DOI:
10.1039/D3TA02951D
(Paper)
J. Mater. Chem. A, 2023,
11, 14860-14869
Selective conversion of CO2 to CH4 enhanced by WO3/In2O3 S-scheme heterojunction photocatalysts with efficient CO2 activation†
Received
18th May 2023
, Accepted 7th June 2023
First published on 8th June 2023
Abstract
Solar-powered CO2 reduction is a promising approach for mitigating the energy crisis and environmental issues. However, its efficiency is hindered by challenges including difficult CO2 activation, rapid charge recombination, and uncontrollable selectivity. Here, we develop WO3/In2O3 S-scheme heterojunction photocatalysts by depositing In2O3 nanoparticles onto WO3 nanosheets for CO2 photoreduction. The Fermi level difference triggers electron transfer upon coupling, generating an internal electric field pointing from In2O3 to WO3 at the interface. This impels oriented charge transfer and effective separation of the powerful photoinduced carriers. With the unique S-scheme mechanism and the efficient activation of CO2 molecules on the In2O3 surface, the resulting WO3/In2O3 heterostructures exhibit enhanced CO2 photoreduction performance with ∼53.7% selectivity for CH4, without any molecule cocatalyst or scavenger. In situ irradiation X-ray photoelectron spectroscopy, in situ diffuse reflectance infrared Fourier transform spectroscopy, along with density functional theory simulations are conducted to elucidate the photocatalytic and CO2-reduction mechanism, and the enhanced CH4-selectivity.
1. Introduction
Recently, the incessant combustion of fossil fuels has led to a substantial increase in atmospheric carbon dioxide (CO2) concentration, causing grave environmental pollution and concerns about energy scarcity.1–9 To tackle these issues, a promising strategy is the photocatalytic reduction of CO2 to valuable solar fuels driven by abundant, clean, and inexhaustible sunlight.10,11 Nevertheless, the linear CO2 molecules exhibit thermodynamic stability with a high dissociation energy of the C
O bond (∼750 kJ mol−1), making their adsorption and activation onto the catalyst surface challenging.12,13 Additionally, the CO2 photoreduction process is intricate and involves multiple photoelectron transfer, which leads to diverse reduction products, including CO, CH4, CH3OH, etc., with unpredictable selectivity. Although CH4, the major component of natural gas, has the lowest reduction potential among all the products (−0.24 V vs. NHE), it requires eight photoelectrons, making it more difficult to produce than others from a kinetic perspective. Although a variety of photocatalysts have been reported for CO2 reduction to CH4,14–21 most still suffer from uncontrollable selectivity and low conversion efficiency. Thus, designing highly active photocatalysts with efficient CO2 activation and high CH4 selectivity is still a daunting task.
Indium oxide (In2O3) is a popular reduction photocatalyst with a high conduction band level, narrow band gap (∼2.8 eV) and visible light absorption.22,23 It has been reported that stable CO2 molecules can be highly activated on the In2O3 surface to enhance CO2 photoreduction activity, but the selectivity of CH4 has not been modulated.22 In addition, the recombination of photogenerated carriers in unitary In2O3 occurs more readily than transferring to the catalyst surface to participate in photoreactions, leading to poor photocatalytic performance.24,25 Therefore, the development of In2O3-based heterojunction photocatalysts that enable efficient spatial separation of electron/hole pairs and selective CO2-to-CH4 conversion enhancement is desirable but challenging.
Coupling two semiconductors to fabricate an S-scheme heterojunction offers tremendous potential in augmenting photoactivity, owing to the effective separation of photogenerated carriers and their robust redox capability.8,26–28 Generally, S-scheme heterojunctions are constituted of oxidation and reduction photocatalysts. The variance in their Fermi levels elicits electron transfer upon contact, thereby resulting in the bending of energy bands and the creation of an internal electric field (IEF) at their interface. As a consequence of the bent bands, IEF, and coulombic attraction, the futile photogenerated electrons in the oxidation photocatalyst and the holes in the reduction photocatalyst amalgamate, accomplishing the efficient spatial separation of charge carriers with adequate redox ability to boost the photocatalytic performance.29–32 Tungsten trioxide (WO3) is an oxidation photocatalyst that exhibits visible-light response and potent oxidizing ability, attributable to its narrow band gap (2.4–2.8 eV) and positive valence band position.33,34 Given the interweaved band structures and disparity in work functions, the combination of In2O3 with WO3 might engender a desirable S-scheme heterojunction photocatalyst with anticipated charge separation, strong redox ability, and heightened CO2 activation, thereby enhancing CO2 photoreduction performance.22,35,36
Herein, we synthesized WO3/In2O3 S-scheme heterojunction photocatalysts by depositing In2O3 nanoparticles on WO3 nanosheets through an immersion-annealing technique. Compared to pure WO3, the resulting WO3/In2O3 heterostructures revealed elevated selectivity in the conversion of CO2 into CH4 (53.7%) and enhanced CO2 reduction efficiency without any molecular cocatalyst or scavenger. Such superior performance was ascribed to the S-scheme charge transfer mechanism and the effective CO2 activation on the In2O3 surface, which were verified through in situ irradiation X-ray photoelectron spectroscopy (XPS), time-resolved photoluminescence spectroscopy (TRPL), density functional theory (DFT) calculation and temperature-programmed desorption of CO2 (CO2-TPD). In situ diffuse reflectance infrared Fourier transform spectroscopy (DRIFTS) and theoretical simulations were also employed to elucidate the CO2 reduction mechanism and the potential causes for the high selectivity of CH4. Our work may present a novel perspective for designing exceptional S-scheme heterojunction photocatalysts that facilitate efficient CO2 activation and improved selective CO2-to-CH4 conversion.
2. Experimental details
2.1 Synthesis of WO3·H2O nanosheets
Typically, a total of 3.6 mmol of sodium tungstate dihydrate (Na2WO4·2H2O) and 3.0 mL of tetrafluoroboric acid (HBF4, 40 wt%) were dissolved in 60 mL of deionized water. The resulting light-yellow solution was transferred to a 100 mL Teflon-lined stainless autoclave and reacted at 100 °C for 12 h. Upon completion of the reaction, yellow precipitates were centrifuged and washed with deionized water and ethanol for three times, followed by drying at 80 °C in an oven to obtain the WO3·H2O nanosheets.
