DOI:
10.1039/D3AN01869E
(Paper)
Analyst, 2024,
149, 100-107
Robust and rapid partitioning in thermoplastic†
Received
27th October 2023
, Accepted 10th November 2023
First published on 14th November 2023
Abstract
Partitioning is the core technology supporting digital assays. It divides a sample into thousands of individual reactors prior to amplification and absolute quantification of target molecules. Thermoplastics are attractive materials for large scale manufacturing, however they have been seldomly used for fabricating partitioning arrays. Patitioning in thermoplastic devices has proven difficult due to the challenge of efficiently displacing the air trapped in the nanoliter structures during priming of thousands of chambers. Here, we report the design of an array of chambers made of thermoplastics where the progression of the liquid–air interface is controlled by capillary effects. Our device performs robust partitioning over a wide range of pressures and can be actuated at low pressure by a simple micropipette. Our thermoplastic device lays the foundation to cost-effective and instrument-free partitioning platforms, which could be deployed in low-resource settings.
Introduction
Digital PCR (dPCR) has rapidly emerged as an alternative method to quantitative PCR due to its absolute quantification capabilities and higher tolerance to biological and sample preparation inhibitors. dPCR relies on partitioning a sample into thousands of independent PCR reactions before amplification and enumeration of the nucleic acid target molecules. The technology has greatly benefited from advances in microfluidic technologies.1 Typical microfluidic methods for generating partitions include droplet microfluidics,2–6 array of microwells,7,8 and array of chambers.9–12
Droplet microfluidics have led the widespread adoption of dPCR but require specialized and expensive equipment. Array of microwells, and array of chambers represent promising alternatives for developing low-cost and equipment-free partitioning platforms. However, the challenge of these formats is to prime thousands of nanoliter chambers or microwells by displacing the air present in the microstructures. To address this challenge, these technologies typically exploit the gas permeability of PDMS (polydimethylsiloxane).8,11,12 The air trapped in the nanoliter structures is evacuated through the PDMS substrate by passive diffusion during filling at low pressure. Still, PDMS manufacturing is laborious and costly, which hampers the widespread implementation of those devices.
Thermoplastics are the materials of choice when scaling up production thanks to its low-cost manufacturing techniques such as embossing and injection-molding. However, thermoplastics are not gas permeable at low injection pressure, and thus there exists only a few examples of thermoplastic partitioning platforms.
Passive diffusion can still be achieved through a thin layer of cyclo olefin polymer (COP), but it requires a pressure as high as 50 psi.13 This high pressure is at the margin of typical bonding strength of thermoplastic microfluidic devices. Hence, it complexifies the manufacturing process and requires specialized equipment to operate the array of chambers.
Alternatively, a floating pressure sensitive adhesive is used to create partitions when pressed against an array of micro-wells.14 This approach also requires specialized equipment to provide reliable partitioning.
Lastly, a couple of schemes controls the progression of the liquid–air interface to ensure proper filling by displacing the air trapped in microwells or chambers. For arrays of microwells, asymmetric contact angles between the top of the array and the microwell walls promote efficient filling.15 The contact angle asymmetry is created by a differential surface treatment, which significantly complexifies the manufacturing process of the device. For arrays of chambers, the liquid–air interface can be directed through a clever design of staggered chambers that harnesses pinning.16 The cyclo olefin copolymer (COC) device is sealed using solvent-assisted bonding. Despite its attractiveness, its design principles limit the chamber geometry. The proof of principle exhibited a 12% standard deviation of the partition volume for a limited number of 6 independent channels containing 168 chambers each.
Here, we report the original design of a thermoplastic array of nanoliter chambers to perform partitioning at low pressure. The progression of the liquid–air interface is controlled by capillary effects while chambers integrate an escape route for the air. The device is made of native COC and sealed with a pressure sensitive tape. Its manufacturing is simple, rapid, and cost-effective. We develop an array of 3200 chambers and characterize its filling and partition efficiencies. We also show that a simple micropipette can be used to perform partitioning efficiently at low pressure. Our device allows for the rapid and efficient creation of uniform partitions without specialized equipment. Our platform will prove critical in providing low-resource settings with access to cutting-edge digital assays.
