Levels of per- and polyfluoroalkyl substances (PFAS) in various wastewater-derived fertilizers – analytical investigations from different perspectives†
Received
30th June 2023
, Accepted 7th September 2023
First published on 8th September 2023
Abstract
Solid wastewater-based fertilizers were screened for per- and polyfluoroalkyl substances (PFAS) by the extractable organic fluorine (EOF) sum parameter method. The EOF values for ten sewage sludges from Germany and Switzerland range from 154 to 7209 μg kg−1. For thermal treated sewage sludge and struvite the EOF were lower with values up to 121 μg kg−1. Moreover, the application of PFAS targeted and suspect screening analysis of selected sewage sludge samples showed that only a small part of the EOF sum parameter values can be explained by the usually screened legacy PFAS. The hitherto unknown part of EOF sum parameter contains also fluorinated pesticides, pharmaceutical and aromatic compounds. Because these partly fluorinated compounds can degrade to (ultra-)short PFAS in wastewater treatment plants they should be considered as significant sources of organic fluorine in the environment. The combined results of sum parameter analysis and suspect screening reveal the need to update current regulations, such as the German fertilizer ordinance, to focus not solely on a few selected PFAS such as perfluorooctane sulfonic acid (PFOS) and perfluorooctanoic acid (PFOA) but consider an additional sum parameter approach as a more holistic alternative. Moreover, diffusion gradient in thin-films (DGT) passive samplers were utilized as an alternative simplified extraction method for PFAS in solid wastewater-based fertilizers and subsequently quantified via combustion ion chromatography. However, the DGT method was less sensitive and only comparable to the EOF values of the fertilizers in samples with >150 μg kg−1, because of different diffusion properties for various PFAS, but also kinetic exchange limitations.
Environmental significance
Persistent per- and polyfluoroalkyl substances (PFAS) have been previously detected in soils and sediments which can be attributed to the application of sewage sludge and industrial by-products as fertilizers. Through the amendment of the Sewage Sludge Ordinance in 2017 the German legislation banned sewage sludge/biosolid application on agricultural land, and by 2029, sludge will be completely prohibited from agricultural application. Currently, plant-available phosphate-fertilizers from sewage sludge/wastewater can be produced using a variety of treatment techniques. However, the fate of legacy and emerging PFAS compounds during these treatment from sewage sludge and wastewater is still unknown. Therefore, various wastewater-based fertilizers were screened with various analytical techniques to understand the PFAS emission in environmental media.
|
Introduction
Per- and polyfluoroalkyl substances (PFAS) are synthetic chemical and include more than 10
000 compounds.1 PFAS have been used extensively in a variety of products and industries due to their inert chemical stability and resistance to degradation by heat or acids.2 Thousands of industrial and military installations have been found to contain contaminated soil surfaces and groundwater resources.3 Furthermore, as a result of the continuous use of PFAS containing consumer products, effluents and sewage sludge from wastewater treatment plants (WWTPs) have been observed to be an important pathway for PFAS into the environment.4–14 In addition to direct discharge of PFAS through effluents, sewage sludge has been observed to be an important pathway for PFAS release to agricultural soils.15–21
The German legislation banned sewage sludge/biosolid application on agricultural land through the amendment of the German fertilizer ordinance22 and by 2029 sewage sludge will be totally prohibited from agricultural application. While environmental exposure of organic pollutants like PFAS and antibiotics is no longer desirable, phosphorus (P) from sewage sludge should still be recycled in WWTPs of cities with a population larger than 50
000 residents. To produce high-quality P-fertilizers for a circular economy, German sewage sludge has to undergo treatment.23 Currently, plant-available P-fertilizers from recycled materials (e.g. sewage sludge, sewage sludge ash, wastewater precipitates) can be produced using a variety of treatment approaches including precipitation, leaching, and thermal treatment.24,25 During thermal treatment, the sewage sludge is heated to varying temperatures to destroy pathogens and organic matter. Since there is a lack of universal regulation, some thermal treatments (e.g. low-temperature conversion/pyrolysis) are conducted at temperatures of 400–500 °C which might not be sufficient for a complete PFAS decomposition. Other incineration techniques may reach higher temperatures in the range of 850–900 °C, at which most PFAS decompose.26,27 However, in the absence of hydrogen sources, incomplete degradation takes place, yielding shorter chained and more volatile PFAS.26,28
Currently, only the sum amount of perfluorooctane sulfonic acid (PFOS) and perfluorooctanoic acid (PFOA) is monitored via the German fertilizer ordinance23 with a limit of 100 μg per kg dry matter by German regulation. Due to the strong diversity of industrial PFAS usage29 this limitation is not adequate to ensure safe application of novel recycled P-fertilizers from WWTPs on agricultural fields. Moreover, wastewater and sewage sludge contain also fluorinated pesticides30 and pharmaceuticals31,32 which potentially break down to form ultrashort PFAS in WWTPs.33 In addition, the European Union has proposed to ban the use of all (non-essential) PFAS to reduce pollutant entry to the environment.34
State-of-the-art method liquid chromatography tandem mass spectrometry (LC-MS/MS) is the most comprehensive tool for characterizing many PFAS in environmental samples. However, the high number of compounds in this group renders this type of comprehensive analysis with justifiable efforts prohibitive. In order to determine the concentration of “total” PFAS in wastewater-based fertilizers, novel PFAS screening methods are needed. In previous work of several researchers, the sum parameter extractable organic fluorine (EOF) by combustion ion chromatography (CIC) was successfully applied for water, sewage sludges, sediments and soil samples.5,19,35
In this work, our goal was on the one hand to analyse PFAS in wastewater-based fertilizer using EOF coupled to CIC for “total” PFAS followed by comparing subset of results with classical LC-MS/MS target analysis, and one selected sample by HR-MS suspect screening. On the other hand, we compared the EOF method with diffusive gradients in thin-films (DGT) passive sampler,36 which also can be used for extraction of solid–liquid mixtures (e.g. soil, sewage sludge)37 (see Fig. 1).
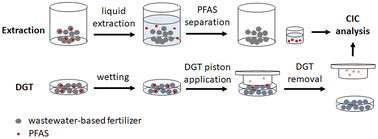 |
| Fig. 1 Schematic PFAS chemically extraction (top) versus extraction with DGT piston (bottom). | |
Because the DGT technique is based on diffusion, in contrast to chemically extraction of the EOF method, this technique might underestimate the amount of PFAS due to sorption and resupply on sludge particles. Moreover, the PFAS adsorption on the DGT binding layer was investigated via Fourier-transform infrared (FT-IR) and fluorine K-edge X-ray absorption near-edge structure (XANES) spectroscopy, respectively.