2.2 Preparation of WO3/In2O3 heterostructures
A certain amount of indium nitrate (In(NO3)3·4H2O) was added to a 30 mL aqueous solution containing 200 mg of WO3·H2O nanosheets. The mixture was magnetically stirred for 2 h, followed by drying in an oven at 90 °C. The resulting solid products were annealed in air at 500 °C for 2 h, with a heating rate of 2 °C min−1. The obtained WO3/In2O3 heterostructures were labelled as WIx (x = 5,10 and 15), where W and I represent WO3 and In2O3 respectively; x is the molar ratio of In2O3 in WO3/In2O3 nanohybrids.
3. Results and discussion
3.1 Activation and photoreduction of CO2 over WO3/In2O3 heterojunctions
The adsorption and activation of CO2 molecules on catalysts were first investigated by DFT calculations since they are essential steps for CO2 photoreduction. Fig. 1a and b show that when CO2 is adsorbed onto the In2O3 surface, the O
C
O bond is visibly bent at an angle of 129.33°, and the bond length is stretched in comparison with free CO2 molecules (1.16 Å). Both the C and O atoms of CO2 form chemical bonds with the O and In atoms of In2O3. Moreover, In2O3 donates 0.2 electrons to CO2 when CO2 adsorbs on In2O3 (Fig. 1c), which is further supported by the planar-averaged charge density difference along the Z direction as shown in Fig. S1.† The investigation of the CO2 adsorption capability reveals that pure In2O3 and WI10 exhibit stronger CO2 adsorption compared to pristine WO3, following the same trend as the N2 adsorption (Fig. S2 and Table S1†). The CO2-TPD results demonstrate that pure WO3, In2O3 and WI10 all display desorption peaks at 80–200 °C assigned to the physical adsorption of CO2 (Fig. 1d).37–39 In the temperature range of 400–800 °C, no significant signals are detected over pristine WO3. However, both pure In2O3 and WI10 reveal three prominent desorption peaks corresponding to the decomposition of b-CO32− (∼450 °C) and m-CO32− (550–750 °C) species, suggesting strong chemisorption interactions between CO2 molecules and In2O3.40–42 Furthermore, in situ DRIFTS spectra obtained after adsorption of CO2 for 15, 30, 45, and 60 min exhibit distinct peaks of formaldehyde (HCHO) (1750 and 1770 cm−1), monodentate carbonate (m-CO32−) (1590, 1560 and 1540 cm−1), bidentate carbonate (b-CO32−) (1425, 1410, 1380, and 1340 cm−1), and bicarbonate (HCO3−) (1180, 1110 and 1030 cm−1) over In2O3 and WI10 (Fig. S3†). These results indicate that CO2 can be chemisorbed and activated by In2O3, and In2O3 is the preferable active site for CO2 photoreduction in the heterojunctions.
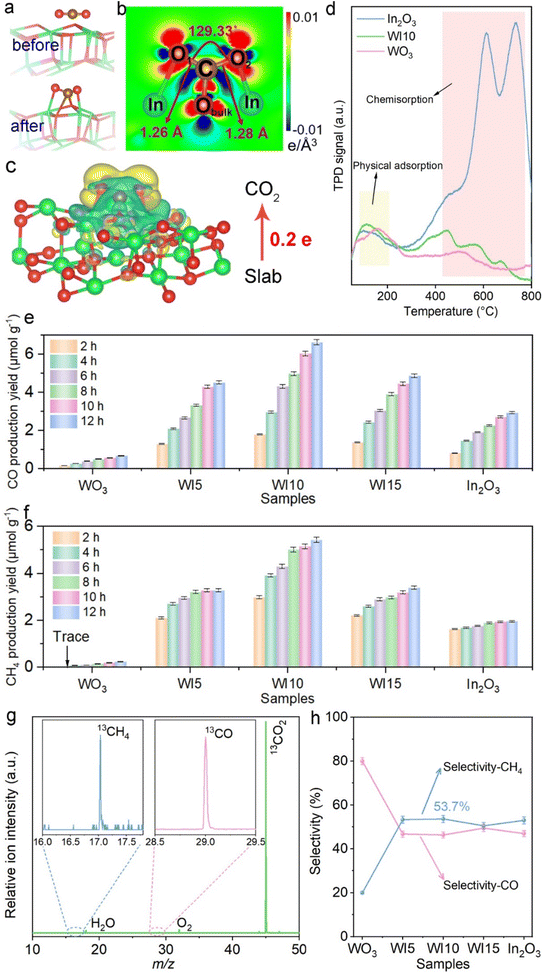 |
| Fig. 1 (a and b) Optimized structure and (c) the corresponding charge density difference images of the CO2 molecule adsorbed on the In2O3 (111) surface. (d) CO2-TPD spectra of In2O3, WI10 and WO3. Photocatalytic CO2 reduction activities over WO3, WIx, and In2O3 during the 12 h experiment performed under visible light irradiation: time course of (e) CO and (f) CH4 production yields. (g) SVUV-PIMS spectra of the products collected after 13CO2 photoreduction for WI10 at hν = 14.2 eV. (h) The selectivity of CO and CH4 over WO3, WIx, and In2O3 samples. | |
The photocatalytic performance toward CO2 reduction was evaluated in an online closed gas-circulation system (OLPCRS-2, Shanghai Boyi Scientific Instrument Co., Ltd) with a quartz reactor under visible light irradiation (Fig. S4†). CO and CH4 were identified as the reduction products, and O2 was determined as the oxidation product. Control experiments revealed that no product can be detected in the dark or in the absence of CO2. As shown in Fig. 1e and f, pristine WO3 and In2O3 both reveal lower production yields of CO and CH4 due to the rapid recombination of photogenerated charge carriers within the unitary photocatalyst. After coupling WO3 with In2O3, the CO2 reduction efficiency is enhanced, reaching the highest evolution yield of 6.6 and 5.4 μmol g−1 for CO and CH4 over WI10. Excessive loading of In2O3 nanoparticles on WO3 nanosheets, i.e., WI15, deteriorates the photocatalytic performance owing to the light shielding effect. During CO2 photoreduction, the photogenerated holes oxidize H2O to produce O2 in the absence of sacrificial agents, and the evolution rate of O2 (Fig. S5†) follows the same tendency as that of CO and CH4. The apparent quantum efficiency (AQE) of CO2 photoreduction was measured at different monochromatic wavelengths including 365, 380, 400, 