An intelligent peptide recognizes and traps Mycobacterium tuberculosis to inhibit macrophage phagocytosis†
Received
18th August 2022
, Accepted 23rd November 2022
First published on 28th November 2022
Abstract
Tuberculosis is a major public health concern worldwide, and it is a serious threat to human health for a long period. Macrophage phagocytosis of Mycobacterium tuberculosis (M. tuberculosis) is a crucial process for granuloma formation, which shelters the bacteria and gives them an opportunity for re-activation and spread. Herein, we report an intelligent anti-microbial peptide that can recognize and trap the M. tuberculosis, inhibiting the macrophage phagocytosis process. The peptide (Bis-Pyrene-KLVFF-WHSGTPH, in abbreviation as BFH) first self-assembles into nanoparticles, and then forms nanofibers upon recognizing and binding M. tuberculosis. Subsequently, BFH traps M. tuberculosis by the in situ formed nanofibrous networks and the trapped M. tuberculosis are unable to invade host cells (macrophages). The intelligent anti-microbial peptide can significantly inhibit the phagocytosis of M. tuberculosis by macrophages, thereby providing a favorable theoretical basis for inhibiting the formation of tuberculosis granulomas.
Introduction
Tuberculosis remains one of the world's largest infectious “killers”.1 The WHO Global Tuberculosis Report 2021 states that the number of deaths from tuberculosis has increased for the first time in nearly a decade due to the COVID-19 pandemic, and it has aggravated an already sub-optimal global tuberculosis response.2 Almost all Mycobacterium tuberculosis (M. tuberculosis) infections are spread by inhalation of aerosols, making lungs the most common site of infection.3,4 At the infected site, alveolar macrophages phagocytose M. tuberculosis.4 More importantly, following the phagocytosis of M. tuberculosis, macrophages secrete a variety of chemoattractant cytokines, which help recruit uninfected macrophages, monocytes and lymphocytes from the circulation to the site of infection, initiating the formation of tuberculous granulomas, which is the histological hallmark of tuberculosis.5–7
Tuberculous granulomas have long been endowed with the role of host protective structures that can “isolate” infecting mycobacteria.7–11 Tuberculosis is caused by bacterium-host interactions involving a complex series of processes.12M. tuberculosis can replicate after being phagocytosed by macrophages in the lung because of their ability to subvert phagocytic endocytic trafficking and resist innate defense mechanisms.13–15 Infected macrophages migrate into tissues and aggregate into tuberculous granulomas.15 The formation of granulomas in turn allows mycobacteria to proliferate and spread within the host.7,16–18 When the human body is affected by factors such as malnutrition, HIV co-infection and immunodeficiency, the host's immunity weakens. This ultimately leads to granuloma destruction through necrolysis of mycobacterial-infected macrophages, thereby causing tuberculosis infection and recurrence.19–21 Therefore, the inhibition of phagocytosis of M. tuberculosis by macrophages is the first and crucial step to prevent the formation of granulomas against tuberculosis infection and recurrence.
Nanomedicine is the medical application of nanotechnology and nanomaterial.22–27 The development of nanotechnology and nanomaterial will bring a profound revolution to the medical field. Supramolecular chemistry, also known as ‘chemistry beyond the molecule’, relies on non-covalent interactions between molecules to self-assemble into molecular assemblies.28 Based on the concept of supramolecular chemistry, peptide may self-assemble into nanomaterials that function as nanomedicine.29 In recent years, the development of biomimetic binding-induced fibrillogenesis (BIF) peptides showed great significance in the fight against cancer and bacteria.30–37 For example, we previously designed a human defensin-6 mimic peptide (HDMP), which recognizes S. aureus through BIF process from nanoparticles (NPs) to nanofibers (NFs) to capture S. aureus, inhibiting the invasion into host cells.38
Herein, we design a biomimetic BIF peptide, Bis-Pyrene-KLVFF-WHSGTPH (BFH), which consists of three modules. (i) A ligand peptide sequence WHSGTPH can target and bind M. tuberculosis.39 (ii) The KLVFF sequence is a peptide skeleton for β-sheet fibrous structures.40 (iii) Bis-pyrene (BP) enables the formation of BFH NPs (Fig. 1a). Moreover, the fluorescence arising from the aggregation-induced emission (AIE) of BP can be used to indicate BFH.41 Mechanistically, BFH first self-assembles into NPs, which subsequently bind to M. tuberculosis, Bacillus Calmette–Guérin (BCG) and transform into NFs to form fibrous networks. The BCG are trapped by fibrous networks of BFH, achieving effective resistance to macrophage phagocytosis (Scheme 1).
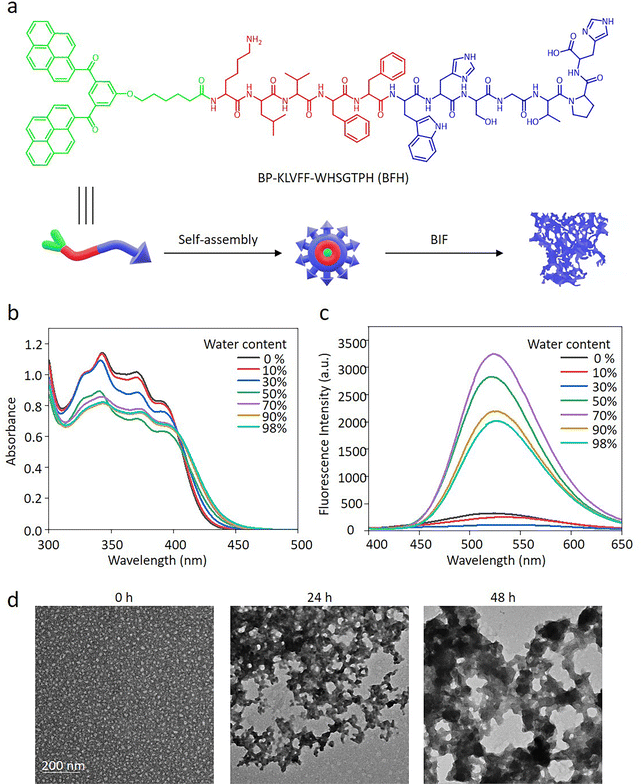 |
| Fig. 1 BFH self-assemble into nanoparticles and transform into nanofibers once incubated with BCG. (a) Molecular structure of BFH and schematic diagram of transformation from NPs to NFs through binding-induced fibrillogenesis (BIF) process. (b) UV−vis and (c) fluorescence spectra during the formation of BFH NPs (30 μM) in mixed H2O/DMSO solutions with different content of water. (d) TEM images of BFH NPs at 0 h, BFH NFs at 24 h, and BFH NFs at 48 h when incubated with BCG. | |
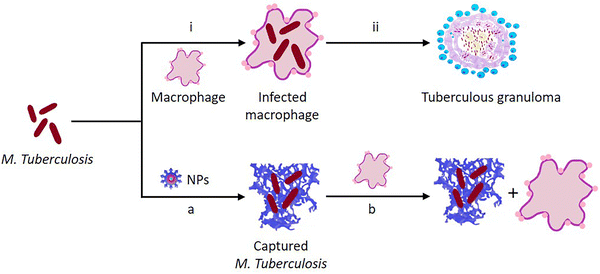 |
| Scheme 1 Schematic mechanism of infection of M. tuberculosis and the formation of tuberculous granulomas, and in situ capture M. tuberculosis by BFH NPs against infection of macrophage. (i) M. tuberculosis is phagocytosed by macrophages to form infected macrophage. (ii) Infected macrophages can recruit more macrophages and other immune cells to phagocytose M. tuberculosis, and the infected cells form tuberculous granulomas. (a) BFH NPs specifically recognize and capture M. tuberculosis by binding-induced fibrillogenesis and resultant fibrous networks. (b) Macrophages are unable to phagocytose M. tuberculosis captured by BFH fibrous networks. | |
Results and discussion
Preparation and characterization of BFH and BAH NPs
The BFH peptide and the control peptide BP-KAAGG-WHSGTPH (BAH) with the replacement of β-sheeting forming KLVFF by KAAGG (Fig. S1 and S2, ESI†) were dissolved in DMSO, respectively, and subsequently injected into water. As a result, the BFH and BAH self-assembled into NPs (Fig. 1a), which was confirmed by the UV-vis absorption and fluorescence spectra. The absorption peaks of BFH and BAH monomers around 320 to 400 nm decreased with the emerging bathochromic absorption peak, indicating the aggregation of BP (Fig. 1b and Fig. S3a, ESI†). Meanwhile, as the water ratio in the mixed solution increased, the fluorescence intensity at 525 nm increased gradually, indicating the aggregation of BP (Fig. 1c and Fig. S3b, ESI†). Through the particle size analysis from dynamic light scattering (DLS), BFH and BAH were well-dispersed NPs with diameters of 24.03 ± 1.00 nm and 28.21 ± 4.77 nm, respectively (Fig. S4, ESI†). The experimental results showed that zeta potentials of BFH and BAH NPs were +12.4 ± 3.5 mV and +11.1 ± 5.1 mV, respectively (Fig. S5, ESI†). The nanoparticulation of BFH or BAH was mainly driven by the hydrophobic and π–π interactions of BP upon dispersion in water.
BFH NPs transform into NFs upon encountering BCG
The peptide sequence WHSGTPH was screened for the BCG binding and utilized in this study to recognize BCG and trigger the BIF process. In order to confirm the hypothesis, the experimental peptide BFH was incubated with BCG and observed by transmission electron microscope (TEM) in 48 h. The BFH formed spherical NPs initially. After interacting with BCG, BFH NPs transformed into NFs in 24 h, and fibrous networks with a wide size distribution were clearly detectable in 48 h. The length and diameter of NFs became larger and larger over time in 48 h (Fig. 1d). For the control peptide BAH, it was found to be in the state of NPs in 48 h (Fig. S6, ESI†). In addition, circular dichroism (CD) spectra of BFH and BAH were in agreement with the TEM results. At the initial stage, BFH NPs did not show the obvious β-sheet secondary structure, which gradually formed with typical 203 nm (positive) and 212 nm (negative) peaks over time in 48 h. In contrast, the control BAH NPs did not show the β-sheet secondary structure in 48 h (Fig S7, ESI†). These results indicated that the binding of WHSGTPH to BCG could induce the fibrillogenesis, and the hydrogen-bonds provided by KLVFF were crucial for the transformation of NPs to NFs.
