DOI:
10.1039/D3TA05297D
(Communication)
J. Mater. Chem. A, 2023,
11, 26435-26441
Efficient SF6 capture and separation in robust gallium- and vanadium-based metal–organic frameworks†
Received
1st September 2023
, Accepted 16th November 2023
First published on 17th November 2023
Abstract
Sulfur hexafluoride (SF6) is a highly potent greenhouse gas (GHG) that is mainly emitted from high-voltage electrical applications. The global warming potential (GWP) of the gas is almost 23
000 times that of CO2 and therefore, controlling its emission and recovery is of great importance from both an environmental and economic perspective. Solid adsorbents and adsorption-based technology is a cost-effective and energy-efficient pathway to recapture SF6 from its sources, which usually consist of dilute SF6 in N2. Here, we present a group of four highly porous and robust gallium- or vanadium-based metal–organic frameworks (MOFs) with exceptional SF6 uptake and selectivity. In particular, the novel gallium 1,2,4,5-tetrakis(4-carboxlatephenyl)benzene (TCPB4−) MOF (Ga-TBAPy) possesses 1-dimensional channels of suitable size (5.2 × 8.4 Å and 5.3 × 10 Å) to adsorb up to 2.25 mmol g−1 of SF6 at 10 kPa with an excellent SF6-over-N2 selectivity of 418. Ga-TCPB also exhibits high chemical stability in aqueous and acidic media as well as in organic solvents. 3D electron diffraction (3D ED) patterns combined with high-resolution electron microscopy images were employed to investigate the structure of these water-stable and cyclable MOF SF6 adsorbents. Furthermore, this study demonstrates the possibility of using these highly stable MOFs to capture SF6 from a gas mixture as well as how MOFs can offer an alternative and efficient way to mitigate the global warming contributions from the emission of SF6.
The rise in global warming and associated climate-related issues has long been connected to the anthropogenic emission of greenhouse gases (GHGs). Six industrially relevant GHGs were specified in the Kyoto Protocol in 1997, namely, carbon dioxide (CO2), hydrofluorocarbons, methane (CH4), nitrous oxide (N2O), perfluorocarbons, and sulfur hexafluoride (SF6).1 While accounting for 76% of the total greenhouse gas emissions,2 CO2 remains the most abundant of the six GHGs. However, the large global warming potential of SF6 (22
800 times higher than that of CO2)3 and its long atmospheric lifetime (3200 years)4 pose serious concerns. The compound has found wide use (typically in a mixture with N2)5 in electrical systems, particularly in high-voltage systems and switchgear, as well as in the semiconductor industry, due to its excellent dielectric properties, high stability, and low toxicity.3 The atmospheric concentration of SF6 has, as a consequence, steadily risen in the last 25 years from 3.5 ppt to 11.32 ppt (as of April 2023)6 due to the growing electricity demands in both developing and developed countries. Sources of SF6 emissions were in these places attributed to the handling, leaks, and improper recycling of the gas during maintenance and repair.7 The recovery, as well as recycling of SF6, particularly from dilute mixtures, is therefore highly important both from an environmental and economic perspective. Adsorption-based processes using solid nanosorbents, such as zeolites,8,9 porous carbons,10,11 and metal–organic frameworks (MOFs),12,13 have shown great promise as a cost-effective and energy-efficient method for GHG capture and separation.14 MOFs have garnered particular attention in the last couple of decades due to their rich structural diversity, tunable pore size, and surface chemistry which arise from the arrangement of their discrete building units – organic molecules (linkers) and metal ions/clusters (secondary building units).12,15 Furthermore, the formation of robust MOFs are highly dependent on how these building units are structured and interact – the geometry and rigidity of the organic linkers, the strength of the coordination bonds in the frameworks, as well as the connectivity of the secondary building units.16–20 MOFs constructed from rod secondary building units (SBUs) encompass an intriguing category of materials that are characterized by their high stability, which is partially derived from their topological structure.16 The infinite SBU chains form framework structures that in many cases possess uniform 1-dimensional channels that may be used for efficient diffusion, separation, and adsorption of guest molecules.21 A number of rod MOFs have been reported to date. These rod MOFs are based on common divalent transition metals, such as cobalt, zinc, copper, manganese, and nickel (e.g. MOF-74 [M2(C8H2O6)] (where M denotes the divalent metal cation)22–25 and MOF-71 [Co(C8H2O4)(C3H7NO)]26), or higher valence metals such as aluminum(III) (e.g. MIL-53 [Al(OH)(C8H2O4)]27 and CAU-10-H [Al(OH)(C8H2O4)]28), gallium(III) (e.g. MIL-53 [Ga(OH)(C8H2O4)]29 and Ga-fumarate [Ga(OH)(C4H2O4)]30), and vanadium(III/IV) (e.g. MIL-68 [V(OH)(C8H2O4)]31 and MIL-47 [VO(C8H2O4)]27). Combining the strong coordinative bonds that emerge between high valence metal cations (such as gallium (Ga)- and vanadium (V)) and carboxylate-based linkers with the topological stability, and the uniform channel arrangements of rod structures, is an effective strategy for constructing MOFs with excellent stability as well as exceptional sorption properties for small guest molecules (e.g. H2O, CO2, SO2).32–35 Furthermore, introducing rigid and extended linkers into these structures is one approach for building frameworks with increased pore dimensions while retaining the framework's structural and chemical properties. Such moisture-stable frameworks exhibiting high thermal and chemical stability, as well as a high adsorption selectivity for larger guest molecules, are therefore greatly relevant for many industrial applications wherein a sorbent's long-term performance and lifetime are important from an economic perspective. MOF sorbents proposed for SF6 capture36–44 have previously not been shown to exhibit these combined properties. With the aim to construct stable rod MOFs with a suitable pore size for capturing the potent greenhouse gas SF6, we synthesized four robust and highly porous MOFs using gallium or vanadium with two different rigid tetratopic linkers (Fig. 1a). Solvothermal reactions between VOSO4·xH2O or Ga(NO3)3·xH2O and H4TBAPy or H4TCPB in N,N-dimethylformamide (DMF) yielded four 3-dimensional porous structures, namely, [V2O2(TBAPy)] (V-TBAPy), [V2O2(TCPB)] (V-TCPB), [Ga2(OH)2(TBAPy)] (Ga-TBAPy), and [Ga2(OH)2(TCPB)] (Ga-TCPB) (Fig. 1b–e and S15–S16, S21†). The crystal structure of the MOFs was determined by 3-dimensional electron diffraction (3D ED) (Fig. S1–S8 and Table S1†). The presence of twinning on Ga-TBAPy prevented the direct determination of the framework structure, however, due to its shared similarities with V-TBAPy, a structure model of Ga-TBAPy was built based on the solved structure of V-TBAPy. In brief, the V(IV) metal ions in V-TBAPy was replaced by Ga(III) ions for Ga-TBAPy, and the entire structure was geometry optimized based on the cell parameters obtained by Le Bail refinement against PXRD data (Fig. S14†) of Ga-TBAPy. Low-dose high resolution transmission electron microscope (HRTEM) images were also collected to verify these structures. As shown in Fig. 1c and S1, S3, S5, and S7,† the pore structures were clearly observed in the HRTEM images and matched well with structure models. The four as-synthesized MOFs crystallized in either a monoclinic P2/m (V-TBAPy, Ga-TBAPy, and Ga-TCPB) or an orthorhombic Pcma space group (V-TCPB) with comparable unit cell dimensions aside from unit cell length along the c-axis (15.638 Å, 16.095 Å, 21.590 Å, and 10.970 Å for V-TBAPy, Ga-TBAPy, V-TCPB, and Ga-TCPB, respectively) (Table S1†). The structure of the MOFs shared similarities with other TBAPy- and TCPB-based framework structures such as ROD-7 ([In2(OH)2(TBAPy)]),45 ACM-1 ([TiO2(TBAPy)]),46 CAU-9 ([Al2(OH)2(TCPB)]),47 and Sc-CAU-9-TCPB ([Sc2(OH)2(TCPB)]),48 which contain rod SBUs consisting of infinite chains of trans corner-sharing octahedra. The Ga(III) and V(IV) metal ions (Fig. S17 and Table S6†) in the structures are coordinated to four different carboxylate groups from the TBAPy4− or TCPB4− linkers and are connected to adjacent metal ions through two bridging μ2-hydroxide or μ2-oxo groups in the inorganic chain. The resulting frameworks thus possess two types of 1-dimensional rhombohedral channels propagating along [100] (Fig. 1d and S10–S13†) – a larger channel with dimensions of approximately 5.3–7.9 × 9.5–10 Å and a smaller one 4.9–5.5 Å × 8.4–10 Å, as well as narrow pores (approx. 3.1 Å–3.4 Å) in the [010] direction (taking the van der Waals radii of the atoms into account). The TCPB-based MOFs were found to possess channels of narrower dimensions (approx. 5.2 Å × 10 Å, Fig. S12–S13†) which are of suitable size to host larger greenhouse gas molecules such as SF6 (kinetic diameter of 5.5 Å). The structural stability of the MOF materials in the presence of external stimuli (e.g. temperature) and different chemical environments play a pivotal role in their utilization as sorbents in real-life applications, particularly relating to operating conditions and sorbent handling as well as lifetime. The as-synthesized TCPB-MOFs showed excellent stability in acidic and neutral aqueous media as well as in both protic and aprotic organic solvents at ambient temperatures, while the TBAPy-MOFs were found to be unstable in acidic solutions (Fig. S18 and S19†). The stability of the frameworks is derived from the strong V–O and Ga–O coordinative bonds in the frameworks in conjunction with the shape of the inorganic building units and pore structure. This may in turn lower solvent accessibility, metal–oxygen bond susceptibility for hydrolysis as well as linker dissociation.49,50 Complete dissolution of the TCPB-based frameworks was only observed in the presence of strong bases such as 0.1 M NaOH. Furthermore, all frameworks also showed high thermal stabilities up to 350 °C and 450 °C for the V- and Ga-based MOFs, respectively (Fig. S20†).