420, 450, and 500 nm over WO3/In2O3 nanohybrids. Clearly, the trend of AQE matches well with the light absorption spectrum of WI10 (Table S2, Fig. S6†), affirming the photocatalytic nature of CO2 reduction.43–45 The synchrotron-radiation vacuum ultraviolet photoionization mass spectrometry (SVUV-PIMS) was employed to disclose the origin of the products by using isotope-labeled carbon dioxide (13CO2) as the substitute source gas. At a photon energy of 14.2 eV, the WO3/In2O3 heterojunctions yield a mixture of 13CH4 (m/z = 17) and 13CO (m/z = 29) as shown in Fig. 1g, which confirms that the evolved reduction products indeed originate from the photoreduction of CO2 rather than any carbonaceous impurities.46
Notably, the selectivity of CH4 over WO3/In2O3 nanohybrids is higher than that of CO and reaches the maximum of 53.7% over WI10 as revealed in Fig. 1h. In order to uncover the underlying reasons for the high selectivity of CH4, as well as the reaction mechanism, in situ DRIFTS experiments along with DFT calculations were carried out. As shown in Fig. 2a, when CO2/H2O is introduced into the system in the dark, peaks of HCHO, m-CO32−, b-CO32− and HCO3− are detected, strongly remanifesting the chemisorption of CO2 on the catalyst surface.47,48 Under light irradiation, new adsorption peaks assigned to *COOH (1669 cm−1), *CO (2079 cm−1), *CHO (1012 cm−1), *CH2O (2855 cm−1) and *CH3O (1042 and 1181 cm−1) are observed, which are significant intermediates in the conversion of CO2 to CH4.49–51 Accordingly, a probable CO2 photoreduction mechanism over WO3/In2O3 composites is proposed as follows, where * stands for the active sites on WO3/In2O3 heterojunctions.
| *CO2 + H+ + e− → *COOH | (2) |
| *CO + H+ + e− → *CHO or *CO → CO + * | (4) |
| *CHO + H+ + e− → *CH2O | (5) |
| *CH2O + H+ + e− → *CH3O | (6) |
| *CH3O + H+ + e− → CH4 + *O | (7) |
| *OH + H+ + e− → * + H2O | (9) |
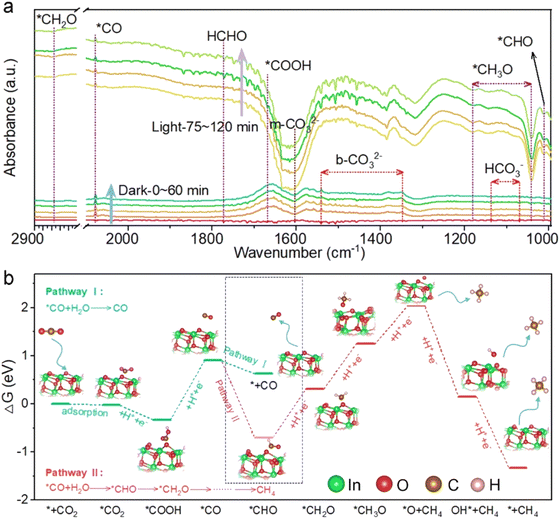 |
| Fig. 2 (a) In situ DRIFT spectra of WI10 after adsorption of CO2/H2O for 15, 30, 45, and 60 min in the dark, and then under light irradiation for 75, 90, 105, and 120 min. (b) Gibbs free energy diagram of CO2 photoreduction over the In2O3 (111) slab. | |
Gibbs free energy (ΔG) calculation of CO2 photoreduction over the In2O3 surface was further conducted to confirm the reaction pathway described above, and the results are presented in Fig. 2b. It is worth noting that the change in ΔG for the protonation of *CO to *CHO (pathway II, −1.61 eV) is much smaller than that for CO desorption form the catalyst surface (pathway I, −0.28 eV), providing a reasonable explanation for the high selectivity of CH4 during CO2 photoreduction.52 Overall, the strong adsorption and activation of CO2 molecules on the In2O3 surface are critical factors contributing to the enhanced CH4 selectivity and CO2 reduction performance.
3.2 Characterization of WO3/In2O3 heterojunctions
Structural characterization studies provide additional insights into understanding the boosted photocatalytic activity. The X-ray diffraction (XRD) pattern of pure WO3 is consistent with the standard monoclinic phase (JCPDS No. 20-1324), and pristine In2O3 is indexed to the cubic phase (JCPDS No. 71-2195), respectively (Fig. S7†). In the WIx nanohybrids, the intensity of diffraction peaks attributed to In2O3 increases with its content (x), indicating the synthesis of WO3/In2O3 heterojunctions. The precursor WO3·H2O exhibits uniform square nanosheets with a smooth surface as revealed in the field emission scanning electron microscopy (FESEM) image (Fig. S8†). After dehydration, pristine WO3 maintains its nanosheet microscopy with some tiny surface wrinkles (Fig. 3a).53 In FESEM and transmission electron microscopy (TEM) images of WO3/In2O3 nanohybrids (WI10) (Fig. 3b and c), In2O3 nanoparticles are clearly observed to be deposited on the surface of WO3 nanosheets. The high-resolution TEM image of WI10 (Fig. 3d) reveals distinct lattice fringes with spacings of 0.292 and 0.375 nm, corresponding to In2O3 (222) and WO3 (020) planes, respectively. The energy-dispersive X-ray spectroscopy (EDX) elemental mappings of WI10 confirm the existence of W, O and In elements, further affirming the formation of WO3/In2O3 nanohybrids. The phase and morphology of spent WI10 were also evaluated, and there are no detectable changes compared with the fresh sample, indicating substantial photostability of the catalysts (Fig. S9†).