Materials and methods
Materials
The cyclic olefin copolymer (COC) thermoplastic (Topas 5013L-10) was purchased from Knightsbridge Plastics (Fremont, CA, USA); the pressure-sensitive adhesive (PSA) film (ThermalSeal RTS, Excel Scientific) from Fisher Scientific (Boston, USA), the PDMS (Sylgard 184, Dow Chemicals) from Krayden (Westminister, CO, USA), the 3′′ P(100) test grade silicon wafer from University Wafer (South Boston, MA, USA), the SU8 2025 photoresist from Kayaku (Woburn, MA, USA), the fluorinated silane 1H,1H,2H,2H-perfluorooctyltrichlorosilane from Gelest (Morrisville, PA, USA), the high density foam (1059N372, medium hardness, 16 psi to compress 25%) from Mc Master-Carr (Elmhurst, IL, USA), the ring magnets (R844-N52) from K&J Magnetics (Pipersville, PA, USA). The Taq buffer (#B9014S) was purchased from New England Biolabs (Ipswich, MA, USA) and the Tetronic 1307 was a gift from BASF. The FC-3283 Fluorinert oil was purchased from 3M (Pittsburgh, PA, USA), and the PFPE-PEG-PFPE surfactant17 was synthesized in house.
The ultra-portable bench press was purchased from FVRosintech (Richmond, BC, Canada) and the oxygen plasma oven model PDC-32G was purchased from Harrick Plasma (Ithaca, NY, USA). An UV mask aligner (500 W UV illuminator, Newport, Irvine, CA) was used to expose the photoresist. Pressure controllers are 30 psi regulators from Triline automation (Rochester, NY, USA) controlled with a custom LabView software (National Instruments, Austin, Texas, USA) via an Arduino Uno (Arduino, Italy) interface. The 1000 μL pipette (#89080-000) was purchased from VWR International (Bridgeport, NJ, USA). 1/32′′ PEEK tubing was custom ordered from Zeus Industrial Products (Orangeburg, SC, USA).
To acquire images of the progressing interface, an inverted brightfield microscope was used (Diaphot-TMD, Nikon, Tokyo, Japan) equipped with a camera (XCD-V60, Sony, Tokyo, Japan) and controlled with a custom Labview software. Large scale images were acquired with an automated Nikon AZ100 monozoom microscope equipped with a controllable stage (Prior Scientific, MA, USA), a DS-Qi2 camera (Nikon), an AZ-Plan Fluor 5× lens (NA = 0.5), and an Aura-2 LED light engine (Lumencor, OR, USA). The system was controlled by the Nikon NIS-Elements software.
Videos were acquired with a cell phone (moto g power 2022, Motorola) by placing the device on top of 3.75′′ square LED backlight (LED-SP, Amscope, Irvine, CA, USA).
Methods
Device microfabrication
Device microfabrication was adapted from ref. 18. The circuit was designed using AutoCAD (AutoDESK, San Rafael, CA, USA) and was printed at high resolution (25
400 dpi) on a Mylar mask (Artnet Pro, San Jose, CA, USA). The design pattern was transferred onto a 3′′ silicon wafer using the negative photoresist SU8 2025 to create a first 40 μm deep layer. The branched inlet and outlet were patterned onto a second 80 μm deep layer using an established protocol.19 The mold was treated with a vapor of fluorinated silane overnight in a vacuum chamber. A 10
:
1 (w/w) PDMS secondary mold was created by casting the silicon SU8 hybrid mold. The PDMS mold was then treated with a vapor of 1H,1H,2H,2H-perfluorooctyltrichlorosilane overnight in a vacuum chamber after oxygen plasma activation. The PDMS mold served to create the 5
:
1 (w/w) PDMS die by casting. After curing, the 5
:
1 PDMS die was treated with a vapor of 1H,1H,2H,2H-perfluorooctyltrichlorosilane overnight in a vacuum chamber after oxygen plasma activation.
COC imprinting
The COC plate was cut to dimension with a micro bandsaw (MBS 240/E, Proxxon), deburred with a razor blade and cleaned with isopropanol. The COC plate was then assembled with the 5
:
1 PDMS die flanked by two glass slides and aluminium plates and pressed by four office binder clips. The sandwich was incubated in a conventional oven at 160 °C overnight. The sandwich was then left to cool at room temperature before disassembly. Ports were drilled with a mini drill press (TBM 220, Proxxon) and the device was thoroughly cleaned with isopropanol and dried. The PSA was applied onto the device with a bench press using a medium hardness density foam to ensure homogenous and repeatable sealing. The pressure was evaluated by monitoring the foam deformation using a ruler affixed to the bench press, it was estimated to be 2.2 psi.
