DOI:
10.1039/D2TB02319A
(Review Article)
J. Mater. Chem. B, 2023,
11, 482-499
ROS-scavenging biomaterials for periodontitis†
Received
25th October 2022
, Accepted 18th November 2022
First published on 21st November 2022
Abstract
Periodontitis is defined as a chronic inflammatory disease in which the continuous activation of oxidative stress surpasses the reactive oxygen species (ROS) scavenging capacity of the endogenous antioxidative defense system. Studies have demonstrated that ROS-scavenging biomaterials should be promising candidates for periodontitis therapy. To benefit the understanding and design of scavenging biomaterials for periodontitis, this review details the relationship between ROS and periodontitis, including direct and indirect damage, the application of ROS-scavenging biomaterials in periodontitis, including organic and inorganic ROS-scavenging biomaterials, and the various dosage forms of fabricated materials currently used for periodontal therapy. Finally, the current situation and further prospects of ROS-scavenging biomaterials in periodontal applications are summarized. Expecting that improved ROS-scavenging biomaterials could be better designed and developed for periodontal and even clinical application.
1. Introduction
Periodontitis with its very high incidence is a chronic inflammatory disease caused by bacterial infection.1 Serious periodontitis may not only cause tooth loss, but can also affect systemic health by increasing the patients' risk of cardiovascular disease,2 pulmonary disease3 and cancer.4 Conversely, some systemic diseases (such as diabetes), as well as genetic factors, changes in hormone levels, smoking, etc. can also induce periodontitis and accelerate the destruction of periodontal tissue.5 As the sixth largest public health problem in the world, severe periodontitis affects 10–15% of the world's population, and has been recognized as the most frequent cause of tooth loss in adults.6–8 As a result, there is an urgent need for the development of approaches that are efficient and affordable for the prevention and treatment of periodontal disease. Throughout history, people have utilized various strategies for the treatment of periodontitis,9 including mechanical debridement of dental plaque, antibiotics and anti-inflammatory drugs to treat pathogenic infections, as well as regenerative surgery for severely affected patients.10 All these methods have relieved the symptoms of periodontitis and achieved positive results. However, with the most basic and main traditional treatment method, mechanical debridement, it is difficult to reach and eliminate deep-seated bacteria, and the treatment process is painful and time-consuming. In addition, the permeability, safety and drug resistance of drugs and the risks of surgery have seriously limited the clinical efficacy of traditional periodontal treatment methods.9,11 In order to overcome the shortcomings of traditional therapeutic methods, it is necessary to develop advanced treatment strategies for periodontitis.9
A number of studies have proved that ROS play a significant role in the pathogenesis of many complicated illnesses, such as cardiovascular diseases, cancer, neurodegenerative diseases, respiratory diseases and periodontal diseases.12,13 Under normal physiological conditions, ROS released by the respiratory chain and most enzymatic reactions are in balance with the endogenous antioxidative defense system, which is essential for various biological processes such as intracellular signal transduction, gene expression regulation and antibacterial defense.14,15 In contrast, in a long-term inflammatory environment, the excessive-production of ROS can cause a variety of adverse reactions, causing intractable damage by inducing oxidative stress.16 Excessive ROS produced in chronic periodontitis can directly destroy biological macromolecules of cells via lipid peroxidation, protein denaturation, and DNA damage, thereby interfering with cell growth and periodic progress, and causing damage to the host periodontal tissue.17,18 In addition, oxidative stress can also aggravate periodontitis by regulating signal transduction and gene transcription, promoting the production and expression of pro-inflammatory cytokines and chemokines, or inhibiting the function of antioxidant defense genes.19 Significantly, direct and indirect damage are the main causes of the occurrence and development of periodontitis.20 Massive studies have confirmed that efficient local antioxidant applications could change the periodontal microenvironment and reduce the pathological process of periodontal inflammation.20–22 Therefore, oxidative regulation has been employed as a promising strategy for severe periodontitis therapy in recent years and has become one of the hotspots in current research fields.
As the cross product of life science and materials science, developing well in recent years, biomaterials have been endowed with the functions of diagnosis, treatment, replacement of tissues and organs in vivo, and the promotion of functional recovery. Scientists have endowed these biomaterials with lots of excellent biological properties. Biomaterials with antibacterial, inflammation regulation and ROS-scavenging effects shine brightly in the biomedical field and have comparably great application potential in the treatment of periodontitis. ROS-scavenging biomaterials are a subset of antioxidants,23 and various forms (such as molecules,16 polymers,24,25 capsules,26 nanoparticles20 and hydrogels23,27,28) of ROS-scavenging biomaterials can adapt well to the different forms of tissue destruction in periodontitis. Fabricated biomaterials which possess promising properties—including improved biocompatibility, extended action time, enhanced bioavailability and controlled release of drugs—endow ROS-scavenging biomaterials with great potential in the treatment of periodontitis. Therefore, this review strives to focus on the role of ROS in periodontitis, mainly the mechanism of ROS in the occurrence and development of periodontitis. Then, the classification of ROS-scavenging biomaterials from organic and inorganic aspects is introduced, and design methods, structural forms and dosage forms of ROS-scavenging biomaterials currently used in periodontal therapy have been extensively illustrated. Finally, the advantages and challenges of periodontitis adjuvant antioxidant therapy are summarized, and prospects are provided for follow-up research and clinical applications (Fig. 1).
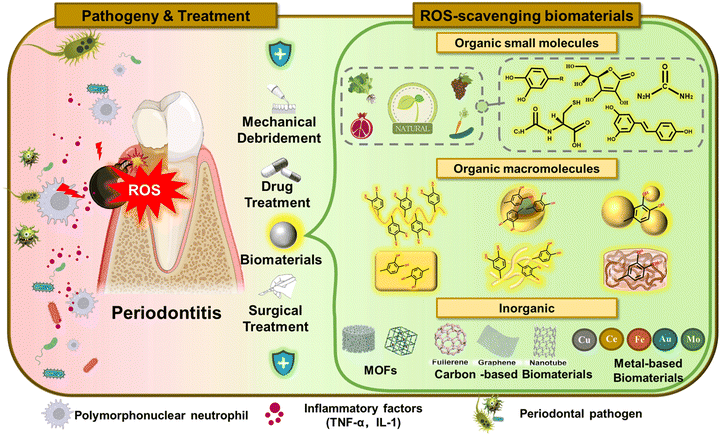 |
| Fig. 1 Overview of the schematic illustration of ROS-scavenging biomaterial for periodontitis. | |
2. The role of ROS in periodontitis
ROS, a double-edged sword, are essential for the normal physiological activities of the body in physiological states such as antibacterial, defense, immune regulation and signal transduction of periodontal tissue.29,30 Generally, ROS exist at picomolar to nanomolar concentrations, and their lifespan ranges between nanoseconds and seconds.31 Although ROS were previously considered to play a harmful role in destroying normal physiological activities, the loss of ROS may also affect tissue repair as they are indispensable signalling molecules.26,32 Thus, the antioxidative therapy for periodontitis should regulate ROS levels rather than thoroughly eliminate ROS, and the final state of ROS levels should match the endogenous oxidative regulating levels which ensure the proliferation, differentiation and regeneration of tissues.31,32 Normally, there is a dynamic balance between ROS production and endogenous antioxidative scavenging.33 However, low immune function or persistent pathogen stimulation can result in prolonged activation of the immune system, excessive ROS production, and disruption of oxidative balance.16 Then, excessive ROS production can cause damage to various cellular biomacromolecules such as nucleic acid, proteins and lipids.34 Accumulating evidence has shown that ROS overproduction induced oxidative stress is closely related to the occurrence and development of many diseases, such as cancer, coronary atherosclerosis, and rheumatoid arthritis, and periodontitis is no exception.11 Gram-negative anaerobe and dental plaque biofilm in the subgingival region are the initiating factors of periodontal disease, but their pathogenicity is not enough. The imbalance between pathogenic microorganisms and host immune response is the main reason for the progression of periodontitis.16,19 In the process of periodontal disease development, a large number of polymorphonuclear neutrophils (PMN) in the damaged area are recruited and activated when periodontal pathogens invade in the early stage. As the first defense barrier of the body, PMN can eliminate periodontal pathogens by releasing ROS, which belong to the inflammatory process of the body's normal immune response. However, due to long-term chronic inflammatory stimulation during periodontitis, ROS produced by immune cells while killing pathogenic microorganisms destroy the redox homeostasis, and excessive ROS are released through the mitochondrial electron transport chain and a series of enzyme reactions to induce oxidative stress, which directly or indirectly leads to irreversible damage to periodontal tissues and cells.11,14 In this section, the role of ROS in periodontitis is described in detail (Fig. 2).
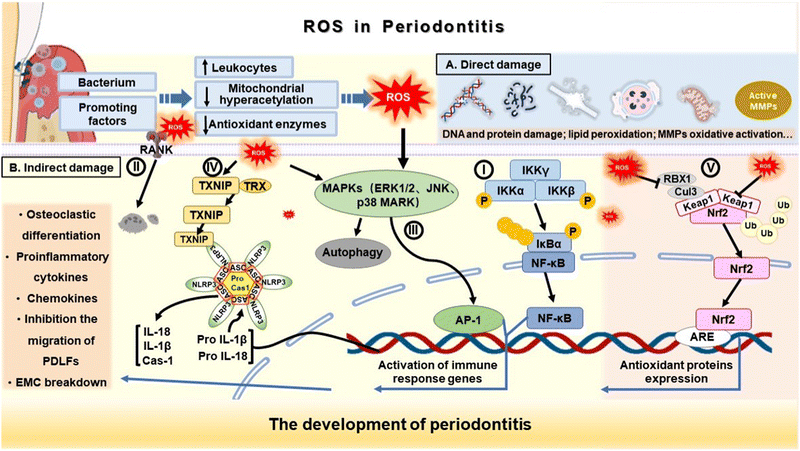 |
| Fig. 2 Schematic representation of the underlying pathways by which ROS-induced oxidative stress regulates inflammation, contributing to the progression of periodontal disease. | |
Direct tissue damage induced by oxidative stress at the level of biological macromolecules and cells can be mediated in the following ways: (1) matrix metalloproteinases (MMPs) oxidative activation and degradation of the extracellular matrix (ECM) components; (2) lipid peroxidation and cell membrane destruction; (3) DNA and protein damage; and (4) mitochondria injury and respiratory burst (Fig. 2A).35,36 Additionally, excess ROS can cause indirect damage to periodontal tissue by activating or inhibiting signaling pathways and transcription factors that regulate the immune inflammatory response.
First, excessive ROS can upregulate multiple inflammation related signal pathways to aggravate periodontal inflammation. For example, activation of the nuclear factor κ-light-chain-enhancer of activated B cells (NF-κB) signaling pathway promotes the movement of NF-κB dimers to the nucleus through phosphorylation and ubiquitination of the nuclear factor of κ-light polypeptide gene enhancer in B-cells inhibitor α (Iκ-Bα) to initiate transcription of multiple genes,37,38 resulting in the expression of pro-inflammatory cytokines, chemokines, and MMPs along with other inflammatory mediators (Fig. 2B(I)).16
The receptor activator of nuclear factor-κB ligand (RANKL) signaling pathway is activated to participate in osteoclast maturation and differentiation under a long-term inflammatory microenvironment, leading to osseous tissue destruction (Fig. 2B(II)).14,19,39 In addition, ROS activates c-Jun N-terminal kinase (JNK) and p38 MAP kinase of the mitogen-activated protein kinase (MAPKs) family.40 As oxidative stress-activated protein kinases, JNK and p38 can induce cell apoptosis, and destroy the periodontal junction epithelium through e-mucin isolation, which may play a role in the pathogenesis of periodontal disease (Fig. 2B(III)).16,41 The overproduction of ROS and oxidative stress not only promote the release and aggregation of pro-inflammatory cytokines and mediators by upregulating the expression of inflammation-related signaling pathways but also aggravate periodontal tissue damage through the assembly of inflammasome.16 NOD-like receptor protein 3 (NLRP3) inflammasome is a cytoplasmic multiprotein complex consisting of NLRP3, ASC (apoptosis-associated speck-like protein containing a CARD), and pro-caspase-1 (the effector cysteine protease caspase 1).18 Studies have shown that the increased expression of interleukin 1β (IL-1β) and interleukin 18 (IL-18) in the gingival tissue and crevicular fluid of patients with different types of periodontal disease is positively correlated with the increased expression of NLRP3 mRNA in the oral epithelium and saliva of patients with periodontal disease.16,42 The NLRP3 inflammasome activated by a two-step process induces maturation and secretion of the pro-inflammatory cytokines IL-1β and IL-18 through cleavage of caspase-1 (Fig. 2B(IV)).42,43 This can inhibit the migration of periodontal membrane fibroblasts, damage the periodontal ligament, and lead to alveolar bone destruction. This suggests that NLRP3 inflammasome may be involved in the pathogenesis of periodontitis.
In addition to the activating aspect, oxidative stress can inhibit the transcription and expression of key factors related to periodontal disease toleration.44 Nuclear factor red line 2 related factor 2 (Nrf2) is a basic leucine zipper transcription factor.16 The Nrf2-ARE signaling pathway strongly regulates the expression of endogenous antioxidative oxidase genes. Under physiological conditions, Nrf2 in the cytoplasm binds to the Keap1 protein, which acts as a mediator of Nrf2 degradation, maintaining acceptable levels of Nrf2 and preventing unnecessary transcription of antioxidative genes. In contrast, the primary transcription factor Nrf2 dissociates from Keap1 under oxidative stress and could be transferred to the nucleus, where it binds to the antioxidant responsive element (ARE) region containing promoters and upregulates relevant endogenous antioxidants, providing important protective effects, such as reducing inflammatory signaling pathways and oxidative damage in periodontal tissue (Fig. 2B(V)).41 However, Sima et al. found that 24 genes in the Nrf2-mediated oxidative stress response pathway were downregulated and antioxidative production was reduced in oral PMN (oPMN) in patients with chronic periodontitis who did not respond to conventional therapy.44 These findings suggest that excessive oxidative stress could weaken the protective effect of the Nrf2-ARE signaling pathway.
Finally, oxidative stress can significantly increase the expression of dynamic-related protein-1 (Drp1), an important regulator of mitochondrial division, which can cause a series of mitochondrial dysfunctions, such as abnormal mitochondrial membrane potential and reduced ATP level.18 Shi et al. showed that phosphorylated Drp1 (P-Drp1) levels were significantly elevated in patients with periodontitis, while ROS inhibitors or mitochondrion inhibitors can effectively reverse the expression of Drp1.45 These results suggest that ROS-Drp1 interaction may also be involved in the progression of periodontal disease.18 Taken together, the activation of NF-κB signaling pathway, RANKL signaling pathway, JNK kinases of the MAPK family, NLRP3 inflammasomes, and the inhibition of periodontitis tolerance-related factors (Nrf2) during chronic inflammatory oxidative stress, play an important role in the destruction and repair of periodontal tissue. Therefore, scholars regard the treatment of oxidative stress as a therapeutic target to treat periodontitis. Regulating ROS levels by ROS-scavenging biomaterials has gradually been recognized as a potentially effective means to prevent and treat periodontitis, which will be discussed in the following section.
3. ROS-scavenging biomaterial for periodontitis
ROS-scavenging biomaterials are defined as “any natural or man-made materials capable of preventing, reducing or eliminating oxidative damage to a target”, with a wide range of uses and types.23 Based on the close relationship between ROS and periodontitis, ROS-scavenging biomaterials used to improve the oxidative stress microenvironment of periodontitis have been widely studied.11,38,46 ROS-scavenging biomaterials can be simply divided into two categories: organic and inorganic materials. Organic materials can be divided into organic small-molecular and organic macromolecular materials, which are discussed in detail in the following sections.47 In addition, design methods and application effects of ROS-scavenging biomaterials in periodontal therapies will also be introduced and discussed in the following sections.
3.1. Organic ROS-scavenging biomaterial
3.1.1. Organic small molecular ROS-scavenging biomaterial.
Organic small molecule ROS-scavenging biomaterials, due to their widespread sources and easy availability, were used very early in biological health care, clinical treatment and other biomedical fields,16 including natural and synthetic materials. As early as 1980, natural organic small molecule ROS-scavenging biomaterials had appeared.48,49 Natural ROS-scavenging small molecules found in a variety of fruits, vegetables, herbs, teas and nutrients have become a key source of exogenous antioxidants, with a unique chemical structure which gives them natural antioxidant capacity, such as polyphenols, vitamin A, C, E and coenzyme Q10 (CoQ10) (Fig. 3A).13 Among them, several kinds of small molecule ROS-scavenging biomaterials have been approved by the Food and Drug Administration (FDA) that can be used to treat oxidative stress-related diseases include catechins, baicalein, vitamin E, C, CoQ10, edaravone, N-acetylcysteine (NAC), O-(β-hydroxyethyl)-rutosides, etc.23,50 In addition to natural organic small molecule ROS-scavenging biomaterials, to enhance or supplement them with isolated components, people have developed many synthetic organic small molecule ROS-scavenging biomaterials, such as butylated hydroxyanisole (BHA), butylated hydroxytoluene (BH), butylated hydroxymethyl toluene (BHT) and their analogues.35 However, due to the inevitable liver and kidney damage and their potential as carcinogens,51 their further clinical development is severely limited. At present, they are mainly used to preserve the bloom, nutritional importance, flavor and color of food products.48 Biomaterials used in the biomedical field should be greener and more natural. Polyphenols and their derivatives, composed of at least one benzene ring and one or more phenolic hydroxyl groups, are the most representative natural organic small molecule ROS-scavenging biomaterials.52 The most crucial signature of polyphenolic materials is the presence of catechol or pyrogallol groups on the benzene rings, which provide them with rich intermolecular interactions, including covalent interactions (such as Michael addition/Schiff base reactions, radical coupling reactions, polyphenol-metal coordination and polyphenol-boronate complexation) and noncovalent interactions (such as hydrogen, electrostatic interaction, cationic–π and π–π electron interactions) (Fig. 3B).52 In addition, the presence of R groups on benzene rings, such as amino and carboxyl groups, provide rich active centers, creating conditions for the further utilization of polyphenols (modification, coordination, and copolymerization).53 Because of these fascinating structural features, the application of polyphenols in inflammatory periodontal destruction are being increasingly studied with interest. Lee et al.54 demonstrated that injection of genistein can inhibit host immune inflammatory induction, prevent further degradation of periodontal tissue, and reduce osteoclast response to oral bacterial pathogens in a mouse model of periodontitis. The underlying mechanism may be that genistein significantly inhibits the differentiation of RANKL or macrophages into osteoclasts by inhibiting the expression of osteoclast specific molecules. In addition, genistein protects human gingival fibroblasts (HGFs) from lipopolysaccharide (LPS)-mediated oxidative stress damage. Similarly, Xu's research group verified that rutin, a typical flavonoid, can improve the properties of periodontal ligament stem cells (PDLSCs) in the inflammatory environment.55 10 μM rutin has the strongest ROS-scavenging effect, which can enhance the antioxidative ability of PDLSCs in the LPS-induced inflammatory environment and promote the proliferation and osteogenic differentiation ability of PDLSCs, through the phosphatidylinositol 3-kinase (PI3K)/AKT pathway (Fig. 3C). This is consistent with previous studies.56 While regulating the local oxidative stress microenvironment, studies revealed that periodontal pathogens and dental plaque biofilm should also be effectively inhibited and removed. He et al. found that resveratrol could inhibit the formation of Fusobacterium nuclear biofilm, which contributed to the electrostatic repulsion and the change in hydrophilicity of the biofilm surface.57,58 However, natural dental plaque biofilms possess complex structures of multiple organisms, which may perform quite differently from monoculture biofilms in a study. Mature dental plaque biofilms have a typical anoxic microenvironment.59 At present, ROS-based therapies including photodynamic therapy (PDT), sonodynamic therapy (SDT), and enzyme-like catalytic therapy are attractive, playing the role of an antibacterial and anti-biofilm by producing ROS.60 However, the oxidative balance should be kept after effectively eliminating biofilms, and the excess ROS should be regulated as soon as possible. Other natural phenolic compounds, such as quercetin,61 curcumin62 and grape seed extract50 have also been shown to be effective in the treatment and repair of experimental periodontal tissue injury. Therefore, polyphenols, a promising biomaterial, should be constantly explored for periodontitis engineering applications in the future.
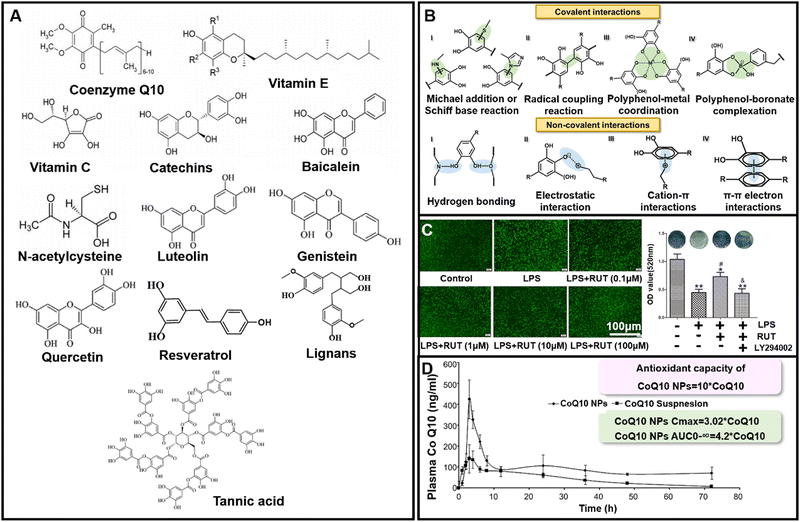 |
| Fig. 3 Chemical structure and application of organic small molecule ROS-scavenging biomaterials. (A) Chemical structure of typical polyphenols. (B) Intermolecular interactions of phenolic compounds. (C) Schematic illustration of rutin's antioxidant capacity and its promotion of bone formation. Reproduced with permission from ref. 55, Copyright Springer Nature B.V. 2020. (D) Schematic illustration of antioxidant capacity and bioavailability of CoQ10 NPs. Reproduced with permission from ref. 68, Copyright Elsevier 2011. | |
Other small molecule ROS-scavenging biomaterials, such as vitamins, in the antioxidative defense system also have excellent antioxidative properties, and they have been applied to treat periodontal disease.63–67 CoQ10, with a structure similar to vitamin K, is contained in the mitochondria of all human cells, and it has many important functions such as antioxidative capacity, regulation of cell growth and death.63 Studies have shown that oral CoQ10 can efficiently inhibit periodontitis by increasing the concentrations of CoQ10 in gingiva, which may be related to the ROS-scavenging activity of CoQ10.63 Swarnakar et al. enhanced the bioactive therapeutic effects by loading CoQ10 onto poly(lactic-co-glycolic acid) (PLGA) nanoparticles. The freeze-dried CoQ10 nanoparticles (CoQ10 NPs) are stable at room temperature for 6 months. The anti-ROS activity of CoQ10 in vitro is nearly 10 times higher than that of free CoQ10.68 Additionally, by comparing the pharmacokinetic parameters after a single oral administration of CoQ10 NPs and free CoQ10 suspension, according to the analysis and calculation of plasma concentration curve and experimental data, the peak plasma concentration (Cmax) and oral bioavailability (AUC0–∞, from time 0 to ∞ under the concentration time curve, reflecting the total amount of drugs entering the blood circulation) of CoQ10 NPs increased by 3.02 times and 4.2 times, respectively, compared with free CoQ10. CoQ10 NPs also showed remarkable liver protection and anti-inflammatory activities in vivo (Fig. 3D). This study makes CoQ10 and its derivatives more suitable for the treatment of oxidative stress induced diseases.
The examples mentioned above clearly prove the application potential of organic small molecule ROS-scavenging biomaterials in periodontal treatment. However, small molecule ROS-scavenging biomaterials have inevitable disadvantages, including low solubility and poor stability in the gastrointestinal tract, resulting in a short cycle time and low bioavailability, making it difficult for them to achieve sustainable antioxidative effects. High doses may lead to systemic toxicity, causing a series of adverse reactions, which limits their applications in biomedicine.12,47 Macromolecular engineering has been developed as a promising strategy to address the limitations of small molecule ROS-scavenging biomaterials, including nanotechnology, bionic design, polymerization or combination with polymers, hoping to enhance ROS-scavenging effects, while improving the long course of treatment and poor efficacy of many inflammatory diseases.28,47
3.1.2. Organic macromolecular ROS-scavenging biomaterials.
Considering the intrinsic defects of traditional small-molecule ROS scavengers, organic macromolecular ROS-scavenging biomaterials may accelerate the further development of ROS scavengers. Although many organic macromolecular ROS scavengers (e.g. the antioxidant defense system of endogenous enzymes, proteins and polysaccharides) exist in nature,69 they have few sources and are difficult to extract and separate.12,70 These natural macromolecular ROS-scavenging biomaterials have strict requirements on the application environment and are prone to denaturation and inactivation in the face in changes of external temperature and pH.69,71 Therefore, researchers have turned their attention to synthetic organic macromolecular ROS-scavenging biomaterials,12 which have not only achieved controlled release and targeted release but also improved the stability and bioavailability of small molecule ROS scavengers,70 greatly reduced the dosage, and avoided the occurrence of adverse effects.23,47 In the following they will be discussed in detail according to the different preparation strategies of organic macromolecular ROS-scavenging biomaterials.12,23,47
(1) Delivery strategies.
Focusing on small molecular ROS scavengers themselves, through simple loading or encapsulating technologies, nanomaterials could be utilized as delivery platforms for functional delivery. Polyphenol coatings on nanomaterials have been actively studied. Polyphenols can act as “polydentate” ligands to provide strong metal chelation effects. Thus, polyphenols can chelate a variety of metal ions to form different polyphenol-metal coordination complexes. The metal phenolic network (MPN) is a typical example.72 An MPN can be formed on the surface of various types of materials, and is a convenient and effective coating;73 it is also accompanied by a series of excellent biological properties, including anti-inflammatory, anti-cancer and antioxidative properties.72 Lee et al. used EGCG as a polyphenol composite and constructed MPN coating on a polycaprolactone (PCL) membrane and poly(L-lactic acid) (PLLA) fiber by direct immersion incubation.74 This study confirmed that the total phenol content (TCP) on the surface of the MPN-PCL membrane can be maintained at 60–80% within 7 days, effectively extending the retention time of EGCG. Moreover, EGCG can react with free radical species by resonance stabilization, such as highly active ˙OH formed by the decomposition of H2O2 under physiological conditions. Hence, it can better protect experimental cells from H2O2-mediated ROS damage and promote tissue regeneration under 2D or 3D culture conditions74 (Fig. 4A). Polyphenols can serve as biomimetic anchors/primers, while their derivatives and conjugates can also be immobilized on the substrate surfaces to graft various interesting molecules. Zhang et al. constructed an MPN coating on a porous poly(DL-lactide) (PPLA) scaffold by using a layer-by-layer (LBL) technique and loaded the active factor bone morphogenetic protein 2 (BMP-2).75 The MPN coating simultaneously played an interface gating role, acting as a physical “gate” through which the BMP-2 passed, achieving the controlled release of drugs and overlapping the time of natural bone repair.75
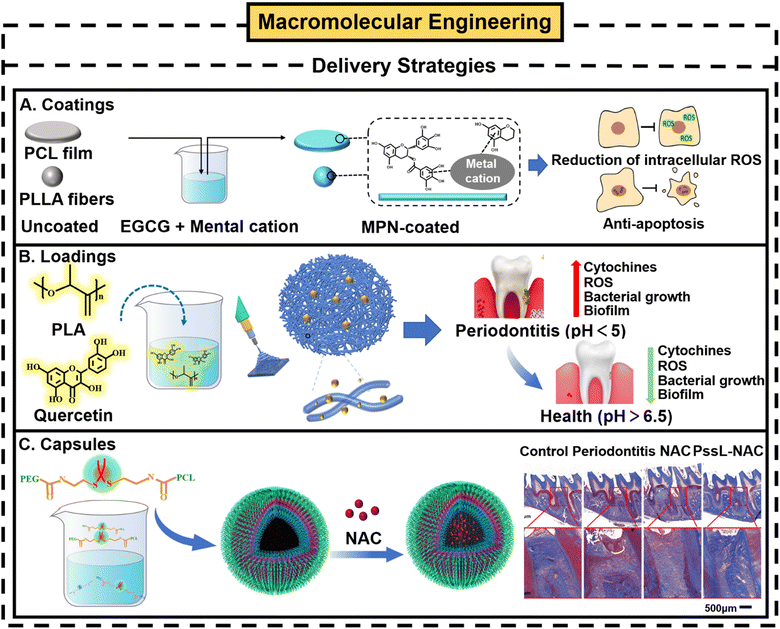 |
| Fig. 4 Loading strategies in macromolecular engineering strategy. (A) Schematic illustration of MPN coating on PCL membrane and the PLLA fiber and functions of them. Reproduced with permission from ref. 74, Copyright Elsevier 2021. (B) Application of PLA-QUE film in the construction process and treatment of periodontitis. (C) Synthesis of PEG-ss-PCL NPs containing NAC, and Masson trichrome staining results for periodontitis treatment. Reproduced with permission from ref. 26, Copyright Elsevier 2021. | |
In addition to direct coating, sending small molecular ROS scavengers as fillers in nanomaterials, or wrapping in nanomaterials as the main content is another delivery strategy.76,77 Quercetin (QUE) is one of the most promising molecules for the treatment of periodontal disease among selected flavonoids.61,78 Cristo et al. encapsulated QUE into electrospun polylactic acid (PLA) nanofibers by electrospinning.76 Owing to the pH responsiveness of PLA nanofibers and the network structure of electrospun membranes, the local residence time of QUE was significantly prolonged and its bioavailability was improved. Computational analysis showed that the prepared PLA-QUE membrane could release QUE continuously for 90 days under acidic and neutral conditions until almost 80% of the encapsulated drugs were released. In addition, because the acidic environment can catalyze the degradation of PLA, which leads to accelerated hydrolysis of ester bonds in the fiber and increases drug release, which endows the PLA-QUE membrane with pH responsiveness. In vitro studies showed that the slope of the release curve of QUE at pH 4.8 was about twice that of QUE at pH 6.8. The above results all prove the potential use of PLA-QUE film as an adjuvant in the treatment of periodontitis76 (Fig. 4B).
Except for directly loading small molecule ROS scavengers into delivery materials, capsulating them in the capsule structure formed by self-assembly of amphiphilic polymers is also a common strategy.26,79 The strategy often requires the introduction of ROS-responsive functional groups, such as sulfides, selenides, and thioketals to form ROS-reactive drug delivery systems.80 In short, these functional groups will be automatically cleaved under oxidative stress, which can achieve the targeted release of drugs. Qiu and his colleagues successfully prepared a new delivery carrier PEG-ss-PCL NPs (PSSL-NAC NPs) by introducing thioketal functional groups into the most commonly used biopolymer PEG-b-PCL.26 By wrapping NAC in customized ROS cleavable amphiphilic PEG-ss-PCL NPs as an intracellular delivery carrier, which is a controllable ROS-scavenging nanoplatform. PSSL-NAC NPs can realize the long-term release of NAC, control the level of ROS scavenging, and maintain a slight inflammatory state in the microenvironment. Studies have shown that the regulated oxidative microenvironment is more conducive to the proliferation and osteogenic differentiation of HPDLCs. Microcomputed tomography (Micro-CT), histological examination and Masson's trichrome staining show similar results. PSSL-NAC NPs can improve bone loss caused by periodontitis, and has better effects than just using NAC (Fig. 4C). To date, the delivery strategies of low-molecular-weight (LMW) ROS scavengers have been extensively studied including rich delivery carriers, such as liposomes, solid lipid nanoparticles, micelles, mesoporous silica, hydrogels, and nanofibers/membranes, as well as a variety of simple synthesis or polymerization methods such as “solvent pouring”, “freeze drying” and “one-pot methods”, all of which are continuously studied and improved.23,81 Except for natural small molecule ROS scavengers, natural macromolecular ROS scavengers, such as superoxide dismutase (SOD) based macromolecules, can be further fabricated with delivery strategies, although they have not been applied in the adjuvant treatment of periodontitis. For example, SOD encapsulated in biodegradable polyketone microparticles (PKSOD) can be continuously released in the myocardium, and significantly prolong the circulation time and residence time after ischemic heart reperfusion under protection by the microparticles against proteolytic degradation.82,83 Employing nanomaterials for the delivery of natural ROS scavengers has made great progress in improving the shortcomings of natural small molecules and exhibits huge potential in improving therapeutic effects. Nevertheless, there is still a gap in their clinical translation, mainly involving difficulties in the biological barrier, repeatability,10 unknown toxicity,12 large-scale production, and undesirable bio-persistence. In order to deal with the above-mentioned challenges, synthetic organic macromolecular biomaterials with ROS-scavenging activity could be prepared by direct polymerization of antioxidant monomers with appropriate structures or by grafting an antioxidant onto the main chain or branch chain of a polymer with good biosafety and biocompatibility.70 Polymerization strategies could better control the ratios of building blocks, and avoid the extra toxicity of high-dose drug administration.12 In addition, other functional drugs, dyes, chemotherapeutic or imaging agents could also be loaded into polymeric ROS-scavenging biomaterials to obtain additional effects, which will promote applications in more advanced biomedical fields.
(2) Polymerization strategies.
According to different antioxidant monomers, the common ones can be roughly divided into the following two categories:12,23,47
(i) Phenolic group-containing polymer nanomaterials. Phenolic compounds, with rich intermolecular interactions, can be widely introduced into polymers as main chain, side chain and end chain groups through condensation polymerization,10,84 free radical polymerization,85 oxidative polymerization, enzyme polymerization86 or metal chelation polymerization,87 to obtain effective organic high molecular ROS scavengers.47 For example, a study on the adjuvant treatment of periodontitis synthesized long-lasting and stable polyphenol nanoparticles by using one-step condensation polymerization.10 Chen et al. developed new functionalized epicatechin-3-gallate (EGCG, a green tea derivative) NPs through one-step polyphenol condensation polymerization using (EGCG), formaldehyde and amine. The results showed that when the concentration of EGCG NPs reached 10 μg mL−1, the clearance rate of ABTS reached 97%. Additionally, EGCG NPs had a long-lasting scavenging ability against ABTS, and its scavenging efficiency remained ≥95% on the 21st day. EGCG NPs not only have strong antioxidant capacity, but also improve the chemical stability of EGCG. In addition, EGCG NPs can effectively clear ROS and downregulate the expression of proinflammatory cytokines by reprogramming macrophages from M1 phenotype to M2 phenotype. In vivo results showed that EGCG NPs could reduce ROS levels by about 50% (from 1346.8 ± 244.9 μm to 596.1 ± 92.1 μm), inhibit alveolar bone loss and reduce osteoclast activity in a rat model of chronic periodontitis10 (Fig. 5A). Moreover, through free radical polymerization between the thermally responsive fragment poly(N-isopropylacrylamide) (PNIPAAm) and gallic acid molecule (GA) and covalent linkage to the biodegradable backbone gelatin network, a novel multifunctional GNGA polymer could be synthesized.85 The GNGA polymer has a strong ROS-scavenging effect, and shows a suitable phase-change temperature and degradation rate, which makes the material thermally responsive and more biodegradable. It has been successfully applied to the treatment of rabbit glaucoma models. Kang et al. covalently bound curcumin to an acid-sensitive amphiphilic polymer (β-amino lipids), wherein the synthesized acid-responsive anti-inflammatory curcumin polymer (ACP) micelles, which are more stable than free curcumin, have higher therapeutic activity, and have the potential to treat various inflammatory diseases (periodontitis) in addition to osteoarthritis.88 In another study, the antioxidant feature was directly introduced by the incorporation of multiple gallic acids, onto the POSS head of the shape amphiphiles using thiol–ene “click” chemistry.89 The series of gallic acid functionalized polyhedral oligomeric silsesquioxane (POSS)-based shape amphiphiles demonstrated good biocompatibility and strong ROS-scavenging activity which may be applicable to periodontal treatment. In addition, the phenolic groups can interact with aminoglycosides,87 proteins,90 polysaccharides91 or β-cyclodextrin,92 which can not only play anti-inflammatory and antioxidative roles but also improve the performance of these biomacromolecules by playing a role in stabilizing and improving hydrophilicity and biocompatibility. There has been increasing research on polymer nanomaterials containing phenolic groups, and considerable progress has been made. However, at present, some attempts will damage the reactivity of amorphous polyphenols in the reaction process, which is not conducive to the design of functional objects for chemical transformation. In addition, polyphenols combined with proteins,93 polysaccharides94 and polymers may also increase unnecessary immunogenicity risk during in vivo application.10
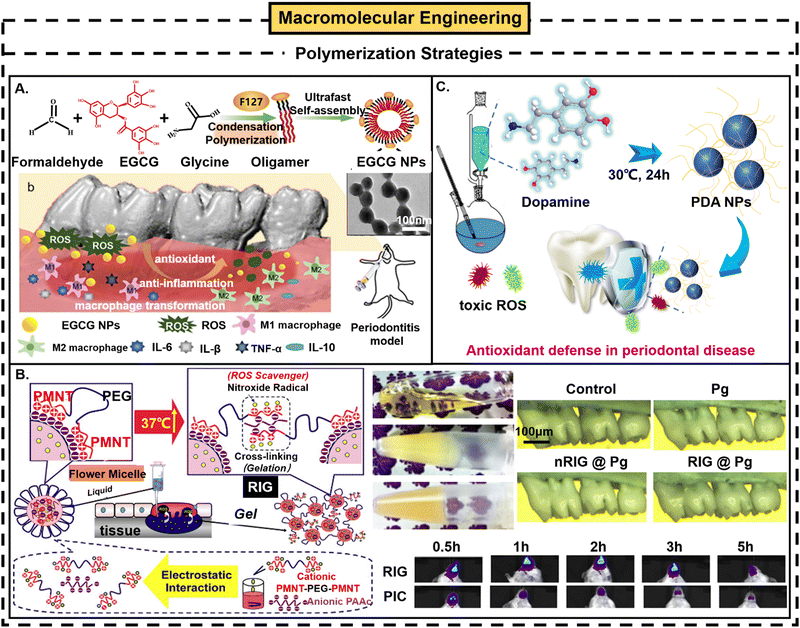 |
| Fig. 5 Polymerization strategies in macromolecular engineering strategy. (A) Schematic illustration of the synthesis process of EGCG NPs and their ROS-scavenging effect in the treatment of periodontitis. Reproduced with permission from ref. 10, Copyright Elsevier B.V. 2021. (B) Schematic diagram of the preparation process of RIG, state of RIG precursor solution before and after heating, and application of RIG in periodontitis treatment. Reproduced with permission from ref. 97 and 98, Copyright Elsevier 2013. (C) Application of PDA NPs in periodontitis. Reproduced with permission from ref. 20, Copyright American Chemical Society 2018. | |
(ii) Free radical trapper-containing polymer nanomaterials. Inspired by various methods to detect the ROS-scavenging capacity of antioxidants, different free radical traps can be used as new free radical scavengers;23 for example, 2,2,6,6-tetramethylpiperidin-N-oxyl (TEMPO) is a renowned ROS scavenger containing nitro-oxygen radicals (such as O2˙− and ˙OH), because it can capture unpaired electrons in other free radicals.95,96 Therefore, scholars are committed to developing antioxidative polymer nanomaterials by integrating TEMPO or its derivatives into the polymer backbone.95–98 Generally, TEMPO covalently bonds to amphiphilic polymer chains by polymerization or post-modification strategies. The polymers inherit the scavenging ability of free TEMPO molecules for ˙OH, O2˙− and H2O2, and are more stable in vivo.88–90 In another study, a redox active injection gel (RIG) system was developed through a simple mixing method.97 The poly(4-(2,2,6,6-tetramethylpiperidine-N-oxyl)aminomethylstyrene)-b-poly(ethylene glycol)-b-poly(4-(2,2,6,6-tetramethylpiperidine-N-oxyl)) aminomethylstyrene (PMNT–PEG–PMNT) triblock copolymer has ROS-scavenging nitrogen oxide radicals (TEMPO) as the side chains of PMNT segments. Cationic PMNT fragments in PMNT–PEG–PMNT form polyionic complexes with anionic polyacrylic acid (PAAC) to form flower-like micelles (about 79 nm), which can freely self-assemble and exhibit in situ thermal irreversible gelation under physiological conditions. In vitro experiments show that the RIG precursor solution is transparent before heating. After heating in vitro at 37 °C, it turns into a turbid gel, and remains in the gel state even after cooling to room temperature. In addition, compared with a PIC micelle solution (no gel formation), the RIG system can be retained in the lesion area of periodontitis for a long time. In contrast to low-molecular-weight nitrogen oxide radical compounds that disappeared from the injection site within less than 1 hour after subcutaneous injection, 40% of RIG remained even after 3 days. Moreover, TEMPO is a SOD-mimetic agent, which is always used together with phenylboronic acid pinacol ester and has a good ROS-scavenging effect99 (Fig. 5B).
Although research on ROS-scavenging biomaterials based on polymers has achieved outstanding results, the relatively new issue of their safety (also defined as nanotoxicity) has attracted considerable attention.23 Biocompatibility and biodegradability are prerequisites and key factors for these biomaterials to be suitable for in vivo applications. It has been proven that these exogenous ROS-scavenging biomaterials can lead to potentially harmful side effects, such as induction of mitochondrial respiration, activation of immune cells, and genotoxicity, which pose a great challenge to clinical application.23 Because we were inspired by nature to start studying ROS-scavenging biomaterials, finding answers in nature would be a wise strategy.100 Perhaps the use of natural macromolecular polymers with inherent ROS-scavenging ability can make up for the above shortcomings. In the continuous exploration of nature, researchers have also found such natural antioxidative polymers with inherent ROS-scavenging activity (such as bilirubin, melanin and melanin derivatives).20,23
(3) Natural polymer with intrinsic ROS-scavenging activity.
In addition to the above-mentioned natural enzyme antioxidant defense system, scientists have paid attention to the antioxidant properties of proteins, peptides and polysaccharides. Bilirubin, as a natural metabolite in the human body, is considered an effective endogenous antioxidant.101 Its antioxidant properties may be attributed to the oxidation of water-insoluble bilirubin to water-soluble biliverdin in the oxidative stress microenvironment.102 However, bilirubin has major problems of poor stability, low water solubility and low bioavailability. In order to maximize the antioxidant properties of bilirubin, Jon and his colleagues synthesized PEGylated bilirubin (PEG-BR NPs) through covalent binding and self-assembly. PEG-BR NPs not only inherit the excellent anti hydrogen peroxide ability of natural bilirubin but are also more stable and sustainable in vivo or in vitro.102,103 In addition, the excellent antioxidant properties of PEG-BR NPs have been verified in many established disease models. They can also promote the conversion of M1-type macrophages to M2-type macrophages by inhibiting the release of inflammatory cytokines, and reduce the inflammatory response.
Melanin is a type of biological macromolecular pigment that exists in most organisms; it has many interesting physical, chemical and biological functions, such as photoprotection, photothermal conversion, metal ion chelation, free radical quenching, anti-inflammatory and antioxidant properties and radiation protection.104 Polydopamine (PDA), as a melanin analog, has similar functions. Based on these excellent functions,105 PDA and its analogues have been used in the treatment of a series of diseases, including ischemic stroke induced by ROS and nitrogen species, acute inflammatory injury, and periodontal disease caused by oxidative stress and osteoarthritis.105,106 Bao et al. successfully developed an intelligent ROS-scavenging platform using PDA NPs. By synthesizing PDA NPs using the classical Stober method at room temperature, the antioxidative properties and related therapeutic mechanisms of PDA NPs in periodontal diseases were explored for the first time. Studies have confirmed that synthetic PDA NPs have more uniform morphologies than natural melanin particles, as well as good stabilities, which enables them to be well dispersed in many physiological solutions and maintains long-term monodispersed stabilities for potential biological related applications. In the LPS-induced periodontitis rat model, PDA NPs significantly reduced the ROS level of the LPS-treated group, and no histological side effects were observed.20 It was found that PDA NPs have the same enzyme catalytic activity as SOD and are more stable and lasting. Even if they are stored at low temperature or different pH conditions for 1 year, their catalytic activity will not decrease significantly.107,108 This study provides valuable insights for the development of a safe and effective antioxidant defense platform (Fig. 5C).
Finally, other covalent organic frameworks (COFs) are very likely to become promising ROS-scavenging biomaterials. COFs are a class of newly emerging porous crystalline polymer framework, prepared solely from light elements (H, C, N, O, B), which exhibit a large specific surface area, high thermal stability, good biocompatibility, and promising biodegradation, attracting great interest in the biomedical field, including in photodynamic therapy,109 chemokinetic therapy,110 immunotherapy111 and drug delivery.112–114 Traditionally used porous polymer materials include mesoporous silica (MSN), metal NPs, porous carbon, organic micelles, and liposomes, which have unavoidable shortcomings and limitations, such as prolonged excretion and retention inside the body, low drug loading capacity, drug leaching, undesirable toxicity, and poor dispersibility.115 Highly ordered structures of COFs can be elaborately designed using reticular framework chemistry and experimentally synthesized through strong covalent bonds, thereby improving the shortcomings of traditional porous polymer materials and provide greater opportunities for controllable design and manufacturing.114 However, the research results and potential properties of COFs in the treatment of periodontitis have rarely been reported. Zhang et al. confirmed that through a facile one-pot method to prepare a curcumin-loaded COF (CUR@COF), which has a high CUR loading capacity of 27.68%, and exhibits increased thermal stability, improved mechanical properties, better biocompatibility, and enhanced antibacterial and antioxidant activities could be achieved.112 COF-based ROS-scavenging biomaterials have rich active centers and high-density active sites, which are undoubtedly beneficial to the design and preparation of COF-based ROS-scavenging biomaterials. Moreover, they can perhaps better adapt to and adjust the oxidative stress microenvironment during periodontitis. In addition to being used as a delivery carrier, with the deepening of research into COF materials, researchers are constantly trying to combine various organic or inorganic structural units with special intrinsic functions into different types of COFs to confer specific functionality to COFs and enable mimicking of the enzymes of catalytic activity.116 However, the types of COFs used to mimic enzymes are relatively single, and most of them use iron porphyrin COFs or CTFs as substrates.116 Therefore, we should focus on designing new COF materials with the properties of mimicking enzymes. In addition, compared with natural enzymes, the specificity of mimicking enzymes is poor, and it is difficult to catalyze a specific substrate. Therefore, in order to realize the specific catalysis of COFs mimicking enzymes, it is necessary to further explore the catalytic mechanism of COFs mimicking enzymes and improve their selectivity.116
3.2. Inorganic ROS-scavenging biomaterial
The overlap between inorganic chemistry and nanotechnology is promoting the rapid development of inorganic ROS-scavenging nanomaterials. Fullerenes with simulated SOD activity are inorganic nanomaterials first reported in 1996, representing a milestone in the development of inorganic nanoparticles to remove ROS.117 Unlike organic ROS-scavenging biomaterials, inorganic nanomaterials, with high stability, stronger tolerance to harsh microenvironments and low cost, additionally have intrinsic catalytic performance or ROS-scavenging properties.23 ROS-based inorganic nanoplatforms have a variety of rich design methods to further improve the ROS-scavenging activity of inorganic biomaterials, to guide the elimination of excess ROS in the body, thereby enabling their participation in the regulation of the cellular redox balance as important exogenous interventions.81,118 In this section, we briefly introduce some inorganic materials with intrinsic antioxidant activity, mainly focusing on ROS-scavenging biomaterials based on metal–organic frameworks (MOFs).
3.2.1. Inorganic material with ROS-scavenging activity.
(1) Metal-based ROS-scavenging biomaterials.
Since Zn2+-triazacyclonane-functionalized gold nanoparticles with phosphoesterase-like activity119 and Fe3O4 nanoparticles with intrinsic peroxidase-like activity120 were reported by Scrimin et al. in 2004 and Yan et al. in 2007, the study of nanozymes has made great progress. Metal-based materials with strong ROS-scavenging abilities are a large group of inorganic antioxidants.118 Metal elements, such as Fe, Cu, Zn, V, Mn, Mo, Ce, Pt, and Au, have been extensively studied, and can exist as metal elements, metal oxides, or metal–metal oxide composites.118 In different experimental results, metal-based ROS-scavenging biomaterials can show catalytic activity similar to peroxidase (POD), CAT, SOD, glucose oxidase (GOx) and glutathione peroxidase (GPx).23 Ceria oxide nanoparticles exhibit both SOD-mimetic and CAT-mimetic activities, attributed to the mixed valence states of Ce3+ and Ce4+.121 Because of their excellent antioxidative properties and automatic regeneration of redox cycling ability, they have become the most widely used inorganic nanomedicines for ROS-scavenging therapy. CeO2 NPs contain two oxidation states Ce3+ and Ce4+, which coexist on the surface of the Ce oxide lattice.25 Ce4+ is mainly contained in the massive CeO2 crystal, but the relative content of Ce3+ increases greatly in the process of reduction to nanometer size.13 The reduced positive charge in unstable Ce3+ is compensated by the corresponding number of oxygen vacancies, so that the Ce3+ concentrations and oxygen vacancies on the surface of CeO2 are higher than those of the bulk material, and Ce4+ can be reduced to Ce3+ given the presence of surface oxygen vacancies, therefore effectively decreasing ROS levels.13 Compared with various biological processes and biological antioxidants, CeO2 NPs have higher catalytic activity.33 Yu et al. attempted to treat periodontitis with an in situ injection of CeO2 NPs.37In vitro and in vivo experiments provided strong evidence for the role of CeO2 NPs in the clearance of multiple ROS and the inhibition of ROS-induced lipopolysaccharide-stimulated inflammatory responses. In addition, CeO2 NPs inhibit inflammatory factors by inhibiting the MAPK-NF-κB signaling pathway. The results of a rat periodontitis model also showed that CeO2 NPs significantly reduced alveolar bone resorption, osteoclast activity and inflammation, thus improving the recovery of damaged tissue (Fig. 6A). Similarly, other studies showed similar results, regardless of whether CeO2 NPs are loaded onto nanofiber membranes by electrospinning or CeO2 NPs are loaded with MSNs.24,25 In addition to the Ceria oxide nanoparticles, some other inorganic nanoparticles have been examined for ROS scavenging, such as gold/platinum,117 selenium,122 copper123 and vanadium,124 all of which have good ROS-scavenging ability, and can effectively cope with the oxidative stress environment and play a role in cell protection. Despite an explosion of fundamental and practical studies that have been carried out for the applications of metallic ROS-scavenging biomaterials in both cellular and in vivo animal models of different oxidative stress-related diseases, the solubility and stability of metal-based ROS-scavenging biomaterials in blood are poor, and there may be other unknown toxicities when they exist in vivo for a long time, all of which hinder the clinical applications of metal-based ROS-scavenging biomaterials.12
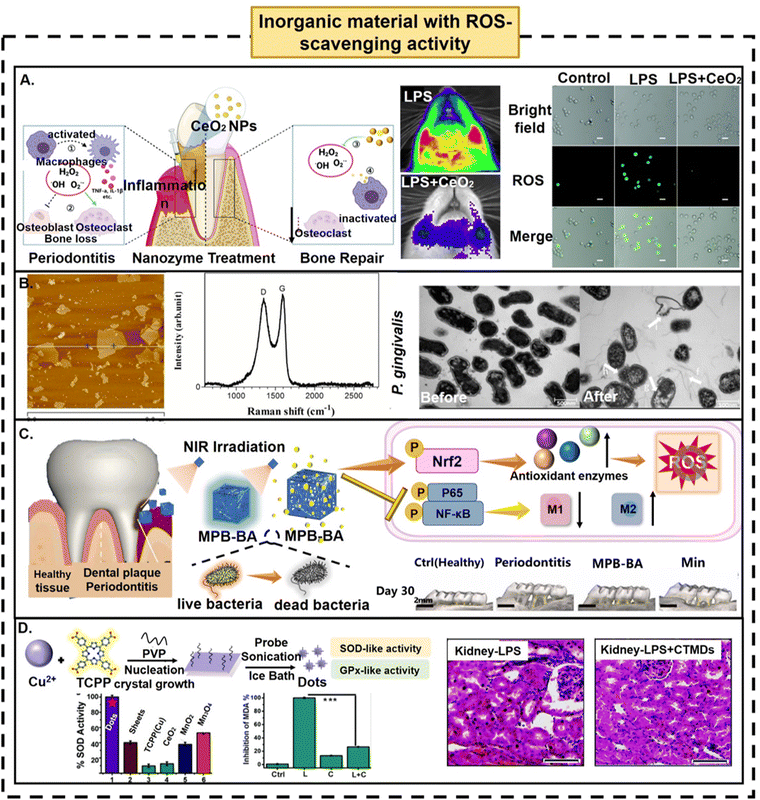 |
| Fig. 6 Polymerization strategies in macromolecular engineering strategy. (A) Schematic illustration of the preparation process and antioxidant effect of CeO2 NPs. Scale bar: 20 μm. Reproduced with permission from ref. 37, Copyright the Royal Society of Chemistry 2022. (B) A typical GO nanosheet and its antibacterial effect on Pg. Scale bar: 500 nm. Reproduced with permission from ref. 130, Copyright American Chemical Society 2015. (C) Preparation process, functional mechanism and application of MBP-BA NPs in a rat periodontitis model. Scale bar: 2 mm. Reproduced with permission from ref. 139, Copyright Elsevier B.V. 2021. (D) Preparation of biomimetic antioxidant enzyme CTMDs and H&E staining images of kidney tissues in each group. Scale bar: 50 μm. Reproduced with permission from ref. 143, Copyright Royal Society of Chemistry 2019. | |
(2) Carbon-based ROS-scavenging biomaterials.
Carbon-based ROS-scavenging biomaterials represent another large class of inorganic antioxidants.23,118 Since the advent of fullerene, various carbon biomaterials with different structures and compositions, such as carbon nanotubes (CNTs), carbon particles, carbon nanoclusters, graphene and graphene quantum dots (GQDs), and carbon quantum dots (CQDs),23,125 have been vigorously explored. Compared with natural enzymes, carbon-based biomaterials with good peroxidase or antioxidant enzyme activity are low in cost, easy to store, and have better stability.23 In general, materials containing conjugated C
C chains typically possess ROS-scavenging capability. For example, the most representative fullerenes and their derivatives can effectively scavenge hydroxyl radicals and superoxide anions, which are mainly determined by electron transfer and adduct formation mechanism.118 In addition, carbon-based biomaterials with large specific surface area, good hydrophilicity, and different oxygen-containing functional group modifications can also affect their ROS-scavenging capacity, which provides a great space to imagine further designs. Based on their excellent physical and chemical properties, their ROS-related biomedical applications have been extensively studied, such as bone/cartilage regeneration,126 gastric injury,127,128 acute kidney injury129 and other diseases. In 2015, Tang et al. using the modified Hummers’ method to prepare graphene oxide (GO) from natural graphite, typical Raman spectra indicating the successful synthesis of GO, confirmed that GO nanosheets can effectively inhibit or even eliminate periodontal pathogens by regulating the level of ROS125,130 (Fig. 6B). Another group found that carbon-based ROS scavengers contributed to the proliferation of periodontal ligament stem cells, osteogenic differentiation and subsequent periodontal tissue regeneration.131–133 However, the mechanism of carbon-based ROS-scavenging biomaterials in periodontal tissue regeneration is still unclear, and more mechanisms and physiological pathways should be illustrated in further research. In addition, carbon-based ROS-scavenging biomaterials can also be used as conductors for the treatment of diabetes periodontitis. Zhao et al. modified GO with PDA to obtain graphene oxide (PGO), which not only has good dispersion stability, but also has better conductivity.134 PGO in the biological scaffold provides a conductive path, through which the scaffold can transmit endogenous electrical signals to cells to activate Ca2+ channels to promote bone regeneration. To date, although great efforts have been made to develop assorted carbon-based ROS-scavenging biomaterials, and in-depth studies have been carried out in animal models of oxidative stress diseases to assess their therapeutic potential, compared with natural enzymes, the catalytic efficiency of carbon-based ROS-scavenging biomaterials is still low, and there are still only a few studies on carbon-based ROS-scavenging biomaterials in periodontal therapy.
3.2.2. Inorganic ROS-scavenging biomaterials with MOF-based nanostructures.
Both organic and inorganic ROS scavengers have made great progress in antioxidation. However, they still have obvious shortcomings affecting their development in clinical application. A promising solution to address these current challenges is to combine the organic and inorganic ROS-scavenging biomaterials and make up for their respective limitations.135 Metal–organic frameworks (MOFs) constructed from metal ion/cluster nodes and functional organic ligands through coordination bonds are an attractive class of coordination polymer.136 Owing to their high surface area, high stability, good biocompatibility, flexible and adjustable nanoscale porosity and rich spatial structure, MOFs have attracted extensive research interest during past decades.136 Their individual structural composition and outstanding inherent characteristics endow MOF-based materials with rich active sites, which can well resist and eliminate excessive free radicals, allowing for effective antioxidation.137 The rich active sites are similarly beneficial for regulating the oxidative stress microenvironment of periodontitis. Except for directly being ROS scavengers, MOF biomaterials can be used as carriers of natural enzymes or small ROS scavengers and hold the promise of efficient cascade catalytic reactions.138 For example, baicalein (BA) was loaded onto mesoporous Prussian blue (MPB) nanoparticles by using simple “one-pot” methods.139 The constructed MPB-BA nano-platform can effectively improve the deficiencies of traditional organic small molecule antioxidant BA. The authors first mixed poly(vinylpyrrolidone) (PVP), potassium ferricyanide (K3[Fe(CN)6]) in HCl solution and stirred them until clarified. Then, the mixed solution was heated for 20 hours to prepare mesoporous Prussian blue (MPB) nanoparticles. Next, 25 mL of BA solution (1 mg mL−1 in PBS) was added to 25 mL of MPB solution (4 mg mL−1 in PBS) at room temperature under continuous stirring, and the final MPB-BA nanoparticles were obtained by rotation, centrifugation and drying. The new MPB-BA system has antioxidant, anti-inflammatory and antibacterial properties by combining photothermal therapy (PTT), immunotherapy and nano-enzymes, to effectively kill periodontal pathogens. MPB-BA shows excellent stability under near-infrared (NIR) light,140,141 and can intelligently control the release of BA, through downregulation of the NF-κB inflammation signal pathway and upregulation of Nrf2 factors to enhance the expression of many antioxidant enzymes, which promote the transformation of macrophages from M1 phenotype to M2 phenotype. Furthermore, the micro-CT reconstruction image of the periodontitis model shows that MPB-BA has good periodontal protection ability, and its effect is better than that of minocycline hydrochloride (Min) (Fig. 6C). Recent efforts have also been made to develop MOF-based enzymes with bionic antioxidant enzyme catalytic activity.138,142 Qu's group prepared biomimetic ultra-small Cu-TCPP-MOF nanodots (CTMDs) of biomimetic size using a simple liquid spalling strategy.143 CTMDs have enzyme catalytic activity similar to natural SOD, owing to the coordinated environment between metals and ordered channels similar to substrate channels, which endow them with even better activity than SOD. In this system, Cu as the active site presents the coordination of N and O atoms comparable to natural SOD. CTMDs can not only effectively simulate the antioxidant SOD, but also shows similar activity to GPx. Combined with GPx simulation activity, bionic CTMDs can effectively eliminate O2˙ and H2O2, and realize the cascade catalytic reaction of enzymes. Both in vivo and in vitro studies have confirmed that CTMDs have a good ROS-scavenging catalytic capacity and can effectively scavenge free radicals in lipopolysaccharide-induced acute kidney injury (Fig. 6D). As mentioned above, MOFs have been widely studied, although there are only a few studies on their application in periodontitis treatment. Based on their excellent biological characteristics and better design flexibility, perhaps ROS-scavenging biomaterials with MOF-based nanostructures can more effectively treat periodontitis. However, the water/chemical stability of MOFs still needs to be improved, as the leaching of toxic metal ions is possible and, hence, their biological safety still needs to be considered.144
4. Current states and future prospects
To sum up, various forms of ROS-scavenging biomaterials applied to periodontal treatment and periodontal tissue regeneration, including films,76 hydrogels and nanoparticles,20 have achieved satisfactory results. However, most of these materials need to be treated with injections,20 surgical implantation76 or oral administration, which are usually cumbersome and invasive, and may cause systemic toxicity. Spray or mouthwash is generally considered an ideal carrier to reach all inaccessible areas in the oral cavity, which have a general plaque control effect and are easy to use.145 However, most traditional mouthwashes are directly mixed with the original ingredients by using organic small molecules in natural extracts as auxiliary active ingredients. Due to the continuous fluidity of saliva, the instability of small molecules and their poor permeability in biofilms, there are usually problems such as unstable drug release, short residence time, low bioavailability and difficulty in controlling dosing, which seriously limit the clinical development and application of mouthwashes and sprays28 (Fig. 7A). With the proposal and development of macromolecular engineering strategies, the shortcomings of natural small molecule materials have been greatly improved. Inspired by the colloidal delivery system, Li et al. reported a long-acting new mouthwash (CCH-MI) in which the active ingredients, such as minocycline hydrochloride (MH), can stay in the biofilm for up to 12 hours.146 The physical adherence and chemical combination between chitosan (CS), the catechol group in hydrocaffeic acid (HCA) and the biofilm are the reason for achieving long-term drug release. The development of this long-lasting mouthwash is exciting, which means that toothpastes or chewing preparations (such as medical chewing gum [MCG]) with ROS-scavenging function can be prepared by using polymer strategies to prevent the formation of plaque biofilms while helping to stabilize the local redox balance of periodontitis patients.28,147 Periodontitis is a chronic inflammatory disease caused by imbalance between host immune response and pathogens, with complex etiology. Antioxidant therapy is considered a method of host immunomodulation therapy.148 However, it is difficult to achieve an ideal therapeutic effect by using antioxidative therapy exclusively.148 As summarized in this review, no matter whether they were the polyphenols, vitamins or proteins mentioned among the organic ROS-scavenging biomaterials, or the metal-based biomaterials as well as carbon-based biomaterials mentioned among the inorganic ROS-scavenging biomaterials, they all showed satisfactory antioxidative properties, but other excellent biological properties, such as hydrophilicity, bio-adhesiveness, and photothermal properties also play an important role. Therefore, according to the biological characteristics of different materials, biomaterials could combine with functional molecules which could be used to guide the design of multi-functional biomaterials, and make materials with multiple responses to physical and chemical factors (ROS, temperature and pH), for better therapeutic effects149,150 (Fig. 7B). Based on the fast development and great potential of ROS-scavenging biomaterials, they can be further integrated with other kinds of intelligent technologies, such as self-detecting systems and smart biosensors/intelligent devices.151,152 In this way, the periodontal condition of the users can be timely detected and fed back to users. Users can know their periodontal condition in real time in their daily lives, and can judge whether treatment should be carried out as early as possible in the hospital according to the results of ROS-related intelligent detection equipment. Through this method patients with early periodontitis can get timely clinical treatment and have better clinical efficacy, rather than teeth loosening and falling out in the late stages of periodontitis (Fig. 7C). In addition, it is also anticipated that ROS-scavenging biomaterials combined with smart biosensors/smart devices can be placed together in periodontal tissues to achieve intelligent controlled drug release, by sensing changes in periodontal microenvironment. It is undoubtedly an effective and good treatment for patients after periodontal surgery, patients with periodontal tissue destruction due to genetic factors or systemic diseases, and the patients with poor compliance (Fig. 7C).
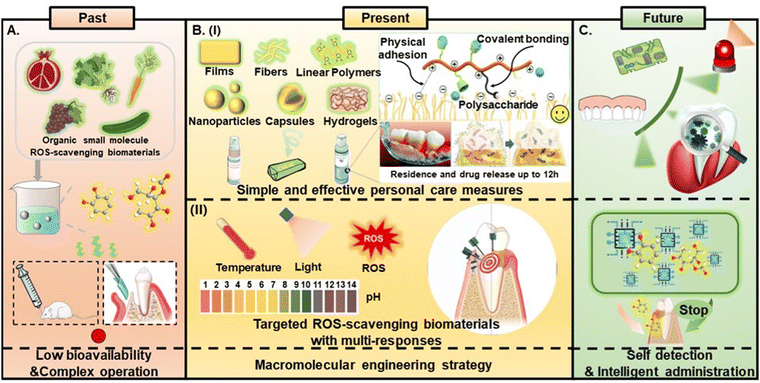 |
| Fig. 7 Past, present and future of ROS-scavenging biomaterials for periodontitis treatment. (A) Past developments of periodontal treatment using organic small molecule ROS-scavenging biomaterials as the ingredients. (B) Current status of periodontal treatment using macromolecular engineering strategy. Reproduced with permission from ref. 146, Copyright Royal Society of Chemistry 2021. (C) Future prospects of ROS-scavenging biomaterials, including self-detecting systems and smart biosensors/intelligent devices. | |
5. Conclusion
This review elucidates the role of ROS in the occurrence and development of periodontitis, including the production process of reactive oxygen species in periodontitis and the possible pathogenesis related to ROS, and discusses it according to two aspects: direct injury and indirect injury. The excessive production of ROS leads to the release of inflammatory factors, which promotes a series of biological events, such as direct damage to bioactive macromolecules and indirect activation of downstream inflammatory pathways. The disorder of redox balance in the body is the main inducement leading to the rapid progress of periodontitis. Therefore, the auxiliary use of ROS-scavenging materials in the treatment of periodontitis has become a promising treatment strategy. Based on this, we summarized the biomaterials with ROS-scavenging activity currently used in the adjuvant treatment of periodontitis from both organic and inorganic aspects. Looking forward to the future, we can even combine ROS-scavenging biomaterials with a variety of intelligent technologies, and introduce a unified evaluation system through BigData analysis to accurately understand what level of ROS is most beneficial to health, and design personalized ROS-scavenging intelligent biomaterials on demand. Although biomaterials with ROS-scavenging activity have made considerable progress in periodontitis treatment, this emerging field is still at a relatively early stage. Many difficulties need to be overcome to achieve clinical conversion of ROS-scavenging biomaterials. We must carefully consider the acute and long-term chronic toxicity, biodegradability and biosafety of ROS-scavenging biomaterials. With continuous exploration and research, it is believed that the above challenges related to ROS-scavenging biomaterials can be addressed in the foreseeable future. We expect that rapid and widespread applications of ROS-scavenging biomaterials for the prevention and/or treatment of periodontitis will be achieved.
Author contributions
E. C. and T. W. contributed equally to this work, and the manuscript was written through the contributions of all authors. All authors have given approval to the final version of the manuscript.
Conflicts of interest
There are no conflicts to declare.
Acknowledgements
The authors are indebted to many scientists whose research results are cited here. This work was financially sponsored by the National Natural Science Foundation of China (No. 52203179), Sichuan Province Science and Technology Support Programs (No. 22SYSX0168).
References
- G. Hajishengallis, Nat. Rev. Immunol., 2015, 15, 30–44 CrossRef CAS.
- R. J. Genco and T. E. Van Dyke, Nat. Rev. Cardiol., 2010, 7, 479–480 CrossRef PubMed.
- Y. W. Han and X. Wang, J. Dent. Res., 2013, 92, 485–491 CrossRef CAS PubMed.
- S. E. Whitmore and R. J. Lamont, PLoS Pathog., 2014, 10, e1003933 CrossRef PubMed.
- R. J. Genco and M. Sanz, J. Periodontol., 2020, 83, 7–13 CrossRef PubMed.
- N. Kassebaum, E. Bernabé, M. Dahiya, B. Bhandari, C. Murray and W. Marcenes, J. Dent. Res., 2014, 93, 1045–1053 CrossRef CAS PubMed.
- P. Petersen and H. J. P. Ogawa, J. Periodontol. 2000, 2012, 60, 15–39 CrossRef.
- A. Gross, K. Paskett, V. Cheever and M. J. P. M. J. Lipsky, Postgrad. Med. J., 2017, 93, 560–565 CrossRef PubMed.
- X. Chen, G. Wu, Z. Feng, Y. Dong, W. Zhou, B. Li, S. Bai and Y. J. Zhao, Crit. Rev. Biotechnol., 2016, 36, 760–775 CrossRef CAS PubMed.
- M. Tian, G. Chen, J. Xu, Y. Lin, Z. Yi, X. Chen, X. Li and S. Chen, Chem. Eng. J., 2022, 433, 132197 CrossRef CAS.
- F. Sczepanik, M. Grossi, M. Casati, M. Goldberg, M. Glogauer, N. Fine and H. J. P. Tenenbaum, J. Periodontol., 2020, 84, 45–68 CrossRef PubMed.
- J. Yeo, J. Lee, S. Lee and W. J. Kim, Adv. Ther., 2021, 4, 2000270 CrossRef CAS.
- H. Kumar, K. Bhardwaj, E. Nepovimova, K. Kuča, D. Dhanjal, S. Bhardwaj, S. Bhatia, R. Verma and D. J. N. Kumar, Nanomaterials, 2020, 10, 8458 Search PubMed.
- H. Kanzaki, S. Wada, T. Narimiya, Y. Yamaguchi, Y. Katsumata, K. Itohiya, S. Fukaya, Y. Miyamoto and Y. Nakamura, Front. Physiol., 2017, 8, 351 CrossRef.
- H. Zhang, C. Huang, J. Zhang, C. Wang, T. Wang, S. Shi, Z. Gu and Y. Li, Giant, 2022, 12, 100120 CrossRef CAS.
- T. Chu, V. P. Tuan, J. S. Te and I. T. Lee, Antioxidants, 2020, 9, 2094665 Search PubMed.
- M. Mittal, M. Siddiqui, K. Tran, S. Reddy, A. J. A. Malik and R. Signaling, Antioxid. Redox Signaling, 2014, 20, 1126–1167 CrossRef CAS PubMed.
- L. Sui, J. Wang, Z. Xiao, Y. Yang, Z. Yang and K. Ai, Front. Chem., 2020, 8, 595530 CrossRef CAS PubMed.
- C. Liu, L. Mo, Y. Niu, X. Li, X. Zhou and X. Xu, Front. Physiol., 2017, 8, 439 CrossRef PubMed.
- X. Bao, J. Zhao, J. Sun, M. Hu and X. Yang, ACS Nano, 2018, 12, 8882–8892 CrossRef CAS PubMed.
- Y. Wang, C. Li, Y. Wan, M. Qi, Q. Chen, Y. Sun, X. Sun, J. Fang, L. Fu, L. Xu, B. Dong and L. Wang, Small, 2021, 17, 2101505 CrossRef CAS PubMed.
- X. Zhao, Y. Yang, J. Yu, R. Ding, D. Pei, Y. Zhang, G. He, Y. Cheng and A. Li, Biomaterials, 2022, 282, 121387 CrossRef CAS PubMed.
- Y. Liu and J. Shi, Nano Today, 2019, 27, 146–177 CrossRef CAS.
- S. Ren, Y. Zhou, K. Zheng, X. Xu, J. Yang, X. Wang, L. Miao, H. Wei and Y. Xu, Bioact. Mater., 2022, 7, 242–253 CrossRef CAS.
- S. Ren, Y. Zhou, R. Fan, W. Peng, X. Xu, L. Li and Y. Xu, Chem. Eng. J., 2021, 423, 130207 CrossRef CAS.
- X. Qiu, Y. Yu, H. Liu, X. Li, W. Sun, W. Wu, C. Liu and L. Miao, Acta Biomater., 2021, 135, 593–605 CrossRef CAS PubMed.
- S. Liu, Y.-N. Wang, L. Yu, J. Li and S. Ge, Chem. Eng. J., 2022, 432, 134308 CrossRef CAS.
- X. Zhang, Z. Li, P. Yang, G. Duan, X. Liu, Z. Gu and Y. Li, Mater. Horiz., 2021, 8, 145–167 RSC.
- G. Pizzino, N. Irrera, M. Cucinotta, G. Pallio, F. Mannino, V. Arcoraci, F. Squadrito, D. Altavilla and A. Bitto, Oxid. Med. Cell. Longevity, 2017, 2017, 8416763 Search PubMed.
- B. Yang, Y. Chen and J. Shi, Chem. Rev., 2019, 119, 4881–4985 CrossRef CAS PubMed.
- D. Q. Tan and T. Suda, Antioxid. Redox Signaling, 2017, 29, 149–168 CrossRef PubMed.
- I. I. C. Chio and D. A. Tuveson, Trends Mol. Med., 2017, 23, 411–429 CrossRef CAS PubMed.
- C. W. Li, L. L. Li, S. Chen, J. X. Zhang and W. L. Lu, Front. Bioeng. Biotechnol., 2020, 8, 200 CrossRef PubMed.
- Y. Wang, O. Andrukhov and X. Rausch-Fan, Front. Physiol., 2017, 8, 910 CrossRef PubMed.
- K. Neha, M. Haider, A. Pathak and M. Yar, Eur. J. Med. Chem., 2019, 178, 687–704 CrossRef CAS PubMed.
- M. Greabu, F. Giampieri, M. M. Imre, M. Mohora, A. Totan, S. M. Pituru and E. Ionescu, Molecules, 2020, 25, 4338 CrossRef CAS PubMed.
- Y. Yu, S. Zhao, D. Gu, B. Zhu, H. Liu, W. Wu, J. Wu, H. Wei and L. J. N. Miao, Nanoscale, 2022, 14, 2628–2637 RSC.
- J. Zhang, X. Wang, V. Vikash, Q. Ye, D. Wu, Y. Liu and W. Dong, Oxid. Med. Cell. Longevity, 2016, 2016, 4350965 Search PubMed.
- T. Nagasawa, M. Kiji, R. Yashiro, D. Hormdee, H. Lu, M. Kunze, T. Suda, G. Koshy, H. Kobayashi, S. Oda, H. Nitta and I. Ishikawa, Exp. Ther. Med., 2007, 43, 65–84 Search PubMed.
- Y. Wang, H. Li, Y. Feng, P. Jiang, J. Su and C. Huang, Int. J. Nanomed., 2019, 14, 963–976 CrossRef CAS PubMed.
- M. Yokoji-Takeuchi, N. Takahashi, M. Yamada-Hara, B. Sulijaya, T. Tsuzuno, Y. Aoki-Nonaka, K. Tabeta, S. Kishino, J. Ogawa and K. Yamazaki, Arch. Oral Biol., 2020, 110, 104602 CrossRef CAS PubMed.
- J. T. Marchesan, M. S. Girnary, K. Moss, E. T. Monaghan, G. J. Egnatz, Y. Jiao, S. Zhang, J. Beck and K. V. Swanson, J. Periodontol., 2020, 82, 93–114 CrossRef.
- F. R. G. Rocha, A. E. Delitto, J. A. C. de Souza, L. A. Gonzalez-Maldonado, S. M. Wallet and C. Rossa Junior, Sci. Rep., 2020, 10, 7823 CrossRef CAS.
- C. Sima, G. M. Aboodi, F. S. Lakschevitz, C. Sun, M. B. Goldberg and M. Glogauer, Am. J. Pathol., 2016, 186, 1417–1426 CrossRef CAS.
- L. Shi, Y. Ji, S. Zhao, H. Li, Y. Jiang, J. Mao, Y. Chen, X. Zhang, Y. Mao, X. Sun, P. Wang, J. Ma and S. Huang, Free Radical Biol. Med., 2021, 172, 19–32 CrossRef CAS PubMed.
- I. Chapple and J. J. P. Matthews, J. Periodontol. 2000, 2007, 43, 160–232 CrossRef PubMed.
- J. Zhang, Y. Fu, P. Yang, X. Liu, Y. Li and Z. Gu, Adv. Mater. Interfaces, 2020, 7, 2000632 CrossRef CAS.
- I. Gulcin, Arch. Toxicol., 2020, 94, 651–715 CrossRef CAS PubMed.
- I. Khalil, W. A. Yehye, A. E. Etxeberria, A. A. Alhadi, S. M. Dezfooli, N. B. M. Julkapli, W. J. Basirun and A. Seyfoddin, Antioxidants, 2020, 9, 24 CrossRef CAS.
- T. Wang, Q. Fan, J. Hong, Z. Chen, X. Zhou, J. Zhang, Y. Dai, H. Jiang, Z. Gu, Y. Cheng and Y. Li, Small, 2021, 17, 2102485 CrossRef CAS PubMed.
- Y. Zheng, C. Branford-White, X.-M. Wu, C.-Y. Wu, J.-G. Xie, J. Quan and L.-M. Zhu, JAOCS, 2010, 87, 305–311 CrossRef CAS.
- H. Cao, L. Yang, R. Tian, H. Wu, Z. Gu and Y. Li, Chem. Soc. Rev., 2022, 51, 4175–4198 RSC.
- T. Wang, J. Zhao, Z. Yang, L. Xiong, L. Li, Z. Gu and Y. Li, Green Chem., 2022, 24, 3605–3622 RSC.
- G. Bhattarai, S. B. Poudel, S.-H. Kook and J.-C. Lee, J. Biomed. Mater. Res., Part A, 2017, 105, 2510–2521 CrossRef CAS PubMed.
- B. Zhao, W. Zhang, Y. Xiong, Y. Zhang, D. Zhang and X. Xu, J. Mol. Histol., 2020, 51, 161–171 CrossRef PubMed.
- A. B. Abdel-Naim, A. A. Alghamdi, M. M. Algandaby, F. A. Al-Abbasi, A. M. Al-Abd, B. G. Eid, H. M. Abdallah and A. M. El-Halawany, Oxid.
Med. Cell. Longevity, 2018, 2018, 5106469 Search PubMed.
- E. Ikeda, Y. Ikeda, Y. Wang, N. Fine, Z. Sheikh, A. Viniegra, O. Barzilay, B. Ganss, H. C. Tenenbaum and M. Glogauer, J. Periodontol., 2018, 89, 586–595 CrossRef CAS PubMed.
- Z. He, Z. Huang, W. Zhou, Z. Tang, R. Ma and J. Liang, Front. Microbiol., 2016, 7, 1065 Search PubMed.
- Y. Khosravi, R. D. P. Kandukuri, S. R. Palmer, E. S. Gloag, S. M. Borisov, E. M. Starke, M. T. Ward, P. Kumar, D. de Beer, A. Chennu and P. Stoodley, BMC Oral Health, 2020, 20, 230 CrossRef CAS PubMed.
- W. Xie, S. Zhang, F. Pan, S. Chen, L. Zhong, J. Wang and X. Pei, J. Mater. Res., 2021, 36, 822–845 CrossRef CAS.
- M. Gómez-Florit, M. Monjo and J. M. Ramis, J. Periodontol., 2014, 85, 966–974 CrossRef PubMed.
- T. Zhou, D. Chen, Q. Li, X. Sun, Y. Song and C. Wang, Acta Odontol. Scand., 2013, 71, 349–356 CrossRef CAS PubMed.
- M. Hans, S. Prakash and S. Gupta, J. Indian Soc. Periodontol., 2012, 16, 193–199 CrossRef PubMed.
- M. V. Eberhardt, C. Y. Lee and R. H. Liu, Nature, 2000, 405, 903–904 CrossRef CAS PubMed.
- R. Bains and V. Bains, Dent. Res. J., 2015, 12, 389–405 CrossRef PubMed.
- X. Xi, Z.-X. Li, Y. Zhao, H. Liu, S. Chen and D.-X. Liu, J. Dent. Sci., 2021, 17, 750–762 CrossRef PubMed.
- H. El-Sharkawy, S. Elmeadawy, U. Elshinnawi and M. Anees, J. Periodontal Res., 2019, 54, 190–197 CrossRef CAS PubMed.
- N. K. Swarnakar, A. K. Jain, R. P. Singh, C. Godugu, M. Das and S. Jain, Biomaterials, 2011, 32, 6860–6874 CrossRef CAS.
- R. Song, Q. Wu, Z. Yun and L. Zhao, IOP Conf. Ser.: Earth Environ. Sci., 2020, 512, 012094 CrossRef.
- C. Zhang, X. Wang, J. Du, Z. Gu and Y. Zhao, Adv. Sci., 2021, 8, 2002797 CrossRef CAS.
- C. Wang and K. Liao, ACS Appl. Mater. Interfaces, 2021, 13, 56752–56776 CrossRef CAS.
- Q. Dai, H. Geng, Q. Yu, J. Hao and J. Cui, Theranostics, 2019, 9, 3170–3190 CrossRef CAS PubMed.
- Z. Wei, L. Wang, C. Tang, S. Chen, Z. Wang, Y. Wang, J. Bao, Y. Xie, W. Zhao, B. Su and C. Zhao, Adv. Funct. Mater., 2020, 30, 2002234 CrossRef CAS.
- S. Lee, J. Lee, H. Byun, S.-J. Kim, J. Joo, H. H. Park and H. Shin, Acta Biomater., 2021, 124, 166–178 CrossRef CAS PubMed.
- Y. Zhang, K. Li, L. Shen, L. Yu, T. Ding, B. Ma, S. Ge and J. Li, ACS Appl. Mater. Interfaces, 2022, 14, 268–277 CrossRef CAS PubMed.
- F. Di Cristo, A. Valentino, I. De Luca, G. Peluso, I. Bonadies, A. Calarco and A. Di Salle, Molecules, 2022, 27, 2205 CrossRef CAS PubMed.
- G. Seshadri, J. C. Sy, M. Brown, S. Dikalov, S. C. Yang, N. Murthy and M. E. Davis, Biomaterials, 2010, 31, 1372–1379 CrossRef CAS PubMed.
- Y. Wei, J. Fu, W. Wu, P. Ma, L. Ren, Z. Yi and J. Wu, Drug Des., Dev. Ther., 2021, 15, 3509–3522 CrossRef PubMed.
- M.-F. Chung, W.-T. Chia, W.-L. Wan, Y.-J. Lin and H.-W. Sung, J. Am. Chem. Soc., 2015, 137, 12462–12465 CrossRef CAS PubMed.
- Y. Liu, Y.-M. Wang, S. Sedano, Q. Jiang, Y. Duan, W. Shen, J.-H. Jiang and W. Zhong, Chem. Commun., 2018, 54, 4329–4332 RSC.
- C. Li, L. Li, S. Chen, J. Zhang and W. Lu, Front. Bioeng. Biotechnol., 2020, 8, 200 CrossRef.
- X. Zhou, J. Lv, G. Li, T. Qian, H. Jiang, J. Xu, Y. Cheng and J. Hong, Biomaterials, 2021, 268, 120600 CrossRef CAS PubMed.
- A. Arsalan and H. Younus, Int. J. Biol. Macromol., 2018, 118, 1833–1847 CrossRef CAS.
- Q. Fan, Z. Yang, Y. Li, Y. Cheng and Y. Li, Adv. Funct. Mater., 2021, 31, 2101646 CrossRef CAS.
- J.-Y. Lai and L.-J. Luo, Biomacromolecules, 2015, 16, 2950–2963 CrossRef CAS PubMed.
- P. Yang, J. Zhang, S. Xiang, Z. Jin, F. Zhu, T. Wang, G. Duan, X. Liu, Z. Gu and Y. Li, ACS Appl. Mater. Interfaces, 2021, 13, 39126–39134 CrossRef CAS PubMed.
- M. Li, H. Wang, J. Hu, J. Hu, S. Zhang, Z. Yang, Y. Li and Y. Cheng, Chem. Mater., 2019, 31, 7678–7685 CrossRef CAS.
- C. Kang, E. Jung, H. Hyeon, S. Seon and D. Lee, Nanomedicine, 2020, 23, 102104 CrossRef CAS PubMed.
- Z. Li, J. Zhang, Y. Fu, L. Yang, F. Zhu, X. Liu, Z. Gu and Y. Li, J. Mater. Chem. B, 2020, 8, 7018–7023 RSC.
- C. Liu, W. Shen, B. Li, T. Li, H. Chang and Y. Cheng, Chem. Mater., 2019, 31, 1956–1965 CrossRef CAS.
- U. G. Spizzirri, I. Altimari, F. Puoci, O. I. Parisi, F. Iemma and N. Picci, Carbohydr. Polym., 2011, 84, 517–523 CrossRef CAS.
- X. Wang, V. Parvathaneni, S. K. Shukla, N. S. Kulkarni, A. Muth, N. K. Kunda and V. Gupta, Int. J. Biol. Macromol., 2020, 164, 638–650 CrossRef CAS PubMed.
- T. Yin, L. Yang, Y. Liu, X. Zhou, J. Sun and J. Liu, Acta Biomater., 2015, 25, 172–183 CrossRef CAS PubMed.
- Z. Hong, Y. Xu, J.-F. Yin, J. Jin, Y. Jiang and Q. Du, J. Agric. Food Chem., 2014, 62, 12603–12609 CrossRef CAS PubMed.
- C. R. DeJulius, B. R. Dollinger, T. E. Kavanaugh, E. Dailing, F. Yu, S. Gulati, A. Miskalis, C. Zhang, J. Uddin, S. Dikalov and C. L. Duvall, Bioconjugate Chem., 2021, 32, 928–941 CrossRef CAS PubMed.
- Q. Wei, K. Chen, X. Zhang, G. Ma, W. Zhang and Z. Hu, Colloids Surf., B, 2022, 209, 112208 CrossRef CAS PubMed.
- M. L. Pua, T. Yoshitomi, P. Chonpathompikunlert, A. Hirayama and Y. Nagasaki, J. Controlled Release, 2013, 172, 914–920 CrossRef CAS PubMed.
- M. Saita, J. Kaneko, T. Sato, S.-S. Takahashi, S. Wada-Takahashi, R. Kawamata, T. Sakurai, M.-C.-I. Lee, N. Hamada, K. Kimoto and Y. Nagasaki, Biomaterials, 2016, 76, 292–301 CrossRef CAS PubMed.
- L. Li, J. Guo, Y. Wang, X. Xiong, H. Tao, J. Li, Y. Jia, H. Hu and J. Zhang, Adv. Sci., 2018, 5, 1800781 CrossRef PubMed.
- L. Yang, C. Wang, L. Li, F. Zhu, X. Ren, Q. Huang, Y. Cheng and Y. Li, Adv. Funct. Mater., 2022, 32, 2108749 CrossRef CAS.
- M. J. Kim, Y. Lee, S. Jon and D. Y. Lee, Biomaterials, 2017, 133, 242–252 CrossRef CAS PubMed.
- D. E. Kim, Y. Lee, M. Kim, S. Lee, S. Jon and S.-H. Lee, Biomaterials, 2017, 140, 37–44 CrossRef CAS PubMed.
- J. Y. Kim, D. Y. Lee, S. Kang, W. Miao, H. Kim, Y. Lee and S. Jon, Biomaterials, 2017, 133, 1–10 CrossRef CAS PubMed.
- Z. Wang, Y. Zou, Y. Li and Y. Cheng, Small, 2020, 16, 1907042 CrossRef CAS PubMed.
- J. Hu, L. Yang, P. Yang, S. Jiang, X. Liu and Y. Li, Biomater. Sci., 2020, 8, 4940–4950 RSC.
- M. Gómez-Florit, J. M. Ramis and M. Monjo, Biochem. Pharmacol., 2013, 86, 1784–1790 CrossRef PubMed.
- T. Sun, D. Jiang, Z. T. Rosenkrans, E. B. Ehlerding, D. Ni, C. Qi, C. J. Kutyreff, T. E. Barnhart, J. W. Engle, P. Huang and W. Cai, Adv. Funct. Mater., 2019, 29, 1904833 CrossRef CAS PubMed.
- P. Yang, Z. Gu, F. Zhu and Y. Li, CCS Chem., 2020, 2, 128–138 CrossRef CAS.
- Y. Fan, J. Zhang, Y. Shen, B. Zheng, W. Zhang and F. Huo, Nano Res., 2020, 14, 1–28 CrossRef.
- L.-L. Zhou, Q. Guan, W.-Y. Li, Z. Zhang, Y.-A. Li and Y.-B. Dong, Small, 2021, 17, 2101368 CrossRef CAS.
- L. Zhang, Y. Xiao, Q.-C. Yang, L.-L. Yang, S.-C. Wan, S. Wang, L. Zhang, H. Deng and Z.-J. Sun, Adv. Funct. Mater., 2022, 32, 2201542 CrossRef CAS.
- Y. Zou, P. Wang, A. Zhang, Z. Qin, Y. Li, Y. Xianyu and H. Zhang, ACS Appl. Mater. Interfaces, 2022, 14, 8680–8692 CrossRef PubMed.
- A. Esrafili, A. Wagner, S. Inamdar and A. P. Acharya, Adv. Healthcare Mater., 2021, 10, e2002090 CrossRef PubMed.
- N. Huang, P. Wang and D. Jiang, Nat. Rev. Mater., 2016, 1, 16068 CrossRef CAS.
- N. Singh, S. Son, J. An, I. Kim, M. Choi, N. Kong, W. Tao and J. S. Kim, Chem. Soc. Rev., 2021, 50, 12883–12896 RSC.
- S. Huang, G. Chen and G. Ouyang, Chem. Soc. Rev., 2022, 51, 6824–6863 RSC.
- M. Sharifi, K. Faryabi, A. J. Talaei, M. S. Shekha, M. Ale-Ebrahim, A. Salihi, N. M. Q. Nanakali, F. M. Aziz, B. Rasti, A. Hasan and M. Falahati, J. Mol. Liq., 2020, 297, 112004 CrossRef CAS.
- Y. Ai, Z. N. Hu, X. Liang, H. B. Sun, H. Xin and Q. Liang, Adv. Funct. Mater., 2021, 32, 2110432 CrossRef.
- F. Manea, F. B. Houillon, L. Pasquato and P. Scrimin, Angew. Chem., 2004, 43, 6165–6169 CrossRef CAS PubMed.
- L. Gao, J. Zhuang, L. Nie, J. Zhang, Y. Zhang, N. Gu, T. Wang, J. Feng, D. Yang, S. Perrett and X. Yan, Nat. Nanotechnol., 2007, 2, 577–583 CrossRef CAS PubMed.
- X. Huang, D. He, Z. Pan, G. Luo and J. Deng, Mater. Today Bio, 2021, 11, 100124 CrossRef CAS PubMed.
- Z. T. Rosenkrans, T. Sun, D. Jiang, W. Chen, T. E. Barnhart, Z. Zhang, C. A. Ferreira, X. Wang, J. W. Engle, P. Huang and W. Cai, Adv. Sci., 2020, 7, 2000420 CrossRef CAS PubMed.
- T. Liu, B. Xiao, F. Xiang, J. Tan, Z. Chen, X. Zhang, C. Wu, Z. Mao, G. Luo, X. Chen and J. Deng, Nat. Commun., 2020, 11, 2788 CrossRef CAS PubMed.
- A. A. Vernekar, D. Sinha, S. Srivastava, P. U. Paramasivam, P. D’Silva and G. Mugesh, Nat. Commun., 2014, 5, 5301 CrossRef CAS PubMed.
- H. Sun, Y. Zhou, J. Ren and X. Qu, Angew. Chem., Int. Ed., 2018, 57, 9224–9237 CrossRef CAS PubMed.
- H. Liu, J. Chen, S. Qiao and W. Zhang, ACS Biomater. Sci. Eng., 2021, 7, 4718–4735 CrossRef CAS PubMed.
- P. Choudhary, S. Biswas, N. Kandoth, D. Tayde, A. Chatterjee, S. Chattopadhyay, A. Das, S. Swarnakar and S. K. Pramanik, iScience, 2022, 25, 104062 CrossRef CAS PubMed.
- L. Zhang, L. Zhang, H. Deng, H. Li, W. Tang, L. Guan, Y. Qiu, M. J. Donovan, Z. Chen and W. Tan, Nat. Commun., 2021, 12, 2002 CrossRef CAS PubMed.
- J. Gao, Y. Liu, B. Jiang, W. Cao, Y. Kan, W. Chen, M. Ding, G. Zhang, B. Zhang, K. Xi, X. Jia, X. Zhao and H. Guo, ACS Appl. Mater. Interfaces, 2020, 12, 31745–31756 CrossRef CAS PubMed.
- J. He, X. Zhu, Z. Qi, C. Wang, X. Mao, C. Zhu, Z. He, M. Li and Z. Tang, ACS Appl. Mater. Interfaces, 2015, 7, 5605–5611 CrossRef CAS PubMed.
- J. Park, S. Park, J. E. Kim, K.-J. Jang, H. Seonwoo and J. H. Chung, Polymers, 2021, 13, 797 CrossRef CAS PubMed.
- K. Kawamoto, H. Miyaji, E. Nishida, S. Miyata, A. Kato, A. Tateyama, T. Furihata, K. Shitomi, T. Iwanaga and T. Sugaya, Int. J. Nanomed., 2018, 13, 2365–2376 CrossRef CAS PubMed.
- O. Tao, D. T. Wu, H. M. Pham, N. Pandey and S. D. Tran, Appl. Sci., 2019, 9, 317 CrossRef CAS.
- Y. Li, L. Yang, Y. Hou, Z. Zhang, M. Chen, M. Wang, J. Liu, J. Wang, Z. Zhao, C. Xie and X. Lu, Bioact. Mater., 2022, 18, 213–227 CrossRef CAS PubMed.
- F. Wang, L. Chen, D. Liu, W. Ma, P. Dramou and H. He, TrAC, Trends Anal. Chem., 2020, 133, 116080 CrossRef CAS.
- J. Yang and Y. W. Yang, Small, 2020, 16, e1906846 CrossRef PubMed.
- H. Zhang, G. Li, C. Liao, Y. Cai and G. Jiang, J. Mater. Chem. B, 2019, 7, 2398–2420 RSC.
- X. Niu, X. Li, Z. Lyu, J. Pan, S. Ding, X. Ruan, W. Zhu, D. Du and Y. Lin, Chem. Commun., 2020, 56, 11338–11353 RSC.
- Y. Tian, Y. Li, J. Liu, Y. Lin, J. Jiao, B. Chen, W. Wang, S. Wu and C. Li, Bioact. Mater., 2021, 9, 428–445 CrossRef PubMed.
- W. Zhang, S. Hu, J. J. Yin, W. He, W. Lu, M. Ma, N. Gu and Y. Zhang, J. Am. Chem. Soc., 2016, 138, 5860–5865 CrossRef CAS PubMed.
- M. A. Busquets and J. Estelrich, Drug Discovery Today, 2020, 25, 1431–1443 CrossRef CAS PubMed.
- S. Li, X. Liu, H. Chai and Y. Huang, TrAC, Trends Anal. Chem., 2018, 105, 391–403 CrossRef CAS.
- L. Zhang, Y. Zhang, Z. Wang, F. Cao, Y. Sang, K. Dong, F. Pu, J. Ren and X. Qu, Mater. Horiz., 2019, 6, 1682–1687 RSC.
- S. S. Nadar, L. Vaidya, S. Maurya and V. K. Rathod, Coord. Chem. Rev., 2019, 396, 1–21 CrossRef CAS.
- R. M. L. Chye, V. Perrotti, A. Piattelli, F. Iaculli and A. Quaranta, Implant Dent., 2019, 28, 74–85 CrossRef PubMed.
- B. Li, L. Shi, R. Liu, Z. Li, S. Cao and J. Li, J. Mater. Chem. B, 2021, 9, 8694–8707 RSC.
- A. F. S. Shivang and A. Chaudhary, Expert Opin. Drug Delivery, 2010, 7, 871–885 CrossRef PubMed.
- J. Luan, R. Li, W. Xu, H. Sun, Q. Li, D. Wang, S. Dong and J. Ding, Acta Pharm. Sin. B, 2022 DOI:10.1016/j.apsb.2022.10.026.
- Q. Wei, Y. Su, H. Xin, L. Zhang, J. Ding and X. Chen, ACS Appl. Mater. Interfaces, 2021, 13, 56719–56724 CrossRef CAS PubMed.
- R. Li, K. Liu, X. Huang, D. Li, J. Ding, B. Liu and X. Chen, Adv. Sci., 2022, 9, 2105152 CrossRef CAS PubMed.
- X. Zheng, F. Zhang, K. Wang, W. Zhang, Y. Li, Y. Sun, X. Sun, C. Li, B. Dong, L. Wang and L. Xu, TrAC, Trends Anal. Chem., 2021, 140, 116281 CrossRef CAS.
- X. Li, C. Luo, Q. Fu, C. Zhou, M. Ruelas, Y. Wang, J. He, Y. Wang, Y. S. Zhang and J. Zhou, Adv. Mater., 2020, 32, e2000060 CrossRef PubMed.
|
This journal is © The Royal Society of Chemistry 2023 |
Click here to see how this site uses Cookies. View our privacy policy here.