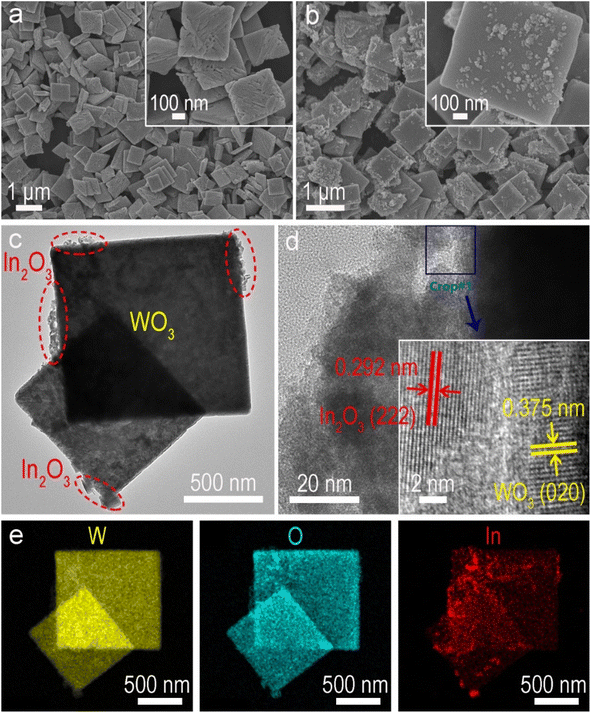 |
| Fig. 3 (a) FESEM image of pure WO3 nanosheets. (b) FESEM, (c) TEM and (d) HRTEM images of WO3/In2O3 nanostructures (WI10). (e) EDX elemental mappings of W, O, and In elements in WO3/In2O3 nanohybrids. | |
UV-vis diffuse reflectance spectroscopy (DRS) was employed to investigate the optical absorption properties of WO3, In2O3 and WI10. The absorption edges of pure WO3 and In2O3 are located at 470 and 465 nm, respectively, corresponding to the bandgap energies of 2.81 and 2.97 eV (Fig. S10†). The light harvesting of WI10 is slightly improved compared to pristine WO3, indicating the successful hybridization of WO3 and In2O3. XPS was carried out to analyze the chemical states and surface compositions of the resultant samples. The survey spectrum of WI10 demonstrates the presence of the In element, as well as W and O elements derived from the WO3 matrix (Fig. S11a†). The W 4f XPS spectra (Fig. 4a) exhibit two distinct peaks at 35.6 and 37.7 eV, corresponding to hexavalent W6+.54,55 The binding energies (BEs) of In 3d5/2 and In 3d3/2 are observed to be 444.2 and 451.7 eV, respectively, confirming the existence of trivalent In3+ (Fig. 4b).56,57 After the photoreaction, the chemical states of WO3/In2O3 heterojunctions exhibit imperceptible changes as manifested by the W 4f and In 3d XPS spectra (Fig. S12†), further confirming the stability of catalysts. The O 1s XPS spectra (Fig. S11b–d†) reveal the presence of lattice oxygen (530.5 eV), adsorbed oxygen (533.2 eV), and surface hydroxyls (531.9 eV) in the samples.58 Notably, the BEs of W 4f in WI10 display negative shifts in comparison with those in pure WO3, while the peaks of In 3d in WI10 shift positively relative to pure In2O3. Such an intriguing phenomenon suggests the migration of electrons from In2O3 to WO3 upon hybridization, creating an IEF with the direction from In2O3 to WO3 at their interface to facilitate the efficient separation of photogenerated charge carriers (as discussed below).
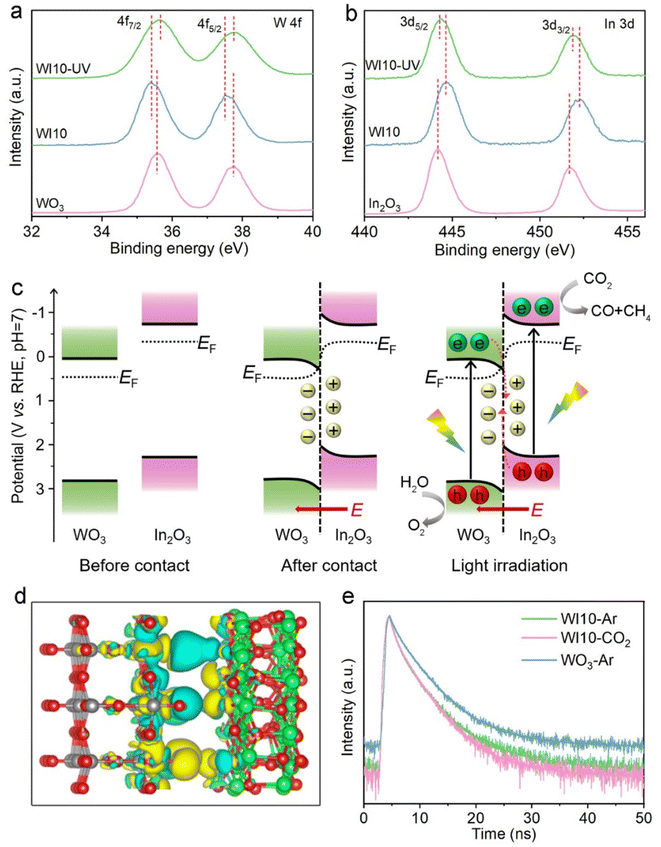 |
| Fig. 4 High-resolution XPS spectra of (a) W 4f, (b) In 3d of WO3, In2O3, and WI10. In situ irradiation XPS measurement was conducted under UV light irradiation. (c) Schematic illustration of the formation and charge separation of the WO3/In2O3 S-scheme heterojunction. (d) The charge density difference of the WO3/In2O3 heterojunctions; cyan and yellow regions represent electron depletion and accumulation, respectively; grey, green, and red spheres represent W, In and O atoms, respectively. (e) TRPL spectra of WO3 and WI10 in different atmospheres. | |
3.3 Insights into the S-scheme charge separation mechanism
The band structure of WO3 and In2O3 was first investigated to unravel the charge transfer and separation mechanism over WO3/In2O3 heterojunctions. The potentials of the VB maximum and CB minimum of WO3 and In2O3 are calculated to be 2.88 and 0.07 V, as well as 2.28 and −0.69 V (vs. RHE), respectively, based on Mott–Schottky plots, valence band XPS spectra (Fig. S13†) and the band gap (Fig. S10b†). According to the electrostatic potentials along the z-axis of WO3 (001) and In2O3 (111) (Fig. S14†), the work function (Φ) of WO3 and In2O3 is estimated to be 6.54 and 5.87 eV, respectively, indicating that In2O3 has a higher Fermi level (EF) than WO3. Additionally, the contact potential difference (CPD) between the samples and the standard gold tip of the Kelvin probe instrument reveals that the calculated Φ level of WO3 is larger than that of In2O3 (Fig. S15†), aligning with the DFT results. As a consequence, electrons in In2O3 will spontaneously diffuse to WO3 upon contact until reaching the same EF at their interface, which will lead to the bending of their energy bands and the creation of an IEF pointing from In2O3 to WO3 (Fig. 4c). The charge density difference of DFT simulation (Fig. 4d) shows that In2O3 donates electrons (cyan area) while WO3 gains electrons (yellow area) at their interface, further confirming the above electron transfer pathway between WO3 and In2O3.