Metrology
The channel depth of the embossed COC device was measured by imaging the channel cross-section after scoring and breaking the device. We used a 40× objective to collect images, an optical calibration target, and Fiji image processing software to extract the channel depths.
Magnetic connectors
To create magnetic connectors, magnetic rings were over-filled with 10
:
1 PDMS, and a central hole was punched after curing. The tubing was inserted into the hole, and the connector was clamped onto the COC device using a second magnetic ring on the opposite side of the device. The PDMS dome created by the overfill ensures sealing with the COC device upon applying a magnetic force. The punched PDMS ensures the tubing sealing similar to regular PDMS devices. This magnetic connector can consistently sustain injection pressures upward of 35 psi (manuscript in preparation).
Solutions and injection
A solution of 1× Taq buffer 1% weight Tetronic 1307 containing a food dye to increase image contrast was injected. The immiscible oil phase consisted of FC-3283 with 2 wt% of a PFPE-PEG-PFPE surfactant (0.6 wt% critical micellar concentration).17 Solutions were injected through a 1/32′′ PEEK tubing with a 30 psi pressure regulator or with a 1000 μL micropipette.
Images of the interface progression were collected with the inverted brightfield microscope. To characterize the filling and partitioning steps, images of the entire device were acquired using the automated monozoom and white light. Large images were stitched from 234 (13 × 18) individual images.
Image analysis
Filling success rate was determined by scanning the arrays and counting the misfilled chambers. Droplet volume was extracted from individual images. A macro under Fiji (https://fiji.sc/)20 was developed to extract the partition areas. The device thickness was then measured by cutting a device and measuring the channel height with the brightfield inverted microscope and calibration target. Due to sampling, volume distributions were compiled from about 2000 droplets (1960 < n < 2100). Graphs were produced within the python Jupyter notebook using the Matplotlib, Panda and Seaborn packages.
Results
Providing an escape route for air and controlling the air–liquid interface with capillary valving
The challenge of filling micron-size chambers is due to the presence of trapped air that must be displaced. In thermoplastics, air cannot be degased through the material when filling at low pressure. To address this conundrum, our design controls the water–air interface to fill chambers while providing an escape route for the air. It relies on capillary valving that precludes an interface from entering the channel of smaller dimension at a bifurcation.
The main channel (or feeding channel) feeds into chambers with a wider opening, effectively diverting the liquid–air interface into the chamber at each junction. The water–air interface progresses into a chamber until it reaches capillary channels that allow for the air to escape (Fig. 1A). At this point, the interface progresses again through the feeding channel to the next chamber (Fig. 1B). Capillary valving relies on interfacial effects and requires operation at low capillary number (Ca). Partitioning can be completed by injecting an immiscible oil from the outlet thanks again to capillary valving (Fig. 1C).
