. Med., 2004, 351, 1187–1196 CrossRef CAS PubMed.
- Y. Sawa, Y. Yoshikawa, K. Toda, S. Fukushima, K. Yamazaki, M. Ono, Y. Sakata, N. Hagiwara, K. Kinugawa and S. Miyagawa, Circ. J., 2015, 79, 991–999 CrossRef.
- M. Sato, M. Yamato, K. Hamahashi, T. Okano and J. Mochida, Anat. Rec., 2014, 297, 36–43 CrossRef CAS PubMed.
- P. Thummarati and M. Kino-oka, Front. Bioeng. Biotechnol., 2020, 8, 578140 CrossRef PubMed.
- J. Lee, D. Shin and J. L. Roh, Theranostics, 2018, 8, 3964–3973 CrossRef CAS PubMed.
- Z. Tang, Y. Akiyama and T. Okano, J. Polym. Sci., Part B: Polym. Phys., 2014, 52, 917–926 CrossRef CAS.
- N. Yamada, T. Okano, H. Sakai, F. Karikusa, Y. Sawasaki and Y. Sakurai, Makromol. Chem., Rapid Commun., 1990, 11, 571–576 CrossRef CAS.
- Y. Arisaka, J. Kobayashi, K. Ohashi, K. Tatsumi, K. Kim, Y. Akiyama, M. Yamato and T. Okano, Regener. Ther., 2016, 3, 97–106 CrossRef.
- K. Kieswetter, Z. Schwartz, D. D. Dean and B. D. Boyan, Crit. Rev. Oral Biol. Med., 1996, 7, 329–345 CrossRef CAS PubMed.
- S. Sakai, Y. Liu, T. Matsuyama, K. Kawakami and M. Taya, J. Mater. Chem., 2012, 22, 1944–1949 RSC.
- S. Sakai, Y. Yamamoto, G. Enkhtuul, K. Ueda, K. Arai, M. Taya and M. Nakamura, Macromol. Biosci., 2017, 17, 1600416 CrossRef PubMed.
- S. Sakai and M. Nakahata, Chem. – Asian J., 2017, 12, 3098–3109 CrossRef CAS PubMed.
- X. Chen, H. Zhang, J. Cui, Y. Wang, M. Li, J. Zhang, C. Wang, Z. Liu and Q. Wei, Gels, 2023, 9, 155 CrossRef CAS.
- M. Li, D. Sun, J. Zhang, Y. Wang, Q. Wei and Y. Wang, Biomater. Sci., 2022, 10, 5430–5458 RSC.
- P. Zhao, Z. Guo, H. Wang, B. Zhou, F. Huang, S. Dong, J. Yang, B. Li and X. Wang, Biomater. Adv., 2023, 152, 213481 CrossRef CAS.
- H. Cao, L. Duan, Y. Zhang, J. Cao and K. Zhang, Signal Transduction Targeted Ther., 2021, 6, 1–31 CrossRef PubMed.
- Y. Yang, K. Yu, F. Xing, H. Lai, K. Shi, Y. Zhou and P. Xiao, Adv. Healthcare Mater., 2022, 11, 2101504 CrossRef CAS.
- J. Ciriza, A. Rodríguez-Romano, I. Nogueroles, G. Gallego-Ferrer, R. M. Cabezuelo, J. L. Pedraz and P. Rico, Mater. Sci. Eng., C, 2021, 123, 112003 CrossRef CAS PubMed.
- K. Vats, G. Marsh, K. Harding, L. Zampetakis, R. E. Waugh and D. S. W. Benoit, J. Biomed. Mater. Res., Part A, 2017, 105, 1112–1122 CrossRef CAS PubMed.
- F. Brandl, F. Sommer and A. Goepferich, Biomaterials, 2007, 28, 134–146 CrossRef CAS PubMed.
- A. J. Engler, M. A. Griffin, S. Sen, C. G. Bönnemann, H. L. Sweeney and D. E. Discher, J. Cell Biol., 2004, 166, 877–887 CrossRef CAS PubMed.
- J. Tomasch, B. Maleiner, P. Heher, M. Rufin, O. G. Andriotis, P. J. Thurner, H. Redl, C. Fuchs and A. H. Teuschl-Woller, Front. Bioeng. Biotechnol., 2022, 10, 836520 CrossRef PubMed.
- J. M. Silva Garcia, A. Panitch and S. Calve, Acta Biomater., 2019, 84, 169–179 CrossRef CAS PubMed.
- M. J. Kujawa, D. G. Pechak, M. Y. Fiszman and A. I. Caplan, Dev. Biol., 1986, 113, 10–16 CrossRef CAS PubMed.
- Y. Leng, A. Abdullah, M. K. Wendt and S. Calve, Matrix Biol., 2019, 78–79, 236 CrossRef CAS.
- H. Tian, L. Lin, J. Chen, X. Chen, T. G. Park and A. Maruyama, J. Controlled Release, 2011, 155, 47–53 CrossRef CAS PubMed.
- W. Mubarok, K. C. M. L. Elvitigala, M. Nakahata, M. Kojima and S. Sakai, Cells, 2022, 11, 881 CrossRef CAS PubMed.
- W. Mubarok, K. C. M. L. Elvitigala and S. Sakai, Gels, 2022, 8, 387 CrossRef CAS PubMed.
- K. C. M. L. Elvitigala, W. Mubarok and S. Sakai, Polymers, 2022, 14, 5034 CrossRef CAS PubMed.
- S. Sakai, Y. Ogushi and K. Kawakami, Acta Biomater., 2009, 5, 554–559 CrossRef CAS PubMed.
- L. T. Denes, L. A. Riley, J. R. Mijares, J. D. Arboleda, K. McKee, K. A. Esser and E. T. Wang, Skeletal Muscle, 2019, 9, 17 CrossRef PubMed.
- M. Kodaka, Z. Yang, K. Nakagawa, J. Maruyama, X. Xu, A. Sarkar, A. Ichimura, Y. Nasu, T. Ozawa, H. Iwasa, M. Ishigami-Yuasa, S. Ito, H. Kagechika and Y. Hata, Exp. Cell Res., 2015, 336, 171–181 CrossRef CAS PubMed.
- S. Sakai, K. Hirose, K. Taguchi, Y. Ogushi and K. Kawakami, Biomaterials, 2009, 30, 3371–3377 CrossRef CAS PubMed.
- M. Khanmohammadi, S. Sakai and M. Taya, Int. J. Biol. Macromol., 2017, 97, 308–316 CrossRef CAS PubMed.
- W. Mubarok, Y. Qu and S. Sakai, ACS Appl. Bio Mater., 2021, 4, 4184–4190 CrossRef CAS PubMed.
- Z. Ma, Y. Wu, Y. He and T. Wu, RSC Adv., 2013, 3, 12049–12051 RSC.
- S. Walcott and S. X. Sun, Proc. Natl. Acad. Sci. U. S. A., 2010, 107, 7757–7762 CrossRef CAS PubMed.
- J. T. Parsons, A. R. Horwitz and M. A. Schwartz, Nat. Rev. Mol. Cell Biol., 2010, 11, 633–643 CrossRef CAS PubMed.
- T. Iskratsch, H. Wolfenson and M. P. Sheetz, Nat. Rev. Mol. Cell Biol., 2014, 15, 825–833 CrossRef CAS PubMed.
- S. Misra, V. C. Hascall, R. R. Markwald and S. Ghatak, Front. Immunol., 2015, 6, 137534 Search PubMed.
- K. J. Kim, O. H. Lee and B. Y. Lee, Br. J. Nutr., 2011, 106, 1836–1844 CrossRef CAS PubMed.
- K. J. M. Boonen, K. Y. Rosaria-Chak, F. P. T. Baaijens, D. W. J. Van Der Schaft and M. J. Post, Am. J. Physiol.: Cell Physiol., 2009, 296, 1338–1345 CrossRef.
- M. Levy-Mishali, J. Zoldan and S. Levenberg, Tissue Eng., Part A, 2009, 15, 935–944 CrossRef CAS PubMed.
- A. Bettadapur, G. C. Suh, N. A. Geisse, E. R. Wang, C. Hua, H. A. Huber, A. A. Viscio, J. Y. Kim, J. B. Strickland and M. L. McCain, Science, 2016, 6, 1–14 Search PubMed.
- L. C. Hunt, C. Gorman, C. Kintakas, D. R. Mcculloch, E. J. Mackie and J. D. White, J. Biol. Chem., 2013, 288, 13006–13021 CrossRef CAS PubMed.
- B. A. Buhren, H. Schrumpf, N. P. Hoff, E. Bölke, S. Hilton and P. A. Gerber, Eur. J. Med. Res., 2016, 21, 5 CrossRef PubMed.
- S. Sakai, T. Matsuyama, K. Hirose and K. Kawakami, Biomacromolecules, 2010, 11, 1370–1375 CrossRef CAS PubMed.
- Y. Liu, S. Sakai and M. Taya, Acta Biomater., 2013, 9, 6616–6623 CrossRef CAS.
Footnotes |
† Electronic supplementary information (ESI) available: Fig. S1: Fluorescence micrographs of C2C12 cells after 2 days of culture on HA-Ph hydrogels obtained by exposing PBS containing 2% w/v HA-Ph and 1 U mL−1 HRP to air containing H2O2 for 15–120 min. Fig. S2: Intensity–time curve (a) and average molecular weight of HA-Ph (b) after exposure to 16 ppm H2O2 supplied from air for 0–120 min. See DOI: https://doi.org/10.1039/d3sm00560g |
‡ List of abbreviations. DMEM, Dulbecco's Modified Eagle Medium; FBS, Fetal bovine serum; HA, Hyaluronic acid; HEPES, 4-(2-hydroxyethyl)-1-piperazineethanesulfonic acid; HPPA, 3-(4-hydroxyphenyl) propionic acid; HPLC, High performance liquid chromatography; HRP, Horseradish peroxidase; NHS, N-hydroxysuccinimide; PBS, Phosphate buffered saline; Ph, Phenolic hydroxyl moieties; PNIPAAm, poly(N-isopropyl acrylamide); RGD, tripeptide arginine-glycine-aspartic acid; RHAMM, Receptor for hyaluronic acid-mediated motility; WSCD·HCl, Water-soluble carbodiimide hydrochloride. |
|
This journal is © The Royal Society of Chemistry 2023 |
Click here to see how this site uses Cookies. View our privacy policy here.