Under light irradiation, the electrons in WO3 and In2O3 VBs are initially photoexcited to their CBs. Due to the bent bands and IEF, the photogenerated electrons in the WO3 CB tend to transfer to the In2O3 VB and recombine with its holes, indicating the formation of S-scheme heterojunctions between WO3 and In2O3. Ultimately, the powerful photoelectrons in the In2O3 CB and photoholes in the WO3 VB are reserved to participate in photocatalytic reactions (Fig. 4c). Such an S-scheme charge transfer route not only efficiently separates photoinduced carriers, but also preserves the strong redox ability of surviving electrons and holes, thereby definitely enhancing CO2 photoreduction performance and selectively producing CH4.
In situ irradiation XPS was carried out to verify the proposed S-scheme charge transfer pathway within the WO3/In2O3 heterostructure. As presented in Fig. 4a and b, the BEs of W 4f shift positively under illumination, while the In 3d BEs show negative shifts with reference to their respective values measured in the dark. These results support the transfer of photogenerated electrons from WO3 to In2O3 and validate the suggested S-scheme photocatalytic mechanism. To gain a better understanding of how charge carriers are transferred in WO3/In2O3 heterostructures, TRPL spectra of pure WO3 and WI10 were collected under different atmospheres. As shown in Fig. 4e and Table S3,† the shorter lifetime (τ1) of WI10 is only 0.76 ns in an Ar atmosphere, which is shorter than that of pure WO3 (0.96 ns). This suggests that the photoexcited electrons in WO3 transfer to In2O3 and the radiative recombination is retarded. Consequently, only a small fraction of photoelectrons of WI10 engage in the photocarrier recombination to emit fluorescence, resulting in shorter lifetimes of τ2 and τ3. These results provide further evidence for the S-scheme charge separation mechanism in WO3/In2O3 heterojunctions. In addition, the average lifetime (τa) of WI10 in a CO2 atmosphere is shorter compared to that in an Ar atmosphere (3.38 vs. 3.56 ns), which indicates that a great deal of photoinduced electrons in the In2O3 CB are delivered to CO2 molecules for surface photoreduction and fewer photocarriers are involved in detectable recombination.59,60 Photoelectrochemical measurements were further conducted to analyze the charge separation efficiency. As shown in Fig. S16,† WI10 displays higher photocurrent density and smaller charge transfer resistance (Rct) as compared with pristine WO3, indicating superior electron/hole separation efficiency and lower resistance for charge transfer in the WO3/In2O3 S-scheme heterojunctions.33,61
Based on the aforementioned analyses, it is suggested that the combination of WO3 and In2O3 to construct S-scheme heterojunctions can effectively promote charge transfer and reduce electron–hole recombination. In concert with the efficient adsorption and activation of CO2 molecules on the In2O3 surface, the resulting WO3/In2O3 S-scheme heterojunctions possess more photoelectrons with high reduction capacity to participate in the CO2 photoreduction reaction and produce multi-electron products such as CH4.
4. Conclusion
To summarize, an S-scheme WO3/In2O3 heterojunction photocatalyst was constructed by depositing In2O3 nanoparticles on WO3 nanosheets via a facile immersion-annealing method. DFT calculations, CO2-TPD and in situ DRIFTS revealed that the WO3/In2O3 heterostructures displayed efficient chemisorption and activation of CO2 molecules. The difference in Fermi levels caused electrons to transfer from In2O3 to WO3, thus forming an IEF and bending the energy bands at the interface, as evidenced by XPS and DFT results. Under light irradiation, photoinduced electrons in the WO3 CB recombined with the holes in the In2O3 VB, driven by the bent bands and IEF, following an S-scheme charge transfer pathway and achieving efficient separation efficiency of powerful photogenerated charge carriers. As a result, the optimized WO3/In2O3 heterojunctions exhibited enhanced CO2 photoreduction performance with ∼53.7% selectivity for CH4, in the absence of any molecule cocatalyst or scavenger. The CO2 photoreduction mechanism and underlying reasons for high CH4-selectivity were uncovered via in situ DRIFTS, along with Gibbs free-energy calculations. This work offers a perspective on designing novel S-scheme heterojunction photocatalysts for efficient CO2 photoreduction and selective conversion of CO2 to CH4.
Author contributions
Y. H., J. Y., and F. X. conceived and designed the experiments. Y. H. and Z. Y. conducted material synthesis, the characterizations of the materials, and the photocatalytic test. C. L. and Y. P. performed the SVUV-PIMS test. Y. H., J. Y., D. X., W. M., and F. X. contributed to data analysis. Y. H. wrote the manuscript. F. X. supervised the project, performed DFT calculations, and revised the manuscript. All authors discussed the results and commented on the manuscript.
Conflicts of interest
There are no conflicts to declare.
Acknowledgements
This work was supported by the National Key Research and Development Program of China (2022YFE0115900), National Natural Science Foundation of China (52003213, 22238009, 51932007, and 22261142666), China Postdoctoral Science Foundation (2022M712958) and the Natural Science Foundation of Hubei Province of China (2022CFA001).
References
- S. Patial, R. Kumar, P. Raizada, P. Singh, Q. Van Le, E. Lichtfouse, D. Le Tri Nguyen and V.-H. Nguyen, Boosting light-driven CO2 reduction into solar fuels: Mainstream avenues for engineering ZnO-based photocatalysts, Environ. Res., 2021, 197, 111134 CrossRef CAS PubMed.