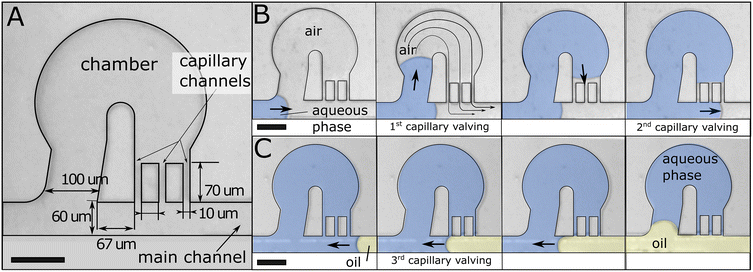 |
| Fig. 1 Design principles. (A) Partitioning unit. (B) Filling based on capillary valving. (C) Partitioning using capillary valving at low Ca: immiscible oil is injected from the outlet. The different phases are colored for clarity (blue: aqueous solution, yellow: immiscible fluorinated oil). Scale bars represent 100 μm. | |
Straightforward fabrication
We identified a combination of cyclic olefin copolymer (COC) thermoplastic to produce the fluidic part and a pressure-sensitive adhesive (PSA) film to provide sealing. COC and PSA have similar hydrophobic surface properties (see advancing angles in Table 1). We used a PCR solution consisting of 1× final Taq buffer containing a food dye and Tetronic 1307 at 1% weight. The addition of Tetronic 1307 to the PCR mix has been shown to improve droplet stability during thermocycling.21 Using COC and PSA allows for rapid and cost-effective fabrication. The COC to PSA bonding has been shown to sustain up to 72 psi.22 We adapted a published protocol18 that assures simple fabrication of COC devices by thermal imprinting (Fig. 2). The COC plate is sandwiched with a 5
:
1 (wt) PDMS die, and the assemblage is incubated at 160 °C overnight in a conventional oven. We measured the channel depth along four different lines at a regular distance (4, 13, 22 and 31 mm) from the inlet. We collected 12 measurements across each line. The average depth across the lines was 40.8, 40.6, 40.5, and 40.8 μm, with a standard deviation of 1.1, 1.3, 1.5, and 1.3%. The standard deviation between the line averages was 0.3%. These measurements prove that the fabrication method provides consistent geometry across the array.
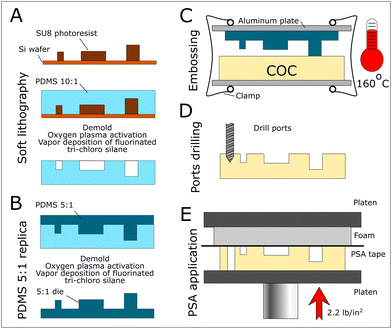 |
| Fig. 2 Fabrication by thermal embossing. (A) Typical soft lithography is used to create a 10 : 1 PDMS imprint from a silicon-SU8 hybrid master. (B) A 5 : 1 PDMS die is molded from the 10 : 1 PDMS negative. (C) The die and the COC plate are sandwiched together with binder clips. Embossing is performed overnight at 160 °C in an oven. (D) Ports are drilled. (E) PSA tape is applied with a bench press at room temperature. | |
Table 1 Contact angles of water and PCR solution on COC and PSA tape
|
Resting angle |
Advancing angle |
Receding angle |
Water on COC |
87.2° ± 2.0 |
87.6° ± 2.2 |
74.8° ± 2.9 |
Water on PSA |
93.3° ± 2.6 |
101.3° ± 2.3 |
71.2° ± 0.8 |
PCR solution on COC |
66.3° ± 1.8 |
85.8° ± 1.8 |
23.2° ± 2.6 |
PCR solution on PSA |
76.8° ± 1.1 |
91.6° ± 2.2 |
52.3° ± 3.1 |
After drilling ports, we apply the PSA tape with a benchtop hydraulic press to ensure a reliable and homogeneous sealing without channel obstruction. Importantly, all materials are compatible with molecular reactions.22 We injected fluids into devices using novel magnetic connectors (manuscript in preparation). They ensure rapid, re-usable and cost-effective interfacing with thermoplastic devices. Finally, using a channel and a single port, we confirmed that the device was airtight as we could not fill the channel even at pressures up to 30 psi. The COC and PSA surfaces are hydrophobic (Table 1) and are not modified during the device manufacturing process. Our design is capillary controlled, and in contrast to capillary driven approaches, pressure is requied to inject liquids.
Improving robustness by design
We designed 32 parallel branches fed by the same inlet via successively splitting channels to create a 3200-partition array (ESI† – CAD file). The array is 40 μm deep and the feeding channels are 80 μm deep. We modified the initial chamber design to improve its robustness for device microfabrication and working performance. Embossing thermoplastic with a PDMS die imposes to minimize the structures aspect ratio to limit channel deformation. We swapped the channel width and height of the feeding channels, which still maintained their capacity at guiding interfaces due to capillary effects. We used a single capillary channel and widened it to 15 μm to minimize distortion during embossing.