Materials and methods
Sampling
Ten dried sewage sludge (SL) samples from various wastewater treatment plants in Germany and Switzerland, six sewage sludge ashes (SSA) from Germany, six thermally treated SL and SSA samples with different additives (temperatures: 700–1050 °C), two low-temperature converted (LTC) SL samples (pyrolysis, temperature: 400 °C)38 and two struvite samples from Germany and Canada were analysed in this study.
Chemicals and reagents
For the EOF extraction process ammonia (25%, Suprapur®, Merck KGaA), formic acid (99–100%, Chemsolute), methanol (MeOH; 99.98%, Rotisolv® HPLC grade, Roth Chemicals), n-hexane (Suprasolv, Supelco) and acetone (99.5%, p.a., Chemsolute) were used. A Labostar DI 2 system (Siemens Evoqua Water Technologies GmbH) generating ultra-pure water (<0.6 μS cm−1), was used for all applications and CIC experiments. All utilized solid-phase extraction (SPE) cartridges that were preassembled Strata-PFAS containing a weakly ion exchange (WAX) resin/graphitized carbon black (GCB) combination, purchased by Phenomenex Ltd., Germany. Furthermore, sodium trifluoromethanesulfonate (Na-TFMS, 98%, BLD Pharma), perfluorooctanesulfonic acid (PFOS, 97%, abcr), sodium trifluoroacetate (Na-TFA, 98%, Alfa Aesar), perfluorooctanoic acid (PFOA, 96%, Sigma-Aldrich), perfluoro-2-propoxypropanoic acid (HFPO-DA, 97%, abcr; carbonic acid of “GenX”), 1H,1H,2H,2H-perfluorooctanephosphonic acid (PFOPA, 95%, Sigma-Aldrich) were used for the DGT experiments and KF solution (fluoride standard solution 1.000 mg F per L KF in water, Berndkraft) for the CIC calibration.
Sample extraction and preparation for quantitative EOF analysis
For determination of the sum parameter EOF, all samples were extracted and prepared in triplicate according to our previously reported work.19 Detailed information can be found in the ESI.†
Sum parameter analysis (EOF and DGT)
All extraction samples (EOF and DGT) were quantified by CIC similar to previously reported work.19 The methanol extracts of all samples (500 μL) were injected into quartz wool filled ceramic boats before being measured by CIC. All samples were measured in duplicates to maintain quality in data consistency. In order to quantify the correct absorption volume, an internal standard of known concentration was added to the absorption solution before each combustion step. Quantification of the samples was enabled using an eleven-point calibration curve from 1 to 20 μg per L F− (R2 = 0.995) for low fluoride containing samples and a six-point curve from 10 to 500 μg per L F− (R2 = 0.999) for higher fluoride value detection. The calibration solutions were prepared from KF stock solution and calibration was performed by combustion of the respective aliquots via the CIC instrument. All EOF values are given in μg per kg dry weight.
Targeted PFAS analysis by LC-MS/MS
Quantitative LC-MS/MS analysis was performed for the SL samples 1, 4, 5, 6, 8 and 10 according to German standard DIN 38414-14:2011-08,39 since they revealed elevated levels of EOF per sample. Therefore, 0.5 g of each SL sample was weighed in conical polypropylene (PE) tubes and subsequently extracted with 2.5 mL methanol in an ultra-sonic bath for 30 min. Prior to that, 50 μL of the 13C-labelled recovery standard solution (Wellington Laboratories, Canada) was added. After settling for 1 h, an 500 μL aliquot of the supernatant was filtered using a 0.45 μm cellulose filter and diluted with 500 μL ultra-pure water (Milli-Q). All samples were extracted in duplicates.
Analyses for SL samples were performed using an Agilent 1260 HPLC and an AB SCIEX TSQ 6500 as mass selective detector. 7 μL of the samples were injected and separated on a Nucleodur C18 Pyramid pre-column (8 mm × 3 mm, 3 μm) and a Nucleodur C18 Pyramid column (125 mm × 2 mm; 3 μm) (both Macherey-Nagel, Düren, Germany) at 35 °C and a flow rate of 0.3 mL min−1 using the following gradient program using water with 10 mM ammonium acetate solution (eluent A) and methanol (eluent B): the eluent composition of 75% A and 25% B at the beginning changed at 9 min to 25% A and 75% B. The ion source was operated at 425 °C and with an ion spray voltage of 1200 V and all measurements were executed in the multireaction mode (MRM).
Suspect screening by liquid chromatography-high resolution mass spectrometry (LC-HRMS)
The EOF extract from SL1 was additionally subjected to a suspect screening using a UPLC-HRMS-MS setup (Agilent 1290 ultra-performance liquid chromatography coupled to Sciex TripleTOF 6500 high resolution mass spectrometer) in negative ESI mode to identify further PFAS and organofluorine compounds. A suspect list of PFAS was compiled from a compound library provided by MS-Dial (MSMS-Public-Neg-VS15.msp, n = 36
860; https://prime.psc.riken.jp/compms/msdial/main.html#MSP) and a target list provided by EPA (list_chemicals-2021-06-07-15-56-49.tsv, n = 597, https://comptox.epa.gov/dashboard/chemical-lists). The MS-Dial software (https://prime.psc.riken.jp/compms/msdial/main.html) was used together with in-house scripts (R, https://www.R-project.org) for data processing and suspect screening.
Diffusive gradients in thin-films (DGT) extraction
DGT devices (window size: 2.54 cm2; 0.8 mm agarose diffusion layer) with a weak anion exchanger (WAX; thickness 0.5 mm) binding layer36 from DGT Research, Lancaster, UK were used for the experiments. The fertilizer samples were incubated at 100% water holding capacity for one hour, then the DGT devices were deployed in duplicate on the samples for 24 h at 23 °C. After deployment, DGTs were removed from the samples and disassembled, and the binding layers were obtained and eluted in 3 mL of MeOH for 24 h. The separated eluates were slowly evaporated to dryness by N2 gas and subsequently reconstituted in 1.5 mL of fresh MeOH. Blank DGT devices were kept in the refrigerator until the processing and extraction of the DGT devices.
Furthermore, DGT devices were loaded with 200 mL solutions (50 mg F per L) of various PFAS compounds (Na-TFMS, PFOS, Na-TFA, PFOA, HFPO-DA and PFOPA). The DGT devices were deployed for 24 h at 23 °C in plastic bakers in constantly agitated solutions. After deployment, the binding layers of the DGT devices were dried at room temperature and spectroscopically investigated as described below.
Fourier-transform infrared (FT-IR) spectroscopy
FT-IR spectra of the DGT binding layers were collected with the PerkinElmer 2000 FT-IR spectrometer. The binding layers were measured with the SensIR DuraSamplIR II attenuated total reflection (ATR) module (spectral resolution 8 cm−1; 32 scans were coadded per spectrum). All FT-IR spectra were blank-subtracted and normalized (min–max to 1495–1382 cm−1 region) with the software OPUS (Bruker, Version 7.0).
Fluorine K-edge X-ray absorption near-edge structure (XANES) spectroscopy
Fluorine K-edge XANES spectra of the DGT binding layers were collected on the PHOENIX II beamline of the Swiss Light Source (SLS, Villigen, Switzerland). The experiments were conducted at room temperature under a high vacuum (10−6 mbar). Bulk-XANES spectra were collected from an area of approx. 2 × 3 mm at the sample over the range 660–780 eV in fluorescence mode, using a silicon drift diode (SDD, manufacturer: Ketek). The collected spectra were normalized, and background corrected using the Athena software from the Demeter 0.9.26 package (Ravel and Newville 2005).
Results and discussion
Extractable organic fluorine (EOF) of wastewater-based fertilizers
First the EOF values of all 26 wastewater-based fertilizers were analysed. Fig. 2, Tables S1 and S2† show the EOF and DGT values of the various SLs and wastewater-based fertilizers. The DGT values will be discussed in the fourth subsection.
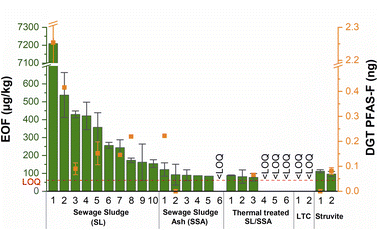 |
| Fig. 2 EOF (green bars; μg kg−1) and DGT results (orange points; ng PFAS–F per binding layer) of various fertilizers from wastewater-based materials. The LOQ is for the EOF values. The exact standard deviation of SL1 is displayed in Fig. 5 and Table S1.† From SSA4 and SS5 two samples (EOF) out of the triplicate were negative after blank correction, hence, values were omitted. Some of the EOF values were published previously19 – see Table S1;† from SL2, SL8 and SSA1 one sample each (DGT) was negative after blank correction, hence, values were omitted. LTC = low-temperature conversion (pyrolysis). | |
The EOF values of the SLs mainly range between 154 and 538 μg kg−1 except for SL1 which showed an elevated EOF value of 7209 μg kg−1 due to high PFAS and other organofluoride contamination. These EOF values are in good agreement with EOF values for previously reported north European SL samples.5 For the SSA samples the EOF values were lower and values between LOQ (approx. 60 μg kg−1) and 121 μg kg−1 could be detected. From SSA4 and SSA5 two samples out of the triplicate were negative after blank correction. The first three SSA samples (SSA1 to SSA3) were taken from a grate firing incinerator and samples SSA4 to SSA6 after incineration via fluidized bed combustion. This is an indication that the type of SL incineration may affect the PFAS degradation rate. Additionally, we analysed the activated carbon adsorber of the off-gas cleaning after the electric precipitator from the mono-incineration facility SSA4.40 This material was quantified with an EOF value of 157.9 ± 10.4 μg kg−1 which indicates that after incineration some organofluoride compounds are still detectable in the off-gas stream.
The various fertilizers derived from thermal treatment of SLs and SSAs, partly contain organo fluorinated compounds with EOF values up to 88 μg kg−1. For the pyrolyzed SLs from LTC no EOF values above the LOQ could be detected. Moreover, the two wastewater-based struvite fertilizers contain 96 and 112 μg per kg EOF, respectively. Altogether, relevant amounts of EOF could be systematically detected in almost all types of fertilizers from wastewater.
Sum parameter analysis EOF vs. targeted PFAS analysis
The EOF parameter provides a good overview on the amount of PFAS and other fluorinated compounds in SLs and wastewater-based fertilizers. However, it cannot give any information on the specific type of PFAS in these fertilizers. In addition, the German fertilizer ordinance23 only prescribes a limit value based on the sum of the PFAS targets PFOS and PFOA. Therefore, several selected SL samples were analysed with regard to PFAS targets according to German Standard DIN 38414-14:2011-08,39 presented in Fig. 3 and Table S3.†
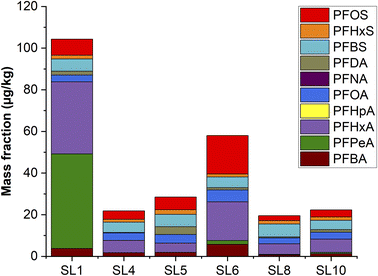 |
| Fig. 3 Targeted analysis by LC-MS/MS according to standard DIN 38414-14:2011-08 (ref. 39) of selected PFAS in sewage sludge (in μg kg−1). | |
In contrast to the EOF values, the sum of PFAS target values are relatively low for all SLs. It has to be noted that the EOF values refer to the amount of fluorine in the extract solution. Since many PFAS contain approx. 60% (w/w) fluorine the EOF values of PFAS would be expected to be higher. Surprisingly, the sum of PFOS and PFOA for SL1 is only approx. 11 μg kg−1 even if the EOF has a very high value of 7209 μg kg−1. However, this agrees with previous examples of Aro et al.5 who showed that only a small portion of organofluorides can be identified in SLs from several north European countries. Moreover, all analysed SLs in the present study would be opened to be used as fertilizers in Germany since their values lie below the ordinance limit of 100 μg kg−1 (sum of PFOS and PFOA). Since the total sum of all analysed PFAS of SL1 by LC-MS/MS was approx. 104 μg kg−1 compared to its EOF value (7209 μg kg−1), we applied a LC-HRMS based suspect screening approach for qualitative identification of additional PFAS and other fluorinated compounds in this SL sample.
PFAS suspect screening analysis
The PFAS suspect screening approach aimed to tentatively identify PFAS that could contribute to the hitherto unknown part of the EOF value. Fig. 4 shows the compound classes of identified PFAS and fluorinated compounds in SL1, while Table S4† lists the individual identified compounds.
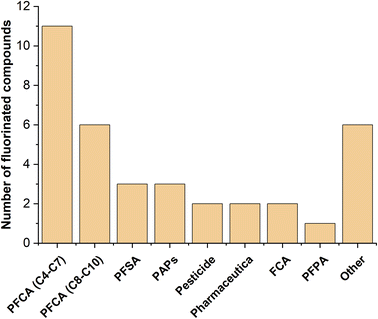 |
| Fig. 4 Determined compound classes through suspect screening of SL1; PFCA = perfluorocarboxylic acids, PFSA = perfluorosulfonic acids, PAPs = mono- and dipolyfluoroalkyl phosphate esters, FCA = fluorine containing aromatics, PFPA = perfluorophosphonic acids. | |
The majority of the detected fluorinated compounds are legacy PFAS such as short- and long-chain perfluorocarboxylic acids (PFCA), perfluorosulfonic acids (PFSA), polyfluoroalkyl phosphate esters (PAPs) and perfluorophosphonic acids (PFPA). Furthermore, the emerging PFAS hexafluoropropylene oxide dimer acid (HFPO-DA, the acid of GenX) could be identified and was classified as “others” (see Table S4†). Moreover, fluorinated pesticides (flufenacet and fludioxonil), pharmaceutical (flufenamic acid and pitavastatin) as well as aromatic compounds (4,4′-difluorobenzophenone and 1-cyclopropyl-6,7-difluoro-1,4-dihydro-8-hydroxy-4-oxo-3-quinoline-3-carboxylic acid) were also identified, which are all included in the EOF parameter. Our findings agree well with previous work of others18,41,42 who were able to detect a broad range of different PFAS and fluorinated compounds in SLs/biosolids from France, Sweden and the US by LC-HRMS suspect screening.
Diffusive gradient in thin-films (DGT) technique for PFAS extraction
Moreover, the DGT technique was applied for PFAS extraction from the wastewater-based fertilizers. Fig. 2 and Table S2† show the PFAS-DGT values for selected fertilizers (orange spots). In contrast to the EOF values which are stated as weight in mass concentration of the sample, the PFAS-DGT values are presented in absolute values of ng PFAS–fluorine per DGT binding layer. Similar to the EOF value, the DGT extraction of SL1 yielded the highest amount of 2.2 ng absolute fluorine.
Overall, there is a correlation between the EOF and the PFAS-DGT values which has a correlation coefficient R2 of 0.98 (see Fig. 5). Since for both applied extraction methods (EOF and DGT) weak anion exchange resin-based materials (WAX; for EOF a combination of WAX and graphitized carbon black) were used, a comparable pool of PFAS and fluorinated compounds was accessed in the fertilizer samples. However, the R2-value of the correlation in Fig. 5 is highly dependent on the value of SL1. Without SL1 the R2-value is only 0.42. Because wastewater-based fertilizers contain various PFAS42 and different PFAS have different diffusion properties this could be an explanation for the dispersion of the DGT values. Moreover, DGT is sampling the freely available/labile portion of PFAS in the sample and Huang et al.43 showed that pH and texture of a samples are important properties which controls the labile pool size of PFAS. They showed also that the rate of supply of PFAS to DGT devices in soil was controlled by the kinetics of release from the solid phase to the solution phase, which may also apply for wastewater-based fertilizers.
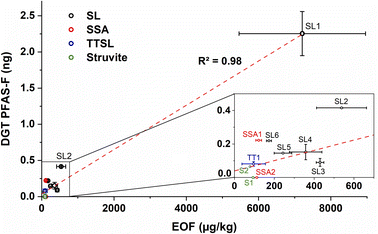 |
| Fig. 5 Correlation between EOF (in μg kg−1) and PFAS-DGT (ng PFAS–F on binding layer) of various wastewater-based fertilizers; SL = sewage sludge, SSA = sewage sludge ash, TTSL = thermal treated sewage sludge (ash). All error bars correspond to the respective standard deviations (see also Table S2†). | |
The kinetic exchange limitations for PFAS of the DGT method might be also the reason that for some wastewater-based fertilizers (SSA2, the thermal treated SL (TT1) and struvite1) the DGT method did not show results above the blank DGT value of approx. 0.11 ng per binding layer which correspond to an EOF value of approx. 150 μg kg−1. Therefore, the EOF method is in this case more sensitive than the DGT method.
FT-IR spectroscopy of PFAS loaded DGT binding layers
In order to prove that PFAS and fluorinated organic compounds adsorb to the WAX containing binding layer of the DGT device, several approaches for direct spectroscopic analysis on the binding layer are known.44,45 Therefore, the DGT devices were saturated using various aqueous PFAS solutions (all 50 mg F per L). Afterwards the binding layers were dried and spectroscopically investigated by FT-IR and fluorine K-edge XANES spectroscopy, respectively. Fig. 6 (top) shows the FT-IR spectra of various PFAS adsorbed to the DGT binding layer where the blank binding layer spectra was subtracted (the raw data are presented in Fig. S1†).
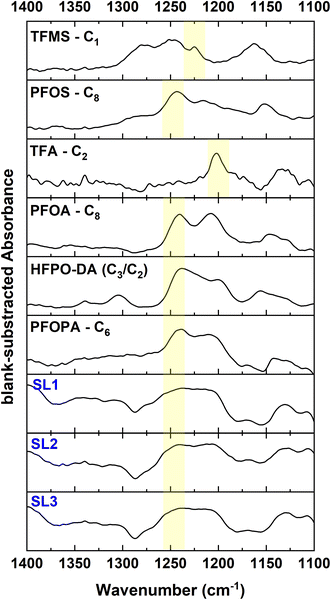 |
| Fig. 6 Normalized and background subtracted FT-IR spectra of loaded DGT binding layers with PFAS (top) and DGT binding layers applied to wetted SL samples (bottom). The strong ν(CF2) and ν(CF3) band, respectively, is marked in yellow. Spectral cutout from 1400 to 1100 cm−1. | |
The ultrashort-chain PFAS compounds TFMS and TFA show a lower signal-to-noise ratio in the FT-IR spectrum compared to the long-chain PFAS which indicate a lower amount of adsorbed PFAS to the DGT binding layer. All PFAS (except TFA and TFMS) show a strong ν(CF2) band at approx. 1250 cm−1, which is in good agreement with previous works.46,47 In opposition, TFA and TFMS show the strong ν(CF3) band at lower wavenumber of approx. 1203 cm−1 and 1223 cm−1, respectively, which also agrees with previous findings.48,49 Furthermore, there are other detectable IR bands of each PFAS which belong to the functional groups of each molecule.46,50 For example, the IR band at 1215 cm−1 of the DGT-PFOS spectrum can be attributed to the R–SO3 vibration of the sulfonate group.46 Thus, FT-IR spectroscopy is a viable method to identify pure PFAS compounds adsorbed to the DGT binding layer.
Additionally, the DGT devices were deployed to the sludges SL1 to SL3, because they have the highest EOF values, and the binding layers investigated by FT-IR spectroscopy (see Fig. 6 bottom). As observed before, all three samples show a ν(CF2) vibration around 1250 cm−1. However, the additional IR bands of the three SL samples are identical and may be attributed to other organic molecules in the SL. Therefore, it was impossible to identify individual PFAS compounds in the SLs by the DGT/FT-IR spectroscopy approach.
Fluorine K-edge XANES spectroscopy of loaded DGT-layers
As reported previously by Yan et al.51 PFAS adsorption to clay can be investigated by means of surface spectroscopic methods such as the scanning transmission X-ray microscopy (STXM)-XANES technique. Based on these results, we investigated the PFAS adsorption to the saturated DGT binding layer also by fluorine K-edge bulk-XANES spectroscopy (see Fig. 7), because it is surface sensitive with a penetration depth of max. 3 μm at the fluorine edge energy.
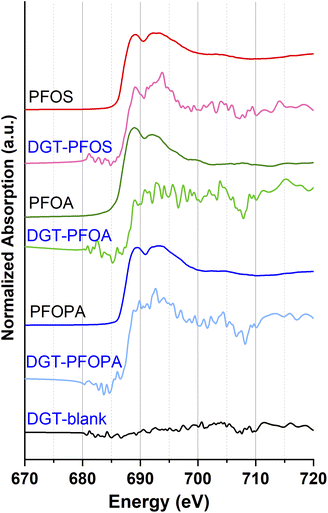 |
| Fig. 7 Fluorine K-edge bulk-XANES spectra of loaded DGT binding layers with PFAS and corresponding PFAS reference compounds. | |
However, for the saturated DGT binding layer with the ultrashort-chain PFAS TFMS, TFA and HFPO-DA no spectra could be collected. One possible reason is that due to the ultra-high vacuum these volatile PFAS evaporate quickly. Moreover, the radiation beam warms up the sample which probably enhance the evaporation. Hence, only for the PFAS compounds PFOS, PFOA and PFOPA–fluorine K-edge XANES spectra could be collected for the loaded binding layers. Although the signal-to-noise ratio is rather low, the fluorine edge of these samples is higher than the one of the DGT blank. Therefore, the presence of an organic fluorine compound on the DGT binding layer could be confirmed.19 Thus, for non-IR transparent samples, like activated carbon, the fluorine K-edge XANES spectroscopy technique is a useful tool to analyse the adsorption of PFAS.
Conclusions
Currently, different treatment methods for nutrient recycling from WWTPs are under development in Germany. Both EOF, as well as target analytical data showed that SLs and currently available wastewater-based fertilizers contain PFAS. Additional LC-HRMS suspect screening indicate that SLs contain a broad range of PFAS and other fluorinated organic compounds, such as common pesticides and pharmaceuticals.
PFAS sum parameter (e.g. EOF/CIC) and suspect screening by LC-HRMS are useful techniques to identify PFAS contaminations in fertilizer samples, especially for research. Target analysis is normally applied for quantification of specific PFAS and used for regulation. But since the number of known PFAS already exceeds 10
000, the currently ordinance limit of 100 μg per kg PFOS + PFOA in the German Fertilizer Ordinance is no longer up to date. With the planned REACH restriction of the EU that is covering the use of all PFAS (except those of essential use) it should also be considered to use a PFAS sum parameter limit in some form for fertilizer regulation.5,52 As an example, an approach for “total” PFAS was already implemented in the EU drinking water directive.53 The sum parameter EOF includes besides legacy PFAS also fluorinated pesticides and pharmaceuticals, which can also end up as ultrashort-chain PFAS in the WWTPs.33 Other sum parameters like the total oxidisable precursor assay (TOPA), do not include fluorinated pesticides and pharmaceuticals but are limited on available 13C-marked internal standards for LC-MS/MS.
Furthermore, we presented the potential of the simplified liquid extraction with the DGT passive sampler as a monitoring tool for PFAS in wastewater-based fertilizers, when DGT extraction gets analysed with CIC analysis. However, in comparison with the EOF/CIC method the DGT/CIC values were more widely spread because wastewater-based fertilizers contain various PFAS including fluorinated pharmaceutical and pesticides42 which have different diffusion properties, but also kinetic exchange limitations have been observed for the DGT method previously.43 Therefore, the DGT approach has the advantage that almost no sample preparation is necessary, but the EOF method is more sensitive to analyse low amounts of “total” PFAS in wastewater-based fertilizers.
Moreover, we found that thermal treatment of SL in different form54 reduces the amount of PFAS in the fertilizer product, however, there is still PFAS pollution detectable to some extent. The lowest PFAS values were detected for the pyrolyzed products which is also in good agreement with previous studies.55,56 However, it has to be kept in mind that thermal treatment of PFAS containing fertilizers may produce volatile ultrashort-chain PFAS57,58 or organofluoride compounds like CF4, CHF3etc.59 which have a high global warming potential, and may afterwards enter the atmosphere, depending on the used filter systems in thermal treatment plants.26,28
Author contributions
CV – corresponding author, formal analysis, writing original draft, conceptualization, data curation, validation, visualization. PR – formal analysis, writing – review & editing. PW – data curation, writing – review & editing. CP – formal analysis, data curation, writing – review & editing. SK – formal analysis, data curation, writing – review & editing. JL – formal analysis, data curation, writing – review & editing. TS – formal analysis, data curation, writing – review & editing. HH – data curation, writing – review & editing. TH – formal analysis, data curation, writing – review & editing. CB – formal analysis, data curation, writing – review & editing. FGS – data curation, writing – review & editing, supervision.
Conflicts of interest
There are no conflicts to declare.
Acknowledgements
We thank Maren Riedel (BAM) for the EOF extractions of the wastewater-based fertilizers. The authors thank the German Federal Ministry of Economic Affairs and Climate Action (BMWK; ZIM program 16KN076702 “PerFluSan-PFTSan” and 16KN076724 “MIDRAPA”) for funding. Furthermore, we acknowledge the Paul Scherrer Institute, Villigen, Switzerland for the provision of synchrotron radiation beamtime at PHOENIX beamline of the Swiss Light Source.
References
- Z. Wang, A. M. Buser, I. T. Cousins, S. Demattio, W. Drost, O. Johansson, K. Ohno, G. Patlewicz, A. M. Richard, G. W. Walker, G. S. White and E. Leinala, A New OECD Definition for Per- and Polyfluoroalkyl Substances, Environ. Sci. Technol., 2021, 55(23), 15578–15578, DOI:10.1021/acs.est.1c06896.
- A. B. Lindstrom, M. J. Strynar and E. L. Libelo, Polyfluorinated Compounds: Past, Present, and Future, Environ. Sci. Technol., 2011, 45, 7954–7961, DOI:10.1021/es2011622.
- N. Bolan, B. Sarkar, Y. Yan, Q. Li, H. Wijesekara, K. Kannan, D. C. W. Tsang, M. Schauerte, J. Bosch, H. Noll, Y. S. Ok, K. Scheckel, J. Kumpiene, K. Gobindlal, M. Kah, J. Sperry, M. B. Kirkham, H. Wang, Y. F. Tsang, D. Hou and J. Rinklebe, Remediation of poly- and perfluoroalkyl substances (PFAS) contaminated soils – to mobilize or to immobilize or to degrade?, J. Hazard. Mater., 2021, 401, 123892, DOI:10.1016/j.jhazmat.2020.123892.
- C. Gallen, A. Bignert, G. Taucare, J. O'Brien, J. Braeunig, T. Reeks, J. Thompson and J. F. Mueller, Temporal trends of perfluoroalkyl substances in an Australian wastewater treatment plant: a ten-year retrospective investigation, Sci. Total Environ., 2022, 804, 150211, DOI:10.1016/j.scitotenv.2021.150211.
- R. Aro, U. Eriksson, A. Kärrman, F. Chen, T. Wang and L. W. Y. Yeung, Fluorine Mass Balance Analysis of Effluent and Sludge from Nordic Countries, ACS ES&T Water, 2021, 1, 2087–2096, DOI:10.1021/acsestwater.1c00168.
- J. Semerád, N. Hatasová, A. Grasserová, T. Černá, A. Filipová, A. Hanč, P. Innemanová, M. Pivokonský and T. Cajthaml, Screening for 32 per- and polyfluoroalkyl substances (PFAS) including GenX in sludges from 43 WWTPs located in the Czech Republic – evaluation of potential accumulation in vegetables after application of biosolids, Chemosphere, 2020, 261, 128018, DOI:10.1016/j.chemosphere.2020.128018.
- T. Stahl, M. Gassmann, S. Falk and H. Brunn, Concentrations and Distribution Patterns of Perfluoroalkyl Acids in Sewage Sludge and in Biowaste in Hesse, Germany, J. Agric. Food Chem., 2018, 66, 10147–10153, DOI:10.1021/acs.jafc.8b03063.
- M. Wilhelm, M. Kraft, K. Rauchfuss and J. Hölzer, Assessment and Management of the First German Case of a Contamination with Perfluorinated Compounds (PFC) in the Region Sauerland, North Rhine-Westphalia, J. Toxicol. Environ. Health, Part A, 2008, 71, 725–733, DOI:10.1080/15287390801985216.
- L. J. Winchell, M. J. M. Wells, J. J. Ross, X. Fonoll, J. W. Norton, S. Kuplicki, M. Khan and K. Y. Bell, Per- and Polyfluoroalkyl Substances Presence, Pathways, and Cycling through Drinking Water and Wastewater Treatment, J. Environ. Eng., 2022, 148, 03121003, DOI:10.1002/wer.1483.
- H. Sun, A. C. Gerecke, W. Giger and A. C. Alder, Long-chain perfluorinated chemicals in digested sewage sludges in Switzerland, Environ. Pollut., 2011, 159, 654–662, DOI:10.1016/j.envpol.2010.09.020.
- C. Gómez-Canela, J. A. C. Barth and S. Lacorte, Occurrence and fate of perfluorinated compounds in sewage sludge from Spain and Germany, Environ. Sci. Pollut. Res., 2012, 19, 4109–4119, DOI:10.1007/s11356-012-1078-7.
- K. A. Thompson, S. Mortazavian, D. J. Gonzalez, C. Bott, J. Hooper, C. E. Schaefer and E. R. V. Dickenson, Poly- and Perfluoroalkyl Substances in Municipal Wastewater Treatment Plants in the United States: Seasonal Patterns and Meta-Analysis of Long-Term Trends and Average Concentrations, ACS ES&T Water, 2022, 2, 690–700, DOI:10.1021/acsestwater.1c00377.
- H. Mu, J. Li, L. Chen, H. Hu, J. Wang, C. Gu, X.-x. Zhang, H.-q. Ren and B. Wu, Distribution, source and ecological risk of per- and polyfluoroalkyl substances in Chinese municipal wastewater treatment plants, Environ. Int., 2022, 167, 107447, DOI:10.1016/j.envint.2022.107447.
- J. Kim, X. Xin, B. T. Mamo, G. L. Hawkins, K. Li, Y. Chen, Q. Huang and C.-H. Huang, Occurrence and Fate of Ultrashort-Chain and Other Per- and Polyfluoroalkyl Substances (PFAS) in Wastewater Treatment Plants, ACS ES&T Water, 2022, 2, 1380–1390, DOI:10.1021/acsestwater.2c00135.
- M. C. S. Costello and L. S. Lee, Sources, Fate, and Plant Uptake in Agricultural Systems of Per- and Polyfluoroalkyl Substances, Curr. Pollut. Rep., 2020 DOI:10.1007/s40726-020-00168-y.
- R. K. Lazcano, Y. J. Choi, M. L. Mashtare and L. S. Lee, Characterizing and Comparing Per- and Polyfluoroalkyl Substances in Commercially Available Biosolid and Organic Non-Biosolid-Based Products, Environ. Sci. Technol., 2020, 54(14), 8640–8648, DOI:10.1021/acs.est.9b07281.
- N. Bolan, B. Sarkar, M. Vithanage, G. Singh, D. C. W. Tsang, R. Mukhopadhyay, K. Ramadass, A. Vinu, Y. Sun, S. Ramanayaka, S. A. Hoang, Y. Yan, Y. Li, J. Rinklebe, H. Li and M. B. Kirkham, Distribution, behaviour, bioavailability and remediation of poly- and per-fluoroalkyl substances (PFAS) in solid biowastes and biowaste-treated soil, Environ. Int., 2021, 155, 106600, DOI:10.1016/j.envint.2021.106600.
- G. Munoz, A. M. Michaud, M. Liu, S. Vo Duy, D. Montenach, C. Resseguier, F. Watteau, V. Sappin-Didier, F. Feder, T. Morvan, S. Houot, M. Desrosiers, J. Liu and S. Sauvé, Target and Nontarget Screening of PFAS in Biosolids, Composts, and Other Organic Waste Products for Land Application in France, Environ. Sci. Technol., 2022, 56(10), 6056–6068, DOI:10.1021/acs.est.1c03697.
- P. Roesch, C. Vogel, T. Huthwelker, P. Wittwer and F.-G. Simon, Investigation of per- and polyfluoroalkyl substances (PFAS) in soils and sewage sludges by fluorine K-edge XANES spectroscopy and combustion ion chromatography, Environ. Sci. Pollut. Res., 2022, 29, 26889–26899, DOI:10.1007/s11356-021-17838-z.
- C. E. Schaefer, J. Hooper, M. Modiri-Gharehveran, D. M. Drennan, N. Beecher and L. Lee, Release of poly- and perfluoroalkyl substances from finished biosolids in soil mesocosms, Water Res., 2022, 217, 118405, DOI:10.1016/j.watres.2022.118405.
- J. Caniglia, D. D. Snow, T. Messer and S. Bartelt-Hunt, Extraction, analysis, and occurrence of per- and polyfluoroalkyl substances (PFAS) in wastewater and after municipal biosolids land application to determine agricultural loading, Front. Water, 2022, 4, 892451, DOI:10.3389/frwa.2022.892451.
-
German Sewage Sludge Ordinance, Verordnung zur Neuordnung der Klärschlammverwertung, Bundesgesetzblatt, Bonn, 2017 Search PubMed.
-
German Fertilizer Ordinance, Verordnung über das Inverkehrbringen von Düngemitteln, Bodenhilfsstoffen, Kultursubstraten und Pflanzenhilfsmitteln (Düngemittelverordnung – DüMV), Bundesgesetzblatt, Bonn, 2012 Search PubMed.
- S. Kratz, C. Vogel and C. Adam, Agronomic performance of P recycling fertilizers and methods to predict it: a review, Nutrient Cycl. Agroecosyst., 2019, 115, 1–39, DOI:10.1007/s10705-019-10010-7.
-
C. Schaum, Phosphorus: Polluter and Resource of the Future, IWA Publishing, 2018 Search PubMed.
- G. K. Longendyke, S. Katel and Y. Wang, PFAS fate and destruction mechanisms during thermal treatment: a comprehensive review, Environ. Sci.: Processes Impacts, 2022, 24, 196–208, 10.1039/D1EM00465D.
- H. Bamdad, S. Papari, E. Moreside and F. Berruti, High-Temperature Pyrolysis for Elimination of Per- and Polyfluoroalkyl Substances (PFAS) from Biosolids, Processes, 2022, 10, 2187, DOI:10.3390/pr10112187.
- L. J. Winchell, J. J. Ross, M. J. M. Wells, X. Fonoll, J. W. Norton Jr and K. Y. Bell, Per- and polyfluoroalkyl substances thermal destruction at water resource recovery facilities: a state of the science review, Water Environ. Res., 2020, 93, 826–843, DOI:10.1002/wer.1483.
- J. Li, J. He, Z. Niu and Y. Zhang, Legacy per- and polyfluoroalkyl substances (PFASs) and alternatives (short-chain analogues, F-53B, GenX and FC-98) in residential soils of China: present implications of replacing legacy PFASs, Environ. Int., 2020, 135, 105419, DOI:10.1016/j.envint.2019.105419.
- Y. Ogawa, E. Tokunaga, O. Kobayashi, K. Hirai and N. Shibata, Current Contributions of Organofluorine Compounds to the Agrochemical Industry, iScience, 2020, 23, 101467, DOI:10.1016/j.isci.2020.101467.
- J. Wang, M. Sánchez-Roselló, J. L. Aceña, C. del Pozo, A. E. Sorochinsky, S. Fustero, V. A. Soloshonok and H. Liu, Fluorine in Pharmaceutical Industry: Fluorine-Containing Drugs Introduced to the Market in the Last Decade (2001–2011), Chem. Rev., 2014, 114, 2432–2506, DOI:10.1021/cr4002879.
- E. Hammel, T. F. Webster, R. Gurney and W. Heiger-Bernays, Implications of PFAS definitions using fluorinated pharmaceuticals, iScience, 2022, 25, 104020, DOI:10.1016/j.isci.2022.104020.
-
UBA, Reducing the Input of Chemicals into Waters: Trifluoroacetate (TFA) as a Persistent and Mobile Substance with Many Sources, 2021 Search PubMed.
-
EU, Poly- and Perfluoroalkyl Substances (PFAS) – Commission Staff Working Document, 2020 Search PubMed.
- L. Gehrenkemper, F. Simon, P. Roesch, E. Fischer, M. von der Au, J. Pfeifer, A. Cossmer, P. Wittwer, C. Vogel, F.-G. Simon and B. Meermann, Determination of organically bound fluorine sum parameters in river water samples—comparison of combustion ion chromatography (CIC) and high resolution-continuum source-graphite furnace molecular absorption spectrometry (HR-CS-GFMAS), Anal. Bioanal. Chem., 2021, 413, 103–115, DOI:10.1007/s00216-020-03010-y.
- Z. Fang, Y. Li, Y. Li, D. Yang, H. Zhang, K. C. Jones, C. Gu and J. Luo, Development and Applications of Novel DGT Passive Samplers for Measuring 12 Per- and Polyfluoroalkyl Substances in Natural Waters and Wastewaters, Environ. Sci. Technol., 2021, 55, 9548–9556, DOI:10.1021/acs.est.0c08092.
- C. Vogel, R. Sekine, D. Steckenmesser, E. Lombi, D. Steffens and C. Adam, Phosphorus availability of sewage sludge-based fertilizers determined by the diffusive gradients in thin films (DGT) technique, J. Plant Nutr. Soil Sci., 2017, 180, 594–601, DOI:10.1002/jpln.201600531.
- D. Steckenmesser, C. Vogel, C. Adam and D. Steffens, Effect of various types of thermochemical processing of sewage sludges on phosphorus speciation, solubility, and fertilization performance, Waste Manage., 2017, 62, 194–203, DOI:10.1016/j.wasman.2017.02.019.
-
Deutsches Institut für Normung, DIN 38414-14 – German Standard Methods for the Examination of Water, Waste Water and Sludge – Sludge and Sediments (Group S) – Part 14: Determination of Selected Polyfluorinated Compounds (PFC) in Sludge, Compost and Soil – Method Using High Performance Liquid Chromatography and Mass Spectrometric Detection, (HPLC-MS/MS), 2011 Search PubMed.
- C. Vogel, O. Krüger, H. Herzel, L. Amidani and C. Adam, Chemical state of mercury and selenium in sewage sludge ash based P-fertilizers, J. Hazard. Mater., 2016, 313, 179–184, DOI:10.1016/j.jhazmat.2016.03.079.
- R. A. Dickman and D. S. Aga, Efficient workflow for suspect screening analysis to characterize novel and legacy per- and polyfluoroalkyl substances (PFAS) in biosolids, Anal. Bioanal. Chem., 2022, 414, 4497–4507, DOI:10.1007/s00216-022-04088-2.
- K. M. Spaan, F. Seilitz, M. M. Plassmann, C. A. de Wit and J. P. Benskin, Pharmaceuticals Account for a Significant Proportion of the Extractable Organic Fluorine in Municipal Wastewater Treatment Plant Sludge, Environ. Sci. Technol. Lett., 2023, 10, 328–336, DOI:10.1021/acs.estlett.3c00108.
- Y.-R. Huang, S.-S. Liu, J.-X. Zi, S.-M. Cheng, J. Li, G.-G. Ying and C.-E. Chen, In Situ Insight into the Availability and Desorption Kinetics of Per- and Polyfluoroalkyl Substances in Soils with Diffusive Gradients in Thin Films, Environ. Sci. Technol., 2023, 57, 7809–7817, DOI:10.1021/acs.est.2c09348.
- C. Vogel, R. Sekine, D. Steckenmesser, E. Lombi, H. Herzel, L. Zuin, D. Wang, R. Felix and C. Adam, Combining diffusive gradients in thin films (DGT) and spectroscopic techniques for the determination of phosphorus species in soils, Anal. Chim. Acta, 2019, 1057, 80–87, DOI:10.1016/j.aca.2019.01.037.
- C. Vogel, M. C. Hoffmann, O. Krüger, V. Murzin, W. Caliebe and C. Adam, Chromium (VI) in phosphorus fertilizers determined with the diffusive gradients in thin-films (DGT) technique, Environ. Sci. Pollut. Res., 2020, 27, 24320–24328, DOI:10.1007/s11356-020-08761-w.
- X. Gao and J. Chorover, Adsorption of perfluorooctanoic acid and perfluorooctanesulfonic acid to iron oxide surfaces as studied by flow-through ATR-FTIR spectroscopy, Environ. Chem., 2012, 9, 148–157, DOI:10.1071/EN11119.
- T. Yan, H. Chen, F. Jiang and X. Wang, Adsorption of Perfluorooctane Sulfonate and Perfluorooctanoic Acid on Magnetic Mesoporous Carbon Nitride, J. Chem. Eng. Data, 2014, 59, 508–515, DOI:10.1021/je400974z.
- E. L. Varetti, The infrared spectra of trifluoromethanesulphonic acid in different states of aggregation, Spectrochim. Acta, Part A, 1988, 44, 733–738, DOI:10.1016/0584-8539(88)80135-1.
- M. I. Delgado-Rosero, N. M. Jurado-Meneses and M. Á. Meléndez-Lira, Structural and vibrational studies on composites polymer electrolytes (PEO)10CF3COONa + x wt.% Al2O3, Rev. Fac. Ing., Univ. Antioquia, 2017, 43–49, DOI:10.17533/udea.redin.n83a06.
- K. A. Kristoffersen, A. van Amerongen, U. Böcker, D. Lindberg, S. G. Wubshet, H. de Vogel-van den Bosch, S. J. Horn and N. K. Afseth, Fourier-transform infrared spectroscopy for monitoring proteolytic reactions using dry-films treated with trifluoroacetic acid, Sci. Rep., 2020, 10, 7844, DOI:10.1038/s41598-020-64583-3.
- B. Yan, J. Wang and J. Liu, STXM-XANES and computational investigations of adsorption of per- and polyfluoroalkyl substances on modified clay, Water Res., 2021, 201, 117371, DOI:10.1016/j.watres.2021.117371.
- B. Göckener, F. T. Lange, L. Lesmeister, E. Gökçe, H. U. Dahme, N. Bandow and A. Biegel-Engler, Digging deep—implementation, standardisation and interpretation of a total oxidisable precursor (TOP) assay within the regulatory context of per- and polyfluoroalkyl substances (PFASs) in soil, Environ. Sci. Eur., 2022, 34, 52, DOI:10.1186/s12302-022-00631-1.
-
EU, Directive (EU) 2020/2184 of the European Parliament and of the Council of 16 December 2020 on the Quality of Water Intended for Human Consumption, 2020 Search PubMed.
- A. Garg, N. P. Shetti, S. Basu, M. N. Nadagouda and T. M. Aminabhavi, Treatment technologies for removal of per- and polyfluoroalkyl substances (PFAS) in biosolids, Chem. Eng. J., 2023, 453, 139964, DOI:10.1016/j.cej.2022.139964.
- E. D. Thoma, R. S. Wright, I. George, M. Krause, D. Presezzi, V. Villa, W. Preston, P. Deshmukh, P. Kauppi and P. G. Zemek, Pyrolysis Processing of PFAS-Impacted Biosolids, a Pilot Study, J. Air Waste Manage. Assoc., 2021, 72, 309–318, DOI:10.1080/10962247.2021.2009935.
- W. Buss, Pyrolysis Solves the Issue of Organic Contaminants in Sewage Sludge while Retaining Carbon—Making the Case for Sewage Sludge Treatment via Pyrolysis, ACS Sustain. Chem. Eng., 2021, 9, 10048–10053, DOI:10.1021/acssuschemeng.1c03651.
- B. A. Seay, K. Dasu, I. C. MacGregor, M. P. Austin, R. T. Krile, A. J. Frank, G. A. Fenton, D. R. Heiss, R. J. Williamson and S. Buehler, Per- and polyfluoroalkyl substances fate and transport at a wastewater treatment plant with a collocated sewage sludge incinerator, Sci. Total Environ., 2023, 874, 162357, DOI:10.1016/j.scitotenv.2023.162357.
- S. Björklund, E. Weidemann and S. Jansson, Emission of Per- and Polyfluoroalkyl Substances from a Waste-to-Energy Plant-Occurrence in Ashes, Treated Process Water, and First Observation in Flue Gas, Environ. Sci. Technol., 2023, 57, 10089–10095, DOI:10.1021/acs.est.2c08960.
- E. P. Shields, J. D. Krug, W. R. Roberson, S. R. Jackson, M. G. Smeltz, M. R. Allen, R. P. Burnette, J. T. Nash, L. Virtaranta, W. Preston, H. K. Liberatore, M. A. G. Wallace, J. V. Ryan, P. H. Kariher, P. M. Lemieux and W. P. Linak, Pilot-Scale Thermal Destruction of Per- and Polyfluoroalkyl Substances in a Legacy Aqueous Film Forming Foam, ACS ES&T Engg, 2023, 3(9), 1308–1317, DOI:10.1021/acsestengg.3c00098.
|
This journal is © The Royal Society of Chemistry 2023 |
Click here to see how this site uses Cookies. View our privacy policy here.