- J. Ma, X. Li, Y. Li, G. Jiao, H. Su, D. Xiao, S. Zhai and R. Sun, Single-atom zinc catalyst for co-production of hydrogen and fine chemicals in soluble biomass solution, Adv. Powder Mater., 2022, 1, 100058 CrossRef.
- H. Zhao, R. Yu, S. Ma, K. Xu, Y. Chen, K. Jiang, Y. Fang, C. Zhu, X. Liu, Y. Tang, L. Wu, Y. Wu, Q. Jiang, P. He, Z. Liu and L. Tan, The role of Cu1-O3 species in single-atom Cu/ZrO2 catalyst for CO2 hydrogenation, Nat. Catal., 2022, 5, 818–831 CrossRef CAS.
- Y. Li, M. Zhang, L. Zhou, S. Yang, Z. Wu and Y. Ma, Recent Advances in Surface-Modified g-C3N4-Based Photocatalysts for H2 Production and CO2 Reduction, Acta Phys.-Chim. Sin., 2021, 37, 2009030 Search PubMed.
- W. Fu, J. Fan and Q. Xiang, Ag2S Quantum Dots Decorated on Porous Cubic-CdS Nanosheets-assembled Flowers for Photocatalytic CO2 Reduction, Chin. J. Struct. Chem., 2022, 41, 2206039–2206047 CAS.
- X. Fei, H. Tan, B. Cheng, B. Zhu and L. Zhang, 2D/2D Black Phosphorus/g-C3N4 S-Scheme Heterojunction Photocatalysts for CO2 Reduction Investigated using DFT Calculations, Acta Phys.-Chim. Sin., 2021, 37, 2010027 Search PubMed.
- V. Hasija, A. Kumar, A. Sudhaik, P. Raizada, P. Singh, Q. Van Le, T. T. Le and V.-H. Nguyen, Step-scheme heterojunction photocatalysts for solar energy, water splitting, CO2 conversion, and bacterial inactivation: a review, Environ. Chem. Lett., 2021, 19, 2941–2966 CrossRef CAS.
- F. Xu, K. Meng, B. Cheng, S. Wang, J. Xu and J. Yu, Unique S-scheme heterojunctions in self-assembled TiO2/CsPbBr3 hybrids for CO2 photoreduction, Nat. Commun., 2020, 11, 4613 CrossRef CAS PubMed.
- F. Xu, K. Meng, S. Cao, C. Jiang, T. Chen, J. Xu and J. Yu, Step-by-Step Mechanism Insights into the TiO2/Ce2S3 S-Scheme Photocatalyst for Enhanced Aniline Production with Water as a Proton Source, ACS Catal., 2022, 12, 164–172 CrossRef CAS.
- B. Fang, Z. Xing, D. Sun, Z. Li and W. Zhou, Hollow semiconductor photocatalysts for solar energy conversion, Adv. Powder Mater., 2022, 1, 100021 CrossRef.
- Z. Li, D. Wu, W. Gong, J. Li, S. Sang, H. Liu, R. Long and Y. Xiong, Highly Efficient Photocatalytic CO2 Methanation over Ru-Doped TiO2 with Tunable Oxygen Vacancies, Chin. J. Struct. Chem., 2022, 41, 2212043–2212050 CAS.
- R. Das, S. Sarkar, R. Kumar, S. D. Ramarao, A. Cherevotan, M. Jasil, C. P. Vinod, A. K. Singh and S. C. Peter, Noble-Metal-Free Heterojunction Photocatalyst for Selective CO2 Reduction to Methane upon Induced Strain Relaxation, ACS Catal., 2022, 12, 687–697 CrossRef CAS.
- X. An, Q. Tang, H. Lan, H. Liu, X. Yu, J. Qu, H. Lin and J. Ye, Facilitating Molecular Activation and Proton Feeding by Dual Active Sites on Polymeric Carbon Nitride for Efficient CO2 Photoreduction, Angew. Chem., Int. Ed., 2022, 61, e2022127 Search PubMed.
- Z. Miao, Q. Wang, Y. Zhang, L. Meng and X. Wang, In situ construction of S-scheme AgBr/BiOBr heterojunction with surface oxygen vacancy for boosting photocatalytic CO2 reduction with H2O, Appl. Catal., B, 2022, 301, 120802 CrossRef CAS.
- Y. Wang, H. Huang, Z. Zhang, C. Wang, Y. Yang, Q. Li and D. Xu, Lead-free perovskite Cs2AgBiBr6@g-C3N4 Z-scheme system for improving CH4 production in photocatalytic CO2 reduction, Appl. Catal., B, 2021, 282, 119570 CrossRef CAS.
- M. Tahir and B. Tahir, Constructing S-scheme 2D/0D g-C3N4/TiO2 NPs/MPs heterojunction with 2D-Ti3AlC2 MAX cocatalyst for photocatalytic CO2 reduction to CO/CH4 in fixed-bed and monolith photoreactors, J. Mater. Sci. Technol., 2022, 106, 195–210 CrossRef.
- L. Wang, B. Zhu, J. Zhang, J. Ghasemi, M. Mousavi and J. Yu, S-scheme heterojunction photocatalysts for CO2 reduction, Matter, 2022, 5, 4187–4211 CrossRef CAS.
- X. Xiong, Y. Zhao, R. Shi, W. Yin, Y. Zhao, G. I. N. Waterhouse and T. Zhang, Selective photocatalytic CO2 reduction over Zn-based layered double hydroxides containing tri or tetravalent metals, Sci. Bull., 2020, 65, 987–994 CrossRef CAS PubMed.
- Z. Liu, S. Wu, M. Li and J. Zhang, Selective Photocatalytic CO2 Reduction to CH4 on Tri-s-triazine-Based Carbon Nitride via Defects and Crystal Regulation: Synergistic Effect of Thermodynamics and Kinetics, ACS Appl. Mater. Interfaces, 2022, 14, 25417–25426 CrossRef CAS PubMed.
- S. Hu, Z. Deng, M. Xing, S. Wu and J. Zhang, Highly Dispersed Cobalt Centers on UiO-66-NH2 for Photocatalytic CO2 Reduction, Catal. Lett., 2023, 153, 1475–1482 CrossRef CAS.