For functional aspects, we optimized the width of the feeding channel using the filling rate of the array as the figure-of-merit. We considered the relative Laplace pressure, a quasi-static situation true at low Ca, for the first two capillary valving steps. For that purpose, we fixed the width of the chamber opening and capillary channel to 100 μm and 15 μm, respectively (ESI Fig. S1†). The analysis shows that the differential Laplace pressure for the first two capillary valving steps is similar for a 25 μm wide feeding channel. We added 8 μm long and 25 μm wide capillary constrictions to better control the relative progression of the interface at branches. A typical failure mode of the initial design was the progression of the interface through one of the capillary channels. To lower the likelihood of this failure mode we used a single capillary channel in the design (Fig. 3).
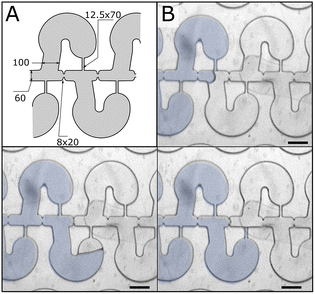 |
| Fig. 3 Microfluidic chambers in series. (A) Design details. (B) Progression of the liquid–air interface through the structure. Scale bars represent 100 μm. | |
Upon closer inspection, we noted several failure modes due to uncontrolled flow: (1) the triple line can detach from the external wall of the chamber (Fig. 4A); and (2) uncontrolled unpinning of the interface can occur at the extremity of the capillary constrictions (Fig. 4B) and capillary channels (Fig. 4C, see ESI Fig. S2† for complete sequences). Fig. 4D highlights the problematic structures of the design. To remediate these issues, we added a progressive opening and curvature to the entrance to improve chamber filling, and curvatures to the capillary channel extremity to avoid pinning (Fig. 4E).
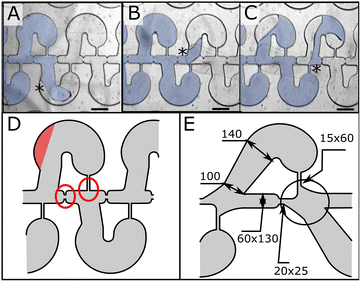 |
| Fig. 4 Failure modes. (A) The triple line does not always follow the external wall of the chambers. (B) and (C) We noticed uncontrolled unpinning at the capillary constrictions (B) and channels (C). Stars point to the design structure associated with the specific failure mode. (D) The design areas that need improvement are highlighted in red. (E) New design to address the failure modes. Scale bars represent 100 μm. | |
Array filling is robust across a wide range of injection pressures
We characterized the filling step by injecting the PCR solution at different pressures (Fig. 5). We tested several COC embossed devices to perform the experiments. We inspected the arrays after filling, counted the number of chambers not properly filled, and reported the success rate as a function of the injection pressure (see an example of a miss-filled chamber in ESI Fig. S3†). At 3 psi, the filling rate is at around 90%, while the filling rate is over 95% at 6 psi and close to 100% in the 12–24 psi range. We did not see any correlation between the different embossing replicates and filling rates, or failures and location across the array.
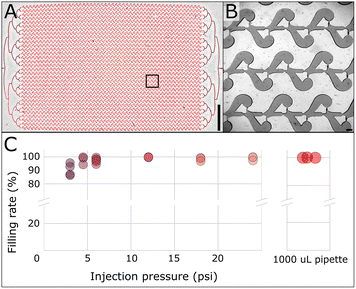 |
| Fig. 5 Robust array filling across a wide pressure range. (A) Overall array after the filling step. The scale bar represents 5 mm. (B) Close-up of panel A depicts chambers filling. The scale bar represents 100 μm. (C) Success rate at different filling pressures and using a 1000 μL pipette (right panel). | |
Interestingly, the array filling performed consistently at a very high success rate over 99.8% (fewer than 7 chambers mis-filled) using a 1000 μL standard pipette to inject the PCR solution (Fig. 5C – right panel). The filling operation takes about 15 seconds with the micropipette (ESI Movie M1†). Using air compressibility and the array filling time, we estimated the delivery pressure of the pipette to be around 4.5 psi. Intriguingly, the pipette performed better in filling the array than the pressure controllers in that pressure range.