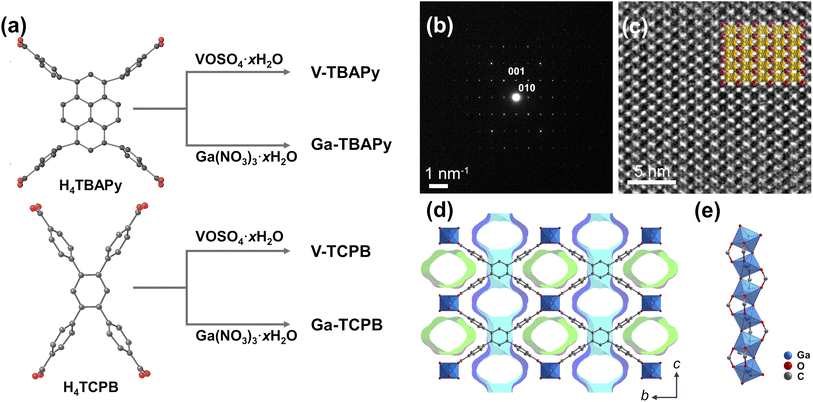 |
| Fig. 1 (a) Synthesis route of Ga- and V-based MOFs with the respective tetratopic H4TBAPy and H4TCPB ligands, (b) selected area electron diffraction (SAED) image of Ga-TCPB along [100], (c) corresponding HRTEM image with an insert of the crystal structure of Ga-TCPB as viewed along the a-axis, (d) structure of Ga-TCPB as viewed along the a-axis showing the presence of two unique pores (colored in green and blue), and (e) the rod SBU running along the a-axis in the structure. | |
The porosity of the TBAPy- and TCPB-MOFs was confirmed by N2 sorption at 77 K (Fig. 2a). All four MOFs showed high Brunauer–Emmett–Teller (BET) surface areas between 1107–1484 m2 g−1 (Fig. S22–S25 and Table S7†). The density functional theory pore size distributions (DFT-PSD) showed the presence of two types of primary pores as identified by the N2 isotherms (Fig. S22–S25 and Table S7†) ranging in size from 6.0–8.6 Å and 5.4–7.0 Å in the TBAPy- and TCPB-based MOFs, respectively. Taking into account the errors in the calculated DFT-PSDs, arising from deviations between the utilized DFT model and experimental data, the estimated pore apertures were comparable with the dimensions of the 1-dimensional channels running along [100] in the crystal structures. The narrow channels along [010] and [001] were not detected due to the kinetic diameter of the N2 molecules exceeding the size of the pores (approx. 3.0–3.5 Å). The adsorption properties of the TBAPy- and TCPB-MOFs were further investigated using SF6 and N2 as well as other well-known greenhouse gases, i.e. CO2, and CH4 at pressures up to 100 kPa and at 293 K (Fig. 2b and S26†). All four MOFs showed preferential uptake towards the greenhouse gases, especially SF6 over N2. The CO2, CH4, and N2 adsorption isotherms (Fig. S26†) of the MOFs showed a linear uptake with increasing pressure (2.83–3.49 mmol g−1, 0.88–1.38 mmol g−1, and 0.30–0.40 mmol g−1 at 100 kPa, respectively). This observation signifies a moderate to low affinity between the frameworks and the CO2 as well as CH4 and N2 molecules, which indicates that the pore dimensions are unsuitable for the selective capture of these small molecules (<3.8 Å). However, in regards to SF6, the TBAPy-based MOFs had the highest SF6 uptake at 100 kPa of 3.50 mmol g−1 and 3.28 mmol g−1 for Ga-TBAPy and V-TBAPy, respectively, followed by 3.07 mmol g−1 and 2.95 mmol g−1 for V-TCPB and Ga-TCPB. These numbers correspond well with the overall decrease in pore volume from the TBAPy- to TCPB-based MOFs. Notably, the shape of the SF6 isotherm over the pressure range differs significantly between the TBAPy- and TCPB-MOFs (Fig. 2b). The TCPB-MOFs adopt Langmuir-shaped isotherms that approach their apparent saturation capacities at lower pressures as compared to the TBAPy-MOFs for which the SF6 uptake capacities can be observed to steadily increase with pressure. These differences are attributed to the structural dissimilarities between the TBAPy- and TCPB-linkers (Fig. S9†) in which the benzene-core of the TCPB-linker contracts the channel dimensions along [100], resulting in a reduction in pore volume and an increased affinity between the framework and SF6 molecules. Correspondingly, the SF6 uptake capacities of the TCPB-MOFs at 10 kPa (2.29 mmol g−1 and 2.26 mmol g−1 in V- and Ga-TCPB), were significantly higher than their TBAPy-counterparts (1.33 mmol g−1 in both V- and Ga-TBAPy). Furthermore, the SF6 uptakes of Ga- and V-TCPB were comparable to other high-performing MOFs such as Co-MOF-74 (2.09 mmol g−1),38 Ni(adc)(dabco)0.5 (2.23 mmol g−1),51 and Ni(ina)2 (2.39 mmol g−1)43 but lower than Cu-MOF-NH2 (3.39 mmol g−1)44 at 10 kPa and 298 K (Fig. 2e, f and Table S9†). The SF6 occupancy per unit cell in the MOFs subsequently reached values of 2.59, 2.90, 4.22 and 2.09 for V-TBAPy, Ga-TBAPy, V-TCPB, and Ga-TCPB, respectively, and corresponding SF6 densities of 860 g L−1, 764 g L−1, 929 g L−1, and 919 g L−1 which equates to 126–154 times the density of gaseous SF6 (6.04 g L−1 at 293 K and 101.325 kPa). The SF6 occupancy per unit cell for V-TCPB is nearly twice that of Ga-TCPB due to the former MOF crystallizing in a different crystal system and thus possessing a larger unit cell. The SF6 densities in the MOFs imply a high affinity between the framework and SF6 molecules as well as efficient packing of the SF6 molecules in the channels of the structures. The guest–host interaction was further evaluated by their isosteric enthalpies (−ΔHads) of SF6 adsorption (Fig. 2c). The −ΔHads at low coverage (i.e. 0.24 mmol g−1) was calculated to be 27–30 kJ mol−1 which is comparable to other proposed SF6 sorbents (Fig. 2f). The −ΔHads furthermore increased to 31–34 kJ mol−1 in the TBAPy-based MOFs while it remained comparatively constant (31–32 kJ mol−1) in the TCPB-based frameworks as the SF6 coverage increased to 2.80 mmol g−1. This increase in −ΔHads is indicative of growing lateral SF6–SF6 interactions which occur as the SF6 coverage in the channels of the TBAPy-based frameworks increases, while the unchanging −ΔHads imply that the adsorption sites and SF6–SF6 interactions in V- and Ga-TCPB are energetically similar.52–54 Overall the calculated −ΔHads at both low- and high-coverage is moderate but typical for physisorption processes, thus implying that the sorbents may be regenerated at mild conditions.
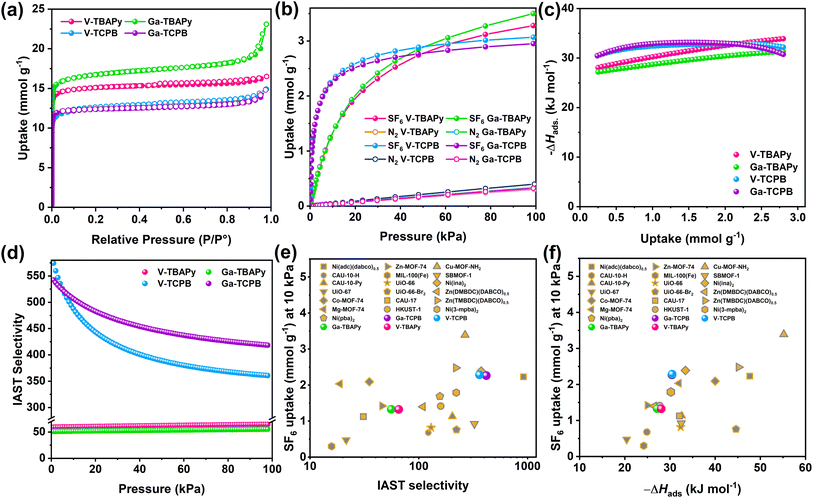 |
| Fig. 2 (a) N2 sorption isotherms recorded at 77 K (filled and open circles denote the adsorption and desorption branches, respectively), (b) SF6 and N2 adsorption isotherms recorded at 293 K, plots of (c) the isosteric enthalpies of SF6 adsorption (−ΔHads), and (d) IAST selectivities, and comparisons between SF6 uptake capacities at 10 kPa and (e) IAST selectivities, (f) −ΔHads (at zero or low coverage) of the synthesized TBAPy- and TCPB-MOFs and other porous sorbents. | |
Differences in guest–host interactions between SF6 and N2 molecules are apparent from the adsorption isotherms (Fig. 2b) for which the gradient of the isotherms, as well as the total N2 uptake capacities (0.30–0.40 mmol g−1 at 293 K and 100 kPa), are significantly smaller for N2 as compared to SF6 and furthermore implies a high adsorption selectivity of SF6 over N2. The Henry's constants (KH) of SF6 and N2 (Fig. S30 and Tables S12–15†), which corresponds to the partition of the adsorbate between the adsorbed phase and bulk phase in the Henry's regime (i.e. low pressures), show SF6 values 50 to 400 times higher than that of N2. The Henry's law SF6-over-N2 ideal selectivities range from 47.5 (Ga-TBAPy), 53.8 (V-TBAPy) to 365 (V-TCPB) and 437 (Ga-TCPB), further indicating the excellent separation performance of, in particular, the TCPB-based MOFs at low pressures. Furthermore, ideal adsorption solution theory (IAST) selectivities of the MOFs were evaluated by considering a gas mixture composed of SF6/N2 at a ratio of 10
:
90. A significant difference between the performance of the TBAPy- and TCPB-based MOFs was observed (Fig. 2d) – the TBAPy-based MOFs exhibited fairly low IAST selectivities of 55 (Ga-TBAPy) and 65 (V-TBAPy) while high selectivities of 361 (V-TCPB) and 418 (Ga-TCPB) were observed for the TCPB-based MOFs at 100 kPa and 293 K. Neither the TBAPy- or TCPB-based MOFs outperform high-performing MOFs such as Cu-MOF-NH2 (3.39 mmol g−1 SF6 uptake at 10 kPa, 298 K and SF6/N2 IAST selectivity of 266.2 at 100 kPa)44 and Ni(adc)(dabco)0.5 (2.23 mmol g−1 SF6 uptake at 10 kPa, 298 K and SF6/N2 IAST selectivity of 989.0 at 100 kPa)51 in terms of SF6 uptake capacity at 10 kPa nor adsorption selectivity. However, it is crucial to highlight that the combined sorbent properties determine their practical application. Ga-TCPB exhibits a high SF6 uptake capacity above 2.20 mmol g−1 at 10 kPa, SF6/N2 IAST selectivity greater than 400, and −ΔHads of SF6 adsorption below 40 kJ mol−1 indicating a low energy penalty for sorbent regeneration, as well as excellent thermal- and chemical stability in aqeous and acidic environments. These combined properties make Ga-TCPB unique among sorbents reported for SF6 capture, to our knowledge, and presents it as a highly promising material for SF6 capture and separation.
Aspects regarding a sorbent's hydrophilic characteristics are also important to consider particularly in regard to handling and for applications using gas mixtures containing water vapor. The TBAPy- and TCPB-based MOFs generally exhibit low water uptake capacities which is attributed to the hydrophobic structure of the TBAPy- and TCPB-linkers (Fig. 3a), in particular, Ga-TBAPy (0.95 mmol g−1, 0.02 g g−1 at P/P° = 0.93) and V-TCPB (8.40 mmol g−1, 0.15 g g−1 at P/P° = 0.93) display linear isotherm shapes throughout the partial pressure range. The water sorption isotherms for V-TBAPy (22.81 mmol g−1, 0.41 g g−1 at P/P° = 0.93) and Ga-TCPB (8.48 mmol g−1, 0.15 g g−1 at P/P° = 0.93), on the other hand, display a Type V shape which has been observed for other microporous MOFs possessing rod-like SBUs such as CAU-10-H55 and MIL-53.56,57 The lack of hysteresis in the sorption isotherms furthermore indicates that the adsorption process in all MOFs proceeds without changes, distortion, or degradation of the frameworks,58 further supporting our previous observations that the structures remain stable in humid environments. Adsorption kinetics and sorbent cycling stability are two other parameters that are important to evaluate in order to assess a sorbent's performance. The TCPB-based MOFs were found to display more rapid SF6 adsorption as compared to their TBAPy counterparts (Fig. 3b) – reaching 80% of their total uptake capacities within 1 min and 1.2–1.4 min, respectively. Furthermore, all MOFs showed excellent recyclability (Fig. 3c) with a negligible decrease in SF6 uptake capacity from the first cycle to the tenth cycle.
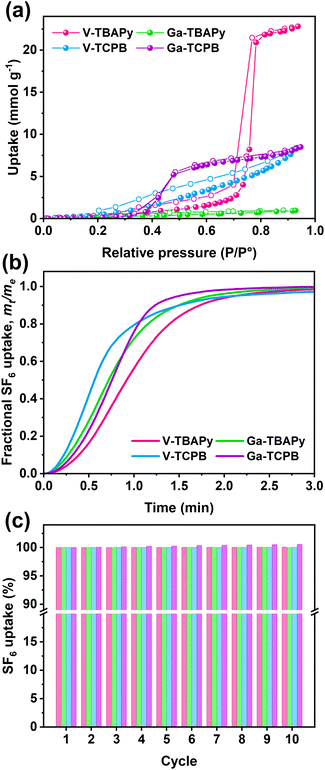 |
| Fig. 3 (a) Water sorption isotherms recorded at 293 K (solid and open spheres represent the adsorption and desorption branches, respectively), (b) gravimetric SF6 adsorption profiles recorded at 303 K, 100 kPa, and (c) gravimetric SF6 adsorption cycles. | |
In summary, we have reported on four highly porous gallium- and vanadium-based rod MOFs containing two different tetratopic linkers – TBAPy4− and TCPB4−. These structures were shown to possess 1-dimensional channels approx. 5.3–7.9 × 9.5–10 Å and 4.9–5.5 Å × 8.4–10 Å in dimensions suitable for SF6 capture. Structural differences between the TBAPy- and TCPB-based linkers were found to impart a narrower pore size in the V- and Ga-TPCB MOFs. The narrow pores enhanced the affinity between the frameworks and the SF6 molecules, as compared to the TBAPy-MOFs. In particular, Ga-TCPB exhibited an excellent SF6 uptake capacity of 2.25 mmol g−1 at 10 kPa and a high SF6/N2 IAST selectivity above 400. The MOF also displayed a moderately low −ΔHads below 40 kJ mol−1 which in combination with the great thermo-chemical stability and recyclability of the material renders it as a highly promising sorbent for SF6 capture. Utilizing robust rod MOFs containing high valence metals and extended tetratopic linkers to both increase guest–host interactions and framework stability is, to our knowledge, unique among reported SF6 sorbents, and is a highly promising strategy for constructing materials suited for practical applications.
Conflicts of interest
There are no conflicts to declare.
Acknowledgements
The authors thank the Swedish Foundation for Strategic Environmental Research (Mistra) (Project Name: Mistra TerraClean, Project number 2015/31), the Swedish Research Council (Grant No. 2020-04029 and No. 2019-03729) and Swedish Research Council for Sustainable Development (FORMAS, Grant No. 2018-00651) for their financial support. We also acknowledge Myfab Uppsala for providing facilities and experimental support. Myfab is funded by the Swedish Research Council (2019-00207) as a national research infrastructure. This work was also supported by the Center for High-resolution Electron Microscopy (CℏEM), ShanghaiTech University (EM02161943), Shanghai Science and Technology Plan (21DZ2260400).
Notes and references
- D. Liu, X. Guo and B. Xiao, Sci. Total Environ., 2019, 661, 750–766 CrossRef CAS PubMed.
- S. Akdag and H. Yildirim, Heliyon, 2020, 6, e03396 CrossRef CAS PubMed.
- W.-T. Tsai, J. Fluorine Chem., 2007, 128, 1345–1352 CrossRef CAS.
-
P. Forster, V. Ramaswamy, P. Artaxo, T. Berntsen, R. Betts, D. W. Fahey, J. Haywood, J. Lean, D. C. Lowe, G. Myhre, J. Nganga, R. Prinn, G. Raga, M. Schulz and R. Van Dorland, Changes in Atmospheric Constituents and in Radiative Forcing, Cambridge, United Kingdom and New York, NY, USA, 2007 Search PubMed.
- L. G. Christophorou and R. J. Van Brunt, IEEE Trans. Dielectr. Electr. Insul., 1995, 2, 952–1003 CrossRef CAS.
-
Trends in Atmospheric Sulfur Hexafluoride, https://gml.noaa.gov/ccgg/trends_sf6/, (accessed 28 August, 2023) Search PubMed.
- B. K. Sovacool, S. Griffiths, J. Kim and M. Bazilian, Renewable Sustainable Energy Rev., 2021, 141, 110759 CrossRef CAS.
- S. Prodinger, R. S. Vemuri, T. Varga, P. B. McGrail, R. K. Motkuri and M. A. Derewinski, New J. Chem., 2016, 40, 4375–4385 RSC.
- O. Cheung, Z. Bacsik, Q. Liu, A. Mace and N. Hedin, Appl. Energy, 2013, 112, 1326–1336 CrossRef CAS.
- H. Zhang, Z. Wang, X. Luo, J. Lu, S. Peng, Y. Wang and L. Han, Front. Chem., 2019, 7, 00919 CrossRef CAS PubMed.
- S. Jung, Y.-K. Park and E. E. Kwon, J. CO2 Util., 2019, 32, 128–139 CrossRef CAS.
- M. Ding, R. W. Flaig, H.-L. Jiang and O. M. Yaghi, Chem. Soc. Rev., 2019, 48, 2783–2828 RSC.
- R. Aniruddha, I. Sreedhar and B. M. Reddy, J. CO2 Util., 2020, 42, 101297 CrossRef CAS.
- A. Dong, D. Chen, Q. Li and J. Qian, Small, 2023, 19, e2201550 CrossRef PubMed.
- R. L. Siegelman, E. J. Kim and J. R. Long, Nat. Mater., 2021, 20, 1060–1072 CrossRef CAS PubMed.
- F. M. Amombo Noa, M. Abrahamsson, E. Ahlberg, O. Cheung, C. R. Göb, C. J. McKenzie and L. Öhrström, Chem, 2021, 7, 2491–2512 CAS.
- X.-L. Lv, S. Yuan, L.-H. Xie, H. F. Darke, Y. Chen, T. He, C. Dong, B. Wang, Y.-Z. Zhang, J.-R. Li and H.-C. Zhou, J. Am. Chem. Soc., 2019, 141, 10283–10293 CrossRef CAS PubMed.
- T. He, X.-J. Kong and J.-R. Li, Acc. Chem. Res., 2021, 54, 3083–3094 CrossRef CAS PubMed.
- N. Li, J. Xu, R. Feng, T.-L. Hu and X.-H. Bu, Chem. Commun., 2016, 52, 8501–8513 RSC.
- R. E. Morris and L. Brammer, Chem. Soc. Rev., 2017, 46, 5444–5462 RSC.
- A. Schoedel, M. Li, D. Li, M. O'Keeffe and O. M. Yaghi, Chem. Rev., 2016, 116, 12466–12535 CrossRef CAS PubMed.
- J. L. C. Rowsell and O. M. Yaghi, J. Am. Chem. Soc., 2006, 128, 1304–1315 CrossRef CAS PubMed.
- S. R. Caskey, A. G. Wong-Foy and A. J. Matzger, J. Am. Chem. Soc., 2008, 130, 10870–10871 CrossRef CAS PubMed.
- R. Sanz, F. Martínez, G. Orcajo, L. Wojtas and D. Briones, Dalton Trans., 2013, 42, 2392–2398 RSC.
- W. L. Queen, M. R. Hudson, E. D. Bloch, J. A. Mason, M. I. Gonzalez, J. S. Lee, D. Gygi, J. D. Howe, K. Lee, T. A. Darwish, M. James, V. K. Peterson, S. J. Teat, B. Smit, J. B. Neaton, J. R. Long and C. M. Brown, Chem. Sci., 2014, 5, 4569–4581 RSC.
- N. L. Rosi, J. Kim, M. Eddaoudi, B. Chen, M. O'Keeffe and O. M. Yaghi, J. Am. Chem. Soc., 2005, 127, 1504–1518 CrossRef CAS PubMed.
- K. Barthelet, J. Marrot, D. Riou and G. Férey, Angew. Chem., Int. Ed., 2002, 41, 281–284 CrossRef CAS PubMed.
- H. Reinsch, M. A. van der Veen, B. Gil, B. Marszalek, T. Verbiest, D. de Vos and N. Stock, Chem. Mater., 2012, 25, 17–26 CrossRef.
- H. Reinsch and D. De Vos, Microporous Mesoporous Mater., 2014, 200, 311–316 CrossRef CAS.
- Y. Zhang, B. E. G. Lucier, S. M. McKenzie, M. Arhangelskis, A. J. Morris, T. Friščić, J. W. Reid, V. V. Terskikh, M. Chen and Y. Huang, ACS Appl. Mater. Interfaces, 2018, 10, 28582–28596 CrossRef CAS PubMed.
- K. Barthelet, J. Marrot, G. Férey and D. Riou, Chem. Commun., 2004, 520–521 RSC.
- X. Qian, B. Yadian, R. Wu, Y. Long, K. Zhou, B. Zhu and Y. Huang, Int. J. Hydrogen, 2013, 38, 16710–16715 CrossRef CAS.
- M. A. van der Veen, S. Canossa, M. Wahiduzzaman, G. Nenert, D. Frohlich, D. Rega, H. Reinsch, L. Shupletsov, K. Markey, D. E. De Vos, M. Bonn, N. Stock, G. Maurin and E. H. G. Backus, Adv. Mater., 2023, e2210050 CrossRef PubMed.
- S. Couck, J. F. M. Denayer, G. V. Baron, T. Rémy, J. Gascon and F. Kapteijn, J. Am. Chem. Soc., 2009, 131, 6326–6327 CrossRef CAS PubMed.
- A. López-Olvera, J. A. Zárate, E. Martínez-Ahumada, D. Fan, M. L. Díaz-Ramírez, P. A. Sáenz-Cavazos, V. Martis, D. R. Williams, E. Sánchez-González, G. Maurin and I. A. Ibarra, ACS Appl. Mater. Interfaces, 2021, 13, 39363–39370 CrossRef PubMed.
- Y. Wu, T. Yan, W. Zhang, S. Chen, Y. Fu, Z. Zhang and H. Ma, Ind. Eng. Chem. Res., 2022, 61, 13603–13611 CrossRef CAS.
- M.-B. Kim, T.-H. Kim, T.-U. Yoon, J. H. Kang, J.-H. Kim and Y.-S. Bae, J. Ind. Eng. Chem., 2020, 84, 179–184 CrossRef CAS.
- M.-B. Kim, S.-J. Lee, C. Y. Lee and Y.-S. Bae, Microporous Mesoporous Mater., 2014, 190, 356–361 CrossRef CAS.
- M.-B. Kim, T.-U. Yoon, D.-Y. Hong, S.-Y. Kim, S.-J. Lee, S.-I. Kim, S.-K. Lee, J.-S. Chang and Y.-S. Bae, Chem. Eng. J., 2015, 276, 315–321 CrossRef CAS.
- M.-B. Kim, K.-M. Kim, T.-H. Kim, T.-U. Yoon, E.-J. Kim, J.-H. Kim and Y.-S. Bae, Chem. Eng. J., 2018, 339, 223–229 CrossRef CAS.
- L. Yan, H.-T. Zheng, L. Song, Z.-W. Wei, J.-J. Jiang and C.-Y. Su, Chem. Eng. J., 2023, 472, 145145 CrossRef CAS.
- S.-T. Zheng, R.-Y. Jiang, Y. Jiang, S. Ni, G.-W. Guan, S.-Q. Shao, Y.-C. Wang, S.-M. Wang and Q.-Y. Yang, Sep. Purif. Technol., 2023, 318, 123957 CrossRef CAS.
- S.-M. Wang, X.-T. Mu, H.-R. Liu, S.-T. Zheng and Q.-Y. Yang, Angew. Chem., Int. Ed., 2022, 61, e202207066 CrossRef CAS PubMed.
- J. Ren, M. Chang, W. Zeng, Y. Xia, D. Liu, G. Maurin and Q. Yang, Chem. Mater., 2021, 33, 5108–5114 CrossRef CAS.
- K. C. Stylianou, R. Heck, S. Y. Chong, J. Basca, J. T. A. Jones, Y. Z. Khimyak, D. Bradshaw and M. J. Rosseinsky, J. Am. Chem. Soc., 2010, 132, 4119–4130 CrossRef CAS PubMed.
- A. Cadiau, N. Kolobov, S. Srinivasan, M. G. Goesten, H. Haspel, A. V. Bavykina, M. R. Tchalala, P. Maity, A. Goryachev, A. S. Poryvaev, M. Eddaoudi, M. V. Fedin, O. F. Mohammed and J. Gascon, Angew. Chem., Int. Ed., 2020, 59, 13468–13472 CrossRef CAS PubMed.
- M. Krüger, R. Siegel, A. Dreischarf, H. Reinsch, J. Senker and N. Stock, Microporous Mesoporous Mater., 2015, 216, 27–35 CrossRef.
- P. Rönfeldt, H. Reinsch, M. P. M. Poschmann, H. Terraschke and N. Stock, Cryst. Growth Des., 2020, 20, 4686–4694 CrossRef.
- Y. An, X. Lv, W. Jiang, L. Wang, Y. Shi, X. Hang and H. Pang, Green Chem. Eng., 2023 DOI:10.1016/j.gce.2023.07.004.
- S. Gai, J. Zhang, R. Fan, K. Xing, W. Chen, K. Zhu, X. Zheng, P. Wang, X. Fang and Y. Yang, ACS Appl. Mater. Interfaces, 2020, 12, 8650–8662 CrossRef CAS PubMed.
- M. Chang, T. Yan, Y. Wei, J.-X. Wang, D. Liu and J.-F. Chen, Chem. Mater., 2022, 34, 9134–9143 CrossRef CAS.
- J. A. Dunne, M. Rao, S. Sircar, R. J. Gorte and A. L. Myers, Langmuir, 1996, 12, 5896–5904 CrossRef CAS.
- T. Düren, Y.-S. Bae and R. Q. Snurr, Chem. Soc. Rev., 2009, 38, 1237–1247 RSC.
- F. R. Siperstein, C. Avendaño, J. J. Ortiz and A. Gil-Villegas, AIChE J., 2021, 67, e17186 CrossRef CAS.
- D. Fröhlich, S. K. Henninger and C. Janiak, Dalton Trans., 2014, 43, 15300–15304 RSC.
- S. Devautour-Vinot, G. Maurin, F. Henn, C. Serre and G. Férey, Phys. Chem. Chem. Phys., 2010, 12, 12478–12485 RSC.
- F.-X. Coudert, A. U. Ortiz, V. Haigis, D. Bousquet, A. H. Fuchs, A. Ballandras, G. Weber, I. Bezverkhyy, N. Geoffroy, J.-P. Bellat, G. Ortiz, G. Chaplais, J. Patarin and A. Boutin, J. Phys. Chem. C, 2014, 118, 5397–5405 CrossRef CAS.
- M. J. Kalmutzki, C. S. Diercks and O. M. Yaghi, Adv. Mater., 2018, 30, e1704304 CrossRef PubMed.
|
This journal is © The Royal Society of Chemistry 2023 |
Click here to see how this site uses Cookies. View our privacy policy here.