BFH NPs specially recognize BCG
The recognition ability of BFH and BAH NPs to BCG was first investigated by confocal laser scanning microscope (CLSM). The BCG was firstly stained by Texas red dye and then incubated with, PBS, BFH and BAH NPs in 500 μL of 0.9% saline for 4 h, respectively. Then, the BCG solution (10 μL) was suspended in physiological saline, which was further dropped on the center of the confocal Petri dish and observed by CLSM. In PBS group, BCG showed red fluorescence on BCG, indicating the successful staining (Fig. S8, ESI†). However, BFH and BAH NPs treated with BCG showed well-merged fluorescence of red and green signals (Fig. 2a and Fig. S9, ESI†). These results indicated that both BFH and BAH containing WHSGTPH sequence can specifically recognize BCG. However, the BFH group exhibited strong fluorescence with large area, but small area for BAH group. This may be due to fibrillogenesis of BFH NPs into fibrous networks after specifically recognizing and binding to BCG, leading to the agglutination of BCG. On the basis of this observation, the BCG actually can be detected with fluorescence and morphology by the fluorescent BFH peptide. In order to further confirm the specific recognition ability of BFH NPs, the mixture of BCG and S. aureus was incubated with BFH NPs. The green fluorescence of BFH was well merged with red fluorescence BCG to show yellow fluorescence from the mixture of both BCG and S. aureus (Fig. 2b). Meanwhile, there were no obvious fluorescence signals on S. aureus, indicating the specificity of BFH to BCG.
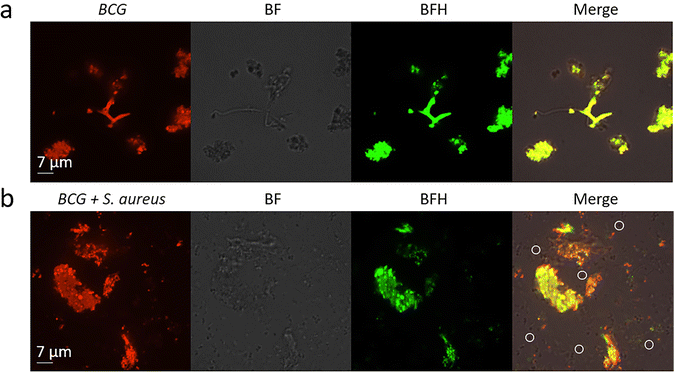 |
| Fig. 2 Specific recognition of BCG by BFH NPs. (a) CLSM images of Taxes red staining BCG (red channel) incubated with BFH NPs (green channel). The merged yellow color indicated the recognition of BCG by BFH NPs. (b) CLSM images of Taxes red staining BCG (red channel) mixed with S. aureus (no staining) co-incubated with BFH NPs (green channel). The merged yellow color indicated the recognition of BCG by BFH NPs. The S. aureus were not merged with BFH NPs (green channel), indicating the specific recognition of BFH NPs to BCG, not to S. aureus. Some S. aureus were highlighted by white circles. | |
The fibrillogenesis of BFH on the surface of BCG
BFH NPs can form NFs in situ after specific recognition of BCG (Fig. 3a). Therefore, the morphological changes of BFH and BAH NPs on the surface of BCG were further observed by bio-TEM. The BFH NPs (the experimental group) and BAH NPs (the control group) (3 × 10−5 M) were incubated with BCG for 4 h at 37 °C in a constant temperature incubator, respectively. After centrifugation, the BCG was washed with 0.9% saline for TEM measurement. In the experimental group, a large number of fibrous networks appeared around the BCG, validating the BIF ability of BFH. In the control group, scattered nanoparticles were found on the surface of BCG (Fig. 3b). Obviously, due to the conversion from BFH NPs to NFs, the adhesion of BFH on the surface of BCG far exceeded that of BAH, which can explain that BCG treated with BFH NPs had stronger fluorescence intensity than BAH NPs. These results verified that the recognition ability of the targeting peptide in BFH and BAH to BCG, and the importance of KLVFF for the conversion from NPs to NFs after recognition.
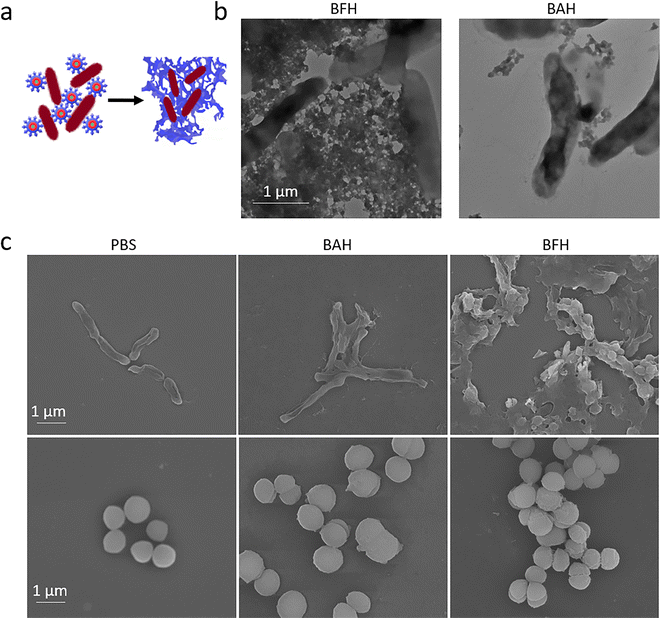 |
| Fig. 3 BFH NPs form fibrous network by binding to BCG. (a) Schematic diagram of BFH NPs specifically recognizing and forming fibrous networks to wrap BCG. (b) TEM images of BCG incubated with BFH forming fibrous network on BCG and BAH NPs without forming fibrous network on BCG. (c) SEM images of PBS, BFH and BAH NPs treated BCG (upper) and S. aureus (lower). There were fibrous networks on BCG and resultant agglutination of BCG. In contrast, No fibrous networks were observed on S. aureus and no obvious agglutination of S. aureus. | |
Scanning electron microscopy (SEM) studies was carried out on BCG incubated with PBS, BAH, and BFH NPs, respectively. PBS group hardly showed BCG aggregation and no fibrous network was found on the surface of BCG. BAH group only exhibited a slight aggregation of BCG, and almost no fibrous networks were formed on the surface of BCG. In contrast, there were not only large amount of BCG agglutination, but also dense fibrous networks entangled on the surface of BCG in BFH group. To further verify the specific binding of BFH to BCG, other bacilli, E. coli, was selected as a control for incubation with BFH. There were no fibrous networks on the surfaces of E. coli, and no agglutination of E. coli from SEM images (Fig. S10, ESI†). At the same time, PBS, BAH and BFH NPs were incubated with S. aureus, and observed by SEM. It was found that there were no entangled fibrous networks with S. aureus for all three groups, indicating no obvious interactions between the targeting peptide WHSGTPH and S. aureus (Fig. 3c).
BFH NPs defend against BCG invasion of macrophages
M. tuberculosis is an invasive bacteria that can bind to and invade into host cells (macrophages), causing the formation and spread of tuberculous granulomas. The fibrillogenesis of BFH NPs on the surface of BCG to form NFs was expected to trap and inhibit the invasion of BCG to host cells (Fig. 4a), which was evaluated by the trapping and invading experiment. In the blank group where only BCG and macrophages were incubated without any other treatment, a large amount of BCG was phagocytosed by macrophages (Fig. 4b). In contrast, In the control group, a lot of BCG (merged red and green channel) was observed inside the macrophages, even BCG were pre-treated and recognized by BAH NPs (Fig. S11, ESI†). However, once the BCG was pretreated with BFH NPs, BCG aggregated into larger BCG-BFH clump-like complexes outside the macrophages and there were almost no BCG were observed in macrophages (Fig. 4c). These results suggested that BFH NPs had a significant inhibitory effect on the phagocytosis of BCG by macrophages. We also carried out the toxicity test of BFH NPs against BCG, where BCG in logarithmic growth phase was incubated with BFH NPs for 24 h. It was found that no significant bacterial toxicity at concentrations up to 180 μM (Fig. S12, ESI†).
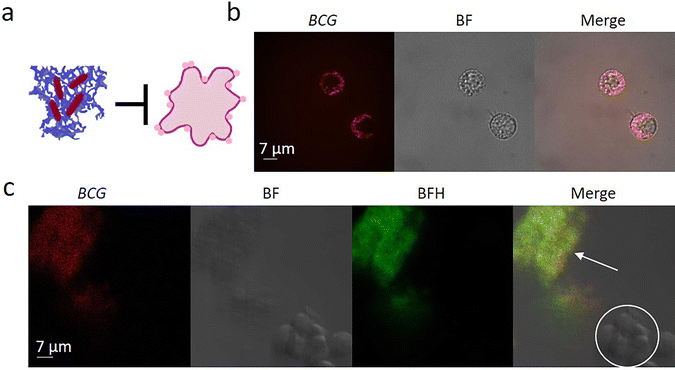 |
| Fig. 4 BFH NPs defend against BCG invasion of macrophages. (a) Schematic illustration of BCG captured by BFH fibrous networks preventing its phagocytosis by macrophages. (b) CLSM images of Taxes red staining BCG and macrophages without any treatment, indicating that phagocytosis of BCG (red channel) by macrophages. (c) CLSM images of co-incubation of BFH NPs, BCG and macrophages, indicating that BCG were captured by BFH to form BCG-BFH clump-like complexes (white arrow). The BCG phagocytosis by macrophages (white circle) was inhibited. | |
Despite the emerging novel technologies for diagnosis and treatment of tuberculosis in recent years, there's still a great challenge to completely overcome the disease.42 In the initial stage of tuberculosis infection, M. tuberculosis will be engulfed by macrophages and survive inside the phagosome.43 By inhibiting the acidification and maturation of phagolysosomes, it evades killing.44–46 Along with the necrosis of macrophages, M. tuberculosis will be released into extracellular space and induce the granuloma expansion.47,48 To make matters worse, the current anti-tuberculosis drugs can not effectively kill dormant M. tuberculosis,49,50 can not form higher drug concentrations inside macrophages, which makes it more difficult to completely kill M. tuberculosis.51,52 Therefore, a new type of intelligent peptide BFH, was designed, which can target and capture M. tuberculosis, forming nanofibrils on the surface of M. tuberculosis, and inhibiting the bacteria invasion into macrophages. Subsequently, the BFH may prevent the expansion of tuberculosis granulomas and make the extracellular M. tuberculosis more effectively killed by anti-tuberculosis drugs.
Conclusions
In this study, an intelligent peptide BFH with particle unit, fiber unit and recognition unit was designed and synthesized. Due to the strong hydrophobic effect of particle unit BP, the BFH can self-assemble into NPs in polar solvents. In addition, the fluorescent signal caused by the AIE effect of BP provides the possibility to detect the location of BFH. Fiber unit is KLVFF sequence from Aβ that folds a fibrous structure. The recognition unit is WHSGTPH sequence, which can target and bind to M. tuberculosis. It was found that the fluorescence intensity at 525 nm gradually increased with the increase of water content in the mixed solution, indicating the aggregation of BP, which induced the self-assembly of BFH. It was observed by TEM that the BFH were NPs at 0 h, and completely transformed into NFs in 48 h upon BCG incubation.
In order to verify its targeting effect of BFH to M. tuberculosis, we co-incubated the BFH NPs with BCG and verified by CLSM. The results showed that strong fluorescence signal completely cover the surface of bacteria, which confirmed the targeting ability of BFH to BCG. To further investigate whether the targeting peptide can induce the transformation of NPs into NFs on the bacterial surface, we co-incubated the BFH NPs with BCG and observed by SEM. The results revealed that BCG was covered and captured by fibrous networks, and agglomeration occurred.
In order to further evaluate the inhibition of BFH on phagocytosis of macrophages on BCG, we co-incubated BFH NPs, BCG and macrophages, and observed the effect of BFH on phagocytosis of BCG by macrophages through CLSM. The results showed that after incubation with BFH, the fluorescence intensity in macrophages was very low, and there were fluorescence signals outside cells. In the control group without BFH, a strong fluorescence signal was observed in macrophages, but no fluorescence signal was observed outside the cells. The above results showed that the BFH can self-assemble into entangled fibrous networks around the bacteria and gather the bacteria to increase their volume, thus inhibiting the phagocytosis of macrophages. We believed that this phenomenon would benefit effective killing of M. tuberculosis by drugs outside macrophages.
The limitation of this study was that only the phenomenon that the BFH inhibiting macrophage phagocytosis of BCG was observed. However, the specific mechanism of action was not clear. The follow-up study was planned to further study whether the above-mentioned phagocytosis inhibition can play a role in killing M. tuberculosis in vitro and in vivo, and to explore the relevant mechanisms.
Experimental
Materials and methods
The starting material BP-COOH was synthesized according to our previous report.41N,N-dimethylformamide (DMF) and dichloromethane need to be treated with molecular sieves before using. Methanol, N′-tetramethylurea hexafluorophosphate (HBTU), 4-methylmorpholine (NMM), trifluoroacetic acid (TFA) and O-(benzotriazol-1-yl) N,N,N′,N′ were purchased from Sigma-Aldrich Chemical Co. All Fmoc amino acids and Wang resins were obtained from GL Biochem (Shanghai) Ltd. The cell lines RAW 264.7 were purchased from the Cell Culture Center of the Institute of Basic Medical Sciences, Chinese Academy of Medical Sciences (Beijing, China). Fetal bovine serum and cell culture medium were purchased from Wisent Inc. (Multicell, Wisent Inc., St. Bruno, Quebec, Canada). BCG was purchased from Chengdu Institute of Biological Products Co., Ltd.
BCG culture and special staining.
Dissolve 60 mg of BCG in physiological saline with concentration 1 × 109 CFU per mL, then pipette 200 μL into neutral Roche medium, and place it in a 37 °C incubator for 3 weeks to facilitate bacterial growth. Dissolve 1 mg of Texas Red dye in 100 μL of anhydrous DMSO to a concentration of 10 mg mL−1 and store it in a −20 °C refrigerator. When performing BCG staining, BCG was diluted with physiological saline to the desired concentration and Texas red dye was added to make the final concentration 100 μg mL−1, then shake for 2 h and centrifuge at 10
000 rpm·for 5 minutes, Finally remove the supernatant.
Preparation of BFH and control molecule BAH.
The BFH and control BP-KAAGG-WHSGTPH (BAH) were synthesized by standard solid-phase peptide synthesis techniques using Fmoc-coupling chemistry. Piperidine (20% v/v) in anhydrous DMF was used as deprotection agents to the Fmoc group. Complete deprotection is verified by ninhydrin test (ninhydrin, phenol, VC 1
:
1:1 v/v). Then, add HBTU (137.2 mM) and Hobt activated amino acid (137.2 mM) to NMM (5%) anhydrous DMF. Repeat the appeal process until the BP-COOH was successfully coupled. Then, the final product was obtained by cleaving the resin from the resin for 3 h through a mixture of TFA (95%, volume percentage), triisopropylsilane (2.5%, volume percentage) and water (2.5%, volume percentage) in an ice bath. Suction the resin and lysate to obtain the filtrate, and blow with nitrogen to 1/10 of the total volume. An appropriate amount of glacial diethyl ether was added to the remaining filtrate to obtain a precipitated solid. The obtained solid was washed 3 times with glacial diethyl ether and dried in a vacuum oven to obtain the final product. The molecular structures of peptides were confirmed by MALDI-TOF-MS (Bruker Daltonics).
Preparation and characterization of BFH and BAH NPs.
BFH and BAH were separately added to DMSO and dissolved sufficiently to form a solution (3 mM). Pipette 20 μL of BFH or BAH solution into DMSO (880, 680, 480, 280, and 80 μL) to dilute and mix with deionized water (100, 300, 500, 700, and 900 μL). The UV-vis and fluorescence spectra of the resulting solutions with varying water were measured.
BFH and BAH NPs recognize bacteria by CLSM.
BCG and S. aureus were incubated with BFH and BAH NPs (30 μM) in 500 μL of 0.9% saline for 4 h, respectively. A separate BCG bacterial solution (without BFH and BAH NPs) was used as the blank group. After washing with 0.9% saline, remove 10 μL of bacterial solution and drop it on the confocal disc for CLSM measurement (Ultra-VIEW Vox, PerkinElmer).
BFH and BAH NPs in situ self-assemble into NFs on BCG by TEM.
The BCG and S. aureus was mixed with BFH NPs and BAH NPs, respectively, and then incubated in a constant temperature incubator for 4 h. The resulting samples were centrifuged at 10
000 rpm for 5 min in a centrifuge and the precipitated bacteria were washed for 3 times with 0.9% saline. The obtained bacteria were used for TEM measurements by a bio-TEM (HT7700 HITACHI TEM).
BFH and BAH NPs in situ trap BCG by SEM.
SEM was used to detect the in situ assembly of BFH NPs and BAH NPs on the surface of the two bacteria (BCG and S. aureus). First, BCG and S. aureus were incubated on silicon wafer in 0.9% saline, which was placed in 24-well plates overnight. One day later, the 0.9% saline was aspirated, the BFH and BAH NPs (30 μM, 1 mL) were added, and the final mixtures were incubated for 4 h. After removal of excess solution, the bacteria were solidified with glutaraldehyde (4%) overnight. Then, the samples were covered with gold for 2 min for SEM measurement (Hitachi-SU8220).
Macrophage resuscitation.
Frozen primary RAW264.7 cells were obtained from the liquid nitrogen tank, which were quickly thawed in a 37 °C water bath and transferred into a 15 mL centrifuge tube. After that, add 5 mL of serum-free RPMI-1640 medium and centrifuge at 1000 rpm·for 3 min, discarding the supernatant. The cells were suspended with appropriate amount of medium, and transferred to a 100 mm Petri dish, which were added with bovine serum/1640 medium at a ratio of 1
:
3 and placed at 37 °C in a 5% CO2 cell incubator. The medium was changed once in 1–2 days. After 3 generations, the experiment was performed after the cell status was stable.
BFH and BAH inhibit macrophage phagocytosis of BCG.
The cultured macrophages were manually counted to prepare 1 mL of 104 mL−1 cell suspension, which was added to the confocal dish. Aspirate the medium in the cell culture dish, wash the cells with 5 mL of phosphate buffer solution, then add 1 mL of trypsin to 37 °C for 3 min. After the cells are completely detached from the dish, rinse with 5 mL of culture medium to a suspended state. The cell suspension was transferred into a 15 mL centrifuge tube, centrifuge at 1000 rpm·for 3 min, pour out the upper layer, add 5 mL of medium. Pipette the cells to obtain a cell suspension, 10 μL of which was dropped into the counting plate and adjusted to the concentration of cells to 106 mL−1.
The frozen BCG was quickly thawed in a 37 °C water bath, and stain the BCG in the same way as above. The experimental group was co-incubated with macrophage suspension (500 μL), BCG (106 CFU per mL) and BFH NPs (30 μM). The control group was co-incubated with macrophages (500 μL), BCG (106 CFU per mL) and BAH NPs (30 μM). The blank control group was co-incubated with macrophage suspension (500 μL) and BCG (106 CFU per mL) without any nanomaterials. After 4 h, the suspension (10 μL) was dropped on the center of the confocal Petri dish and covered with a cover glass for CLSM measurement (Ultra-VIEWVox, PerkinElmer).
Conflicts of interest
There are no conflicts to declare.
Acknowledgements
This study is supported by the Strategic Priority Research Program of Chinese Academy of Sciences, Grant No. XDB36000000, the National Natural Science Foundation of China (81702174), and Hygiene and Health Development Scientific Research Fostering Plan of Haidian District Beijing, Grant No. HP2021-04-80501.
Notes and references
- D. Falzon, S. den Boon, A. Kanchar, M. Zignol, G. B. Migliori and T. Kasaeva, Eur. Respir. J., 2022, 59, 2102753 CrossRef PubMed.