Array partitioning is robust with a simple micropipette
We characterized the partitioning step by injecting a solution of FC-3283 fluorinated oil (3 M) containing 2 wt% PEG-PFPE-PEG surfactant17,23 using a 1000 μL pipette. We noticed that the presence of surfactant is critical to avoid PSA delamination. We collected images across the array and extracted the droplet volume to report its distribution across five experimental replicates using the same COC embossed device. The partitioning operation takes about 15 seconds (Fig. 6A and ESI Movie M2†). First, the partitioning step proceeded with a very low number of failures, which are characterized by a bypass of the oil through the capillary channel and the chamber and leaves a smaller partition in the feeding channel (left insert Fig. 6B). Second, the partition volume exhibited a normal and tight distribution with a standard deviation ranging from 2.4 to 3% (right insert Fig. 6B). Third, the 5 experimental results exhibited all a very robust and highly repeatable partitioning with an average volume of 2.9 nL (overall range of 2.89 to 2.91 nL).
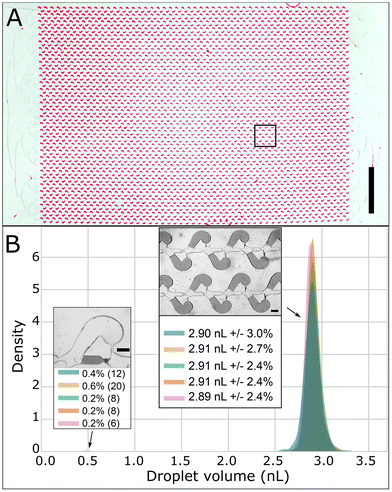 |
| Fig. 6 Array partitioning using a 1000 μL pipette. (A) Overall array after partitioning. The scale bar represents 5 mm. (B) Volume distribution of the partitions for 5 experimental repeats. Left inset depicts a bypass of partitioning failures with the respective percentages. Right inset depicts successful partitioning with the associated statistical parameters of the corresponding distribution peaks. The scale bars represent 100 μm. | |
We also performed partitioning on three different imprinted COC devices. In this case, we obtained an average volume from 2.75 to 2.9 nL with a standard deviation ranging from 2.8 to about 5% (ESI Fig. S4†).
Discussion
Filling nanoliter chambers has been a long-standing challenge of microfluidics that has been occulted by the use of the gas permeable PDMS as a material of choice. In this report, we present a novel design that allows capillary-controlled reliable filling of nanoliter chambers in a thermoplastic device. Interestingly, capillary-controlled flow has been used to control the filling of large chambers using rails or phase guides24 but not for an array of nanoliter chambers. The principle of our design is to provide an escape route for the air trapped in nanoliter chambers during filling. The progression of the liquid–air interface is controlled between the chamber and the feeding channel. Interestingly, we did not observe any air trapped in the capillary channel after partitioning, which could complicate thermocycling.
The filling step of the device performs remarkably well across a wide range of injection pressures, even though higher velocities at higher injection pressures should challenge the basic principles of our design. To evaluate at which pressure our design principle would break down, let's consider the capillary number Ca:
where
μ is the dynamic viscosity,
σ the interfacial energy, and
v the typical velocity. We previously observed that capillary effects tend to be overcome by viscous effects above a
Ca of 1.5 × 10
−6.
25 Using the properties for water and air (
m = 10
−3 Pa s and
s = 72 × 10
−3 N m
−1), we can deduct that the velocity in our device should reach 108 mm s
−1 to operate at this
Ca value. This velocity is not attainable at a reasonable pressure in our design. Our device is 36.5 mm long and it would take 0.3 seconds to fill it at such
Ca. As a comparison, it takes 4 s to fill our device at 24 psi, which means that we would need to use a pressure of up to 250 psi to fill the device in 0.3 s. The flow is controlled by the aqueous phase-air interface. The high interfacial energy compounds with the low viscosity of the liquid phase, which maintains a low capillary number across a wide range of velocities and pressures.
Intriguingly, a simple pipette yields better and more consistent results than pressure controllers. We noticed that managing air in the injected liquid is easier with the pipette, especially for injecting oil due to a more direct visual inspection of the tip compared to opaque tubing. Also, we expect pressure controllers to create small flow instability upon actuation. Importantly, the possibility of using a simple pipette opens the possibility of instrument-free digital assays.