- S. Hu, Z. Deng, M. Xing, S. Wu and J. Zhang, Construction of Cu cocatalyst on TiO2 for regulating the selectivity of photocatalytic CO2 reduction, Res. Chem. Intermed., 2022, 48, 3275–3287 CrossRef CAS.
- G. Han, C. Liu, Y. Pan, W. Macyk, S. Wageh, A. A. Al-Ghamdi and F. Xu, Artificial Photosynthesis over Tubular In2O3/ZnO Heterojunctions Assisted by Efficient CO2 Activation and S-Scheme Charge Separation, Adv. Sustain. Syst., 2023, 7, 2200381 CrossRef CAS.
- H. Xu, Y. Wang, X. Dong, N. Zheng, H. Ma and X. Zhang, Fabrication of In2O3/In2S3 microsphere heterostructures for efficient and stable photocatalytic nitrogen fixation, Appl. Catal., B, 2019, 257, 117932 CrossRef CAS.
- L. Wang and J. Yu, CO2 capture and in situ photocatalytic reduction, Chem Catal., 2022, 2, 428–430 CrossRef CAS.
- L. Wang, C. Bie and J. Yu, Challenges of Z-scheme photocatalytic mechanisms, Trends Chem., 2022, 4, 973–983 CrossRef CAS.
- S. Wageh, A. A. Al-Ghamdi and L. Liu, S-Scheme Heterojunction Photocatalyst for CO2 Photoreduction, Acta Phys.-Chim. Sin., 2021, 37, 2010024 Search PubMed.
- Z. Wang, B. Cheng, L. Zhang, J. Yu, Y. Li, S. Wageh and A. A. Al-Ghamdi, S-Scheme 2D/2D Bi2MoO6/BiOI van der Waals heterojunction for CO2 photoreduction, Chin. J. Catal., 2022, 43, 1657–1666 CrossRef CAS.
- K. Alkanad, A. Hezam, Q. A. Drmosh, S. S. G. Chandrashekar, A. A. AlObaid, I. Warad, M. A. Bajiri and L. N. Krishnappagowda, Construction of Bi2S3/TiO2/MoS2 S-Scheme Heterostructure with a Switchable Charge Migration Pathway for Selective CO2 Reduction, Sol. RRL, 2021, 5, 2100501 CrossRef CAS.
- L. Zhang, J. Zhang, H. Yu and J. Yu, Emerging S-Scheme Photocatalyst, Adv. Mater., 2022, 34, 2107668 CrossRef CAS PubMed.
- F. A. Qaraah, S. A. Mahyoub, A. Hezam, A. Qaraah, F. Xin and G. Xiu, Synergistic effect of hierarchical structure and S-scheme heterojunction over O-doped g-C3N4/N-doped Nb2O5 for highly efficient photocatalytic CO2 reduction, Appl. Catal., B, 2022, 315, 121585 CrossRef CAS.
- Q. Xu, L. Zhang, B. Cheng, J. Fan and J. Yu, S-Scheme Heterojunction Photocatalyst, Chem, 2020, 6, 1543–1559 CAS.
- Z. Wang, B. Cheng, L. Zhang, J. Yu and H. Tan, BiOBr/NiO S-Scheme Heterojunction Photocatalyst for CO2 Photoreduction, Sol. RRL, 2022, 6, 2100587 CrossRef CAS.
- Y. Lin, G. Huang, L. Chen, J. Zhang and L. Liu, Enhanced CO2 Photoreduction by Ni(OH)2-x/WO3 Nanofibers with Efficient CO2 Activation and Charge Separation, Adv. Sustain. Syst., 2022, 7, 2200364 CrossRef.
- W. Shi, X. Guo, C. Cui, K. Jiang, Z. Li, L. Qu and J. Wang, Controllable synthesis of Cu2O decorated WO3 nanosheets with dominant (001) facets for photocatalytic CO2 reduction under visible-light irradiation, Appl. Catal., B, 2019, 243, 236–242 CrossRef CAS.
- Y. Yang, Y. Pan, X. Tu and C. Liu, Nitrogen doping of indium oxide for enhanced photocatalytic reduction of CO2 to methanol, Nano Energy, 2022, 101, 107613 CrossRef CAS.
- S. Wang, B. Guan and X. Lou, Construction of ZnIn2S4-In2O3 Hierarchical Tubular Heterostructures for Efficient CO2 Photoreduction, J. Am. Chem. Soc., 2018, 140, 5037–5040 CrossRef CAS PubMed.
- Z. Deng, S. Hu, J. Ji, S. Wu, H. Xie, M. Xing and J. Zhang, Deep insight of the influence of Cu valence states in co-catalyst on CO2 photoreduction, Appl. Catal., B, 2022, 316, 121621 CrossRef CAS.
- J. Li, X. Pei, Z. Wang, Y. Li and G. Zhang, Boosted charge transfer and selective photocatalytic CO2 reduction to CH4 over sulfur-doped K0.475WO3 nanorods under visible light: Performance and mechanism insight, Appl. Surf. Sci., 2022, 605, 154632 CrossRef CAS.
- M. Li, Z. Liu, S. Wu and J. Zhang, Advances for CO2 Photocatalytic Reduction in Porous Ti-Based Photocatalysts, ACS ES&T Eng., 2022, 2, 942–956 Search PubMed.
- S. Hu, C. Dong, Z. Deng, M. Xing and J. Zhang, Tuning Reaction Pathway of CO2 Photoreduction via PtRu Bimetallic Microstructure Regulation, J. Phys. Chem. C, 2021, 125, 10406–10412 CrossRef CAS.
- Y. Pu, Y. Luo, X. Wei, J. Sun, L. Li, W. Zou and L. Dong, Synergistic effects of Cu2O-decorated CeO2 on photocatalytic CO2 reduction: Surface Lewis acid/base and oxygen defect, Appl. Catal., B, 2019, 254, 580–586 CrossRef CAS.
- J. Zhao, Y. Wang, Y. Li, X. Yue and C. Wang, Phase-dependent enhancement for CO2 photocatalytic reduction over CeO2/TiO2 catalysts, Catal. Sci. Technol., 2016, 6, 7967–7975 RSC.
- A. Meng, B. Cheng, H. Tan, J. Fan, C. Su and J. Yu, TiO2/polydopamine S-scheme heterojunction photocatalyst with enhanced CO2-reduction selectivity, Appl. Catal., B, 2021, 289, 120039 CrossRef CAS.
- S. Wageh, O. A. Al-Hartomy, M. F. Alotaibi and L. Liu, Ionized cocatalyst to promote CO2 photoreduction activity over core-triple-shell ZnO hollow spheres, Rare Met., 2022, 41, 1077–1079 CrossRef CAS.
- M. Sayed, F. Xu, P. Kuang, J. Low, S. Wang, L. Zhang and J. Yu, Sustained CO2-photoreduction activity and high selectivity over Mn, C-codoped ZnO core-triple shell hollow spheres, Nat. Commun., 2021, 12, 4936 CrossRef CAS PubMed.
- X. Li, Y. Sun, J. Xu, Y. Shao, J. Wu, X. Xu, Y. Pan, H. Ju, J. Zhu and Y. Xie, Selective visible-light-driven photocatalytic CO2 reduction to CH4 mediated by atomically thin CuIn5S8 layers, Nat. Energy, 2019, 4, 690–699 CrossRef CAS.
- K. Wang, J. Lu, Y. Lu, C. Lau, Y. Zheng and X. Fan, Unravelling the C-C coupling in CO2 photocatalytic reduction with H2O on Au/TiO2-x: Combination of plasmonic excitation and oxygen vacancy, Appl. Catal., B, 2021, 292, 120147 CrossRef CAS.
- Y. Zeng, Z. Tang, X. Wu, A. Huang, X. Luo, G. Q. Xu, Y. Zhu and S. L. Wang, Photocatalytic oxidation of methane to methanol by tungsten trioxide-supported atomic gold at room temperature, Appl. Catal., B, 2022, 306, 120919 CrossRef.
- L. Liu, H. Zhao, J. M. Andino and Y. Li, Photocatalytic CO2 Reduction with H2O on TiO2 Nanocrystals: Comparison of Anatase, Rutile, and Brookite Polymorphs and Exploration of Surface Chemistry, ACS Catal., 2012, 2, 1817–1828 CrossRef CAS.
- J. Sheng, Y. He, J. Li, C. Yuan, H. Huang, S. Wang, Y. Sun, Z. Wang and F. Dong, Identification of Halogen-Associated Active Sites on Bismuth-Based Perovskite Quantum Dots for Efficient and Selective CO2-to-CO Photoreduction, ACS Nano, 2020, 14, 13103–13114 CrossRef CAS PubMed.
- Y. Liu, D. Shen, Q. Zhang, Y. Lin and F. Peng, Enhanced photocatalytic CO2 reduction in H2O vapor by atomically thin Bi2WO6 nanosheets with hydrophobic and nonpolar surface, Appl. Catal., B, 2021, 283, 119630 CrossRef CAS.
- X. Yang, S. Wang, N. Yang, W. Zhou, P. Wang, K. Jiang, S. Li, H. Song, X. Ding, H. Chen and J. H. Ye, Oxygen vacancies induced special CO2 adsorption modes on Bi2MoO6 for highly selective conversion to CH4, Appl. Catal., B, 2019, 259, 118088 CrossRef CAS.
- H. Yu, M. Wang, J. Yan, H. Dang, H. Zhu, Y. Liu, M. Wen, G. Li and L. Wu, Complete mineralization of phenolic compounds in visible-light-driven photocatalytic ozonation with single-crystal WO3 nanosheets: Performance and mechanism investigation, J. Hazard. Mater., 2022, 433, 128811 CrossRef CAS PubMed.
- S. Cao, J. Yu, S. Wageh, A. A. Al-Ghamdi, M. Mousavi, J. B. Ghasemi and F. Xu, H2-production and electron-transfer mechanism of a noble-metal-free WO3@ZnIn2S4 S-scheme heterojunction photocatalys, J. Mater. Chem. A, 2022, 10, 17174–17184 RSC.
- S. Li, M. Cai, Y. Liu, C. Wang, R. Yan and X. Chen, Constructing Cd0.5Zn0.5S/Bi2WO6 S-scheme heterojunction for boosted photocatalytic antibiotic oxidation and Cr(VI) reduction, Adv. Powder Mater., 2023, 2, 100073 CrossRef.
- S. Han, B. Li, L. Huang, H. Xi, Z. Ding and J. Long, Construction of ZnIn2S4-CdIn2S4 Microspheres for Efficient Photo-catalytic Reduction of CO2 with Visible Light, Chin. J. Struct. Chem., 2022, 41, 2201007–2201013 CAS.
- L. Yin, D. Chen, M. Hu, H. Shi, D. Yang, B. Fan, G. Shao, R. Zhang and G. Shao, Microwave-assisted growth of In2O3 nanoparticles on WO3 nanoplates to improve H2S-sensing performance, J. Mater. Chem. A, 2014, 2, 18867–18874 RSC.
- B. Lei, W. Cui, P. Chen, L. Chen, J. Li and F. Dong, C-Doping Induced Oxygen-Vacancy in WO3 Nanosheets for CO2 Activation
and Photoreduction, ACS Catal., 2022, 12, 9670–9678 CrossRef CAS.
- S. Nayak, L. Mohapatra and K. Parida, Visible light-driven novel g-C3N4/NiFe-LDH composite photocatalyst with enhanced photocatalytic activity towards water oxidation and reduction reaction, J. Mater. Chem. A, 2015, 3, 18622–18635 RSC.
- Y. Hu, X. Hao, Z. Cui, J. Zhou, S. Chu, Y. Wang and Z. Zou, Enhanced photocarrier separation in conjugated polymer engineered CdS for direct Z-scheme photocatalytic hydrogen evolution, Appl. Catal., B, 2020, 260, 118131 CrossRef CAS.
- M. Sayed, B. Zhu, P. Kuang, X. Liu, B. Cheng, A. A. Al Ghamdi, S. Wageh, L. Zhang and J. Yu, EPR Investigation on Electron Transfer of 2D/3D g-C3N4/ZnO S-Scheme Heterojunction for Enhanced CO2 Photoreduction, Adv. Sustain. Syst., 2022, 6, 2100264 CrossRef CAS.
|
This journal is © The Royal Society of Chemistry 2023 |
Click here to see how this site uses Cookies. View our privacy policy here.