- C. Jeremiah, E. Petersen, R. Nantanda, B. N. Mungai, G. B. Migliori, F. Amanullah, P. Lungu, F. Ntoumi, N. Kumarasamy, M. Maeurer and A. Zumla, Int. J. Infect. Dis., 2022 DOI:10.1016/j.ijid.2022.03.011.
- M. G. Moule and J. D. Cirillo, Front. Cell. Infect. Microbiol., 2020, 10, 65 CrossRef PubMed.
- E. Guirado, L. S. Schlesinger and G. Kaplan, Semin. Immunopathol., 2013, 35, 563–583 CrossRef CAS PubMed.
- Z. Huang, Q. Luo, Y. Guo, J. Chen, G. Xiong, Y. Peng, J. Ye and J. Li, PLoS One, 2015, 10, e0129744 CrossRef PubMed.
- M. Silva Miranda, A. Breiman, S. Allain, F. Deknuydt and F. Altare, Clin. Dev. Immunol., 2012, 2012, 139127 CrossRef PubMed.
- J. M. Davis and L. Ramakrishnan, Cell, 2009, 136, 37–49 CrossRef CAS PubMed.
- L. Ramakrishnan, Nat. Rev. Immunol., 2012, 12, 352–366 CrossRef CAS PubMed.
- E. J. Rubin, N. Engl. J. Med., 2009, 360, 2471–2473 CrossRef CAS PubMed.
- T. D. Bold and J. D. Ernst, Cell, 2009, 136, 17–19 CrossRef CAS PubMed.
- T. Ulrichs and S. H. Kaufmann, J. Pathol., 2006, 208, 261–269 CrossRef CAS PubMed.
- K. Borah, Y. Xu and J. McFadden, Front. Immunol., 2021, 12, 762315 CrossRef CAS PubMed.
- C. L. Cosma, D. R. Sherman and L. Ramakrishnan, Annu. Rev. Microbiol., 2003, 57, 641–676 CrossRef CAS PubMed.
- K. Rohde, R. M. Yates, G. E. Purdy and D. G. Russell, Immunol. Rev., 2007, 219, 37–54 CrossRef CAS PubMed.
- C. M. McClean and D. M. Tobin, Pathog. Dis., 2016, 74, ftw068 CrossRef PubMed.
- H. E. Volkman, T. C. Pozos, J. Zheng, J. M. Davis, J. F. Rawls and L. Ramakrishnan, Science, 2010, 327, 466–469 CrossRef CAS PubMed.
- J. M. Davis, H. Clay, J. L. Lewis, N. Ghori, P. Herbomel and L. Ramakrishnan, Immunity, 2002, 17, 693–702 CrossRef CAS PubMed.
- H. E. Volkman, H. Clay, D. Beery, J. C. Chang, D. R. Sherman and L. Ramakrishnan, PLoS Biol., 2004, 2, e367 CrossRef PubMed.
- P. J. Cardona, Enferm. Infecc. Microbiol. Clin., 2018, 36, 38–46 CrossRef PubMed.
- L. Ramakrishnan, Adv. Exp. Med. Biol., 2013, 783, 251–266 CrossRef PubMed.
- H. Clay, H. E. Volkman and L. Ramakrishnan, Immunity, 2008, 29, 283–294 CrossRef CAS PubMed.
- L. Zhang, Q. Cheng, C. Li, X. Zeng and X. Z. Zhang, Biomaterials, 2020, 248, 120029 CrossRef CAS PubMed.
- C. Xu, Z. Lu, Y. Luo, Y. Liu, Z. Cao, S. Shen, H. Li, J. Liu, K. Chen, Z. Chen, X. Yang, Z. Gu and J. Wang, Nat. Commun., 2018, 9, 4092 CrossRef PubMed.
- F. H. Ma, Y. An, J. Wang, Y. Song, Y. Liu and L. Shi, ACS Nano, 2017, 11, 10549–10557 CrossRef CAS PubMed.
- Y. Zeng, J. Wang, Z. Wang, G. Chen, J. Yu, S. Li, Q. Li, H. Li, D. Wen, Z. Gu and Z. Gu, Nano Today, 2020, 35, 100984 CrossRef CAS.
- Y. Zhang, K. Cai, C. Li, Q. Guo, Q. Chen, X. He, L. Liu, Y. Zhang, Y. Lu, X. Chen, T. Sun, Y. Huang, J. Cheng and C. Jiang, Nano Lett., 2018, 18, 1908–1915 CrossRef CAS PubMed.
- P. Zhang, Y. Zhang, X. Ding, W. Shen, M. Li, E. Wagner, C. Xiao and X. Chen, Adv. Mater., 2020, 32, e2000013 CrossRef PubMed.
- J. M. Lehn, Science, 1993, 260, 1762–1763 CrossRef CAS PubMed.
- G. V. Oshovsky, D. N. Reinhoudt and W. Verboom, Angew. Chem., Int. Ed., 2007, 46, 2366–2393 CrossRef CAS PubMed.
- K. Zhang, P. P. Yang, P. P. He, S. F. Wen, X. R. Zou, Y. Fan, Z. M. Chen, H. Cao, Z. Yang, K. Yue, X. Zhang, H. Zhang, L. Wang and H. Wang, ACS Nano, 2020, 14, 7170–7180 CrossRef CAS PubMed.
- P. P. Yang, Y. J. Li, Y. Cao, L. Zhang, J. Q. Wang, Z. Lai, K. Zhang, D. Shorty, W. Xiao, H. Cao, L. Wang, H. Wang, R. Liu and K. S. Lam, Nat. Commun., 2021, 12, 4494 CrossRef CAS PubMed.
- J. Q. Fan, Y. J. Li, Z. J. Wei, Y. Fan, X. D. Li, Z. M. Chen, D. Y. Hou, W. Y. Xiao, M. R. Ding, H. Wang and L. Wang, Nano Lett., 2021, 21, 6202–6210 CrossRef CAS PubMed.
- P.-P. He, X.-D. Li, J.-Q. Fan, Y. Fan, P.-P. Yang, B.-N. Li, Y. Cong, C. Yang, K. Zhang, Z.-Q. Wang, D.-Y. Hou, H. Wang and L. Wang, CCS Chem., 2020, 2, 539–554 CrossRef CAS.
- J. Fan, Y. Fan, Z. Wei, Y. Li, X. Li, L. Wang and H. Wang, Chin. Chem. Lett., 2020, 31, 1787–1791 CrossRef CAS.
- Z. Chen, K. Zhang, J. Fan, Y. Fan, C. Yang, W. Tian, Y. Li, W. Li, J. Zhang, H. Wang and L. Wang, Chin. Chem. Lett., 2020, 31, 3107–3112 CrossRef CAS.
- P. P. He, X. D. Li, L. Wang and H. Wang, Acc. Chem. Res., 2019, 52, 367–378 CrossRef CAS PubMed.
- G. B. Qi, Y. J. Gao, L. Wang and H. Wang, Adv. Mater., 2018, 30, e1703444 CrossRef PubMed.
- Y. Fan, X. D. Li, P. P. He, X. X. Hu, K. Zhang, J. Q. Fan, P. P. Yang, H. Y. Zheng, W. Tian, Z. M. Chen, L. Ji, H. Wang and L. Wang, Sci. Adv., 2020, 6, eaaz4767 CrossRef CAS PubMed.
- H. Yang, L. Qin, Y. Wang, B. Zhang, Z. Liu, H. Ma, J. Lu, X. Huang, D. Shi and Z. Hu, Int. J. Nanomed., 2015, 10, 77–88 Search PubMed.
- V. Castelletto, P. Ryumin, R. Cramer, I. W. Hamley, M. Taylor, D. Allsop, M. Reza, J. Ruokolainen, T. Arnold, D. Hermida-Merino, C. I. Garcia, M. C. Leal and E. Castaño, Sci. Rep., 2017, 7, 43637 CrossRef CAS PubMed.
- L. Wang, W. Li, J. Lu, Y.-X. Zhao, G. Fan, J.-P. Zhang and H. Wang, J. Phys. Chem. C, 2013, 117, 26811–26820 CrossRef CAS.
- C. M. Gill, L. Dolan, L. M. Piggott and A. M. McLaughlin, Breathe, 2022, 18, 210149 CrossRef PubMed.
- D. Mahamed, M. Boulle, Y. Ganga, C. Mc Arthur, S. Skroch, L. Oom, O. Catinas, K. Pillay, M. Naicker, S. Rampersad, C. Mathonsi, J. Hunter, E. B. Wong, M. Suleman, G. Sreejit, A. S. Pym, G. Lustig and A. Sigal, eLife, 2017, 6, 22028 CrossRef PubMed.
- I. Vergne, J. Chua, H. H. Lee, M. Lucas, J. Belisle and V. Deretic, Proc. Natl. Acad. Sci. U. S. A., 2005, 102, 4033–4038 CrossRef CAS PubMed.
- G. Arora, R. Misra and A. Sajid, Curr. Top. Med. Chem., 2017, 17, 2077–2099 CrossRef CAS PubMed.
- R. Domingo-Gonzalez, O. Prince, A. Cooper and S. A. Khader, Microbiol. Spectr., 2016, 4, TBTB2-0018-2016 Search PubMed.
- A. J. Pagán and L. Ramakrishnan, Cold Spring Harbor Perspect. Med., 2014, 5, a018499 CrossRef PubMed.
- P. J. Cardona, Inflammation Allergy: Drug Targets, 2007, 6, 27–39 CrossRef CAS PubMed.
- M. Gengenbacher and S. H. Kaufmann, FEMS Microbiol. Rev., 2012, 36, 514–532 CrossRef CAS PubMed.
- R. M. McCune, F. M. Feldmann, H. P. Lambert and W. McDermott, J. Exp. Med., 1966, 123, 445–468 CrossRef CAS PubMed.
- K. Schaaf, V. Hayley, A. Speer, F. Wolschendorf, M. Niederweis, O. Kutsch and J. Sun, Assay Drug Dev. Technol., 2016, 14, 345–354 CrossRef CAS PubMed.
- A. Gupta, A. Kaul, A. G. Tsolaki, U. Kishore and S. Bhakta, Immunobiology, 2012, 217, 363–374 CrossRef CAS PubMed.
|
This journal is © The Royal Society of Chemistry 2023 |
Click here to see how this site uses Cookies. View our privacy policy here.