The partitioning success rate and volume variation measured for at least one COC imprint is comparable to microfluidic droplets that typically exhibit 1% in droplet diameter, hence 3% variation in volume. Our device performs as well as the QIacuity digital PCR system from Qiagen that reports a success rate of 96.5% for 8500 wells14 although for a higher number of smaller chambers. The partitioning for the same COC imprint is remarkably repeatable. We also noticed a degree of variability between different imprints using the same die. This underlines inconsistencies in our current manufacturing process. PDMS compliance has been recognized to affect the imprint quality.26 Despite this limitation, the three volume distributions obtained with three different imprints greatly overlap with each other. We also note that the volume standard deviation is much lower than the 10% limit necessary for proper quantification.27
The variability of the partitioning step critically depends on the wettability of the substrate to the partitioning oil. A hydrophilic substrate permits to perform self-filling operation at the cost of a higher partitioning variability.16 This is a major difference between our approach and self-filling strategies that cannot provide partitions with a tight volume distribution. Finally, our design should be adaptable to other materials that have similar wetting properties to COC and PSA.
Conclusions
In this report, we present a design able to perform partitioning in thermoplastics using capillary-controlled flow. The device performs robustly across a wide range of pressures and can be actuated by a simple micropipette. Partitioning is highly repeatable with a tight droplet volume distribution comparable to microfluidic droplet technologies. Partitioning is very rapid requiring less than 30 s for the total operation. The low-cost fabrication and equipment-free operation will enable the development of digital quantitative assays for low-resource and at-home settings.
Author contributions
Phenix-Lan Quan performed experiments, analyzed data, reviewed, and edited the manuscript. Maria Alvarez-Amador and Yuhe Jiang developed manufacturing techniques and collected preliminary data. Martin Sauzade participated to the conceptualization of the design. Eric Brouzes conceptualized the design, performed experiments, analyzed data, mentored co-authors, and wrote the manuscript.
Conflicts of interest
MS and EB are co-inventors on a patent filed by Stony Brook University to protect the design presented here.
Acknowledgements
The project was initiated thanks to a grant from NIH-NCI (R01 CA181595).
References
- P. L. Quan, M. Sauzade and E. Brouzes, dPCR: A Technology Review, Sensors, 2018, 18(4), 1271 CrossRef.
- A. C. Hatch, J. S. Fisher, A. R. Tovar, A. T. Hsieh, R. Lin, S. L. Pentoney, D. L. Yang and A. P. Lee, 1-Million droplet array with wide-field fluorescence imaging for digital PCR, Lab Chip, 2011, 11(22), 3838–3845 RSC.
- C. M. Hindson, J. R. Chevillet, H. A. Briggs, E. N. Gallichotte, I. K. Ruf, B. J. Hindson, R. L. Vessella and M. Tewari, Absolute quantification by droplet digital PCR versus analog real-time PCR, Nat. Methods, 2013, 10(10), 1003–1005 CrossRef CAS PubMed.
- Z. Chen, P. Liao, F. Zhang, M. Jiang, Y. Zhu and Y. Huang, Centrifugal micro-channel array droplet generation for highly parallel digital PCR, Lab Chip, 2017, 17(2), 235–240 RSC.
- Q. Zhong, S. Bhattacharya, S. Kotsopoulos, J. Olson, V. Taly, A. D. Griffiths, D. R. Link and J. W. Larson, Multiplex digital PCR: breaking the one target per color barrier of quantitative PCR, Lab Chip, 2011, 11(13), 2167–2174 RSC.
- J. Madic, A. Zocevic, V. Senlis, E. Fradet, B. Andre, S. Muller, R. Dangla and M. E. Droniou, Three-color crystal digital PCR, Biomol. Detect. Quantif., 2016, 10, 34–46 CrossRef CAS PubMed.
- T. Morrison, J. Hurley, J. Garcia, K. Yoder, A. Katz, D. Roberts, J. Cho, T. Kanigan, S. E. Ilyin, D. Horowitz, J. M. Dixon and C. J. H. Brenan, Nanoliter high throughput quantitative PCR, Nucleic Acids Res., 2006, 34(18), e123–e123 CrossRef PubMed.
- D. E. Cohen, T. Schneider, M. Wang and D. T. Chiu, Self-Digitization of Sample Volumes, Anal. Chem., 2010, 82(13), 5707–5717 CrossRef CAS PubMed.
- E. A. Ottesen, J. W. Hong, S. R. Quake and J. R. Leadbetter, Microfluidic digital PCR enables multigene analysis of individual environmental bacteria, Science, 2006, 314(5804), 1464–1467 CrossRef CAS.
- F. Shen, W. Du, J. E. Kreutz, A. Fok and R. F. Ismagilov, Digital PCR on a SlipChip, Lab Chip, 2010, 10(20), 2666–2672 RSC.
- K. A. Heyries, C. Tropini, M. Vaninsberghe, C. Doolin, O. I. Petriv, A. Singhal, K. Leung, C. B. Hughesman and C. L. Hansen, Megapixel digital PCR, Nat. Methods, 2011, 8(8), 649–651 CrossRef CAS.
- A. M. Thompson, A. Gansen, A. L. Paguirigan, J. E. Kreutz, J. P. Radich and D. T. Chiu, Self-Digitization Microfluidic Chip for Absolute Quantification of mRNA in Single Cells, Anal. Chem., 2014, 86(24), 12308–12314 CrossRef CAS PubMed.
- M. E. Dueck, R. Lin, A. Zayac, S. Gallagher, A. K. Chao, L. Jiang, S. S. Datwani, P. Hung and E. Stieglitz, Precision cancer monitoring using a novel, fully integrated, microfluidic array partitioning digital PCR platform, Sci. Rep., 2019, 9(1), 19606 CrossRef CAS.
- Qiagen, https://www.qiagen.com/us/products/instruments-and-automation/pcr-instruments/qiacuity-digital-pcr-system. Company website, 2021.
- S. Padmanabhan, J. Y. Han, I. Nanayankkara, K. Tran, P. Ho, N. Mesfin, I. White and D. L. DeVoe, Enhanced sample filling and discretization in thermoplastic 2D microwell arrays using asymmetric contact angles, Biomicrofluidics, 2020, 14(1), 014113 CrossRef CAS.
- A. J. Sposito and D. L. DeVoe, Staggered trap arrays for robust microfluidic sample digitization, Lab Chip, 2017, 17(23), 4105–4112 RSC.
- C. Holtze, A. C. Rowat, J. J. Agresti, J. B. Hutchison, F. E. Angile, C. H. Schmitz, S. Koster, H. Duan, K. J. Humphry, R. A. Scanga, J. S. Johnson, D. Pisignano and D. A. Weitz, Biocompatible surfactants for water-in-fluorocarbon emulsions, Lab Chip, 2008, 8(10), 1632–1639 RSC.
-
K. Zhou and I. Papautsky, Optimization of COC hot embossing with soft PDMS tools, in MOEMS-MEMS 2007 Micro and Nanofabrication, SPIE, 2007, vol. 6465 Search PubMed.
- M. Sauzade, L. Li, T. Bakowski, H. H. Strey and E. Brouzes, Multi-layering of SU-8 exhibits distinct geometrical transitions from circular to planarized profiles, Biomicrofluidics, 2020, 14(1), 014116 CrossRef CAS PubMed.
- J. Schindelin, I. Arganda-Carreras, E. Frise, V. Kaynig, M. Longair, T. Pietzsch, S. Preibisch, C. Rueden, S. Saalfeld, B. Schmid, J.-Y. Tinevez, D. J. White, V. Hartenstein, K. Eliceiri, P. Tomancak and A. Cardona, Fiji: an open-source platform for biological-image analysis, Nat. Methods, 2012, 9(7), 676–682 CrossRef CAS.
- R. Tewhey, J. B. Warner, M. Nakano, B. Libby, M. Medkova, P. H. David, S. K. Kotsopoulos, M. L. Samuels, J. B. Hutchison, J. W. Larson, E. J. Topol, M. P. Weiner, O. Harismendy, J. Olson, D. R. Link and K. A. Frazer, Microdroplet-based PCR enrichment for large-scale targeted sequencing, Nat. Biotechnol., 2010, 27(11), 1025–1031 CrossRef.
- M. Serra, I. Pereiro, A. Yamada, J. L. Viovy, S. Descroix and D. Ferraro, A simple and low-cost chip bonding solution for high pressure, high temperature and biological applications, Lab Chip, 2017, 17(4), 629–634 RSC.
- R. Scanga, L. Chrastecka, R. Mohammad, A. Meadows, P. L. Quan and E. Brouzes, Click Chemistry Approaches to Expand the Repertoire of PEG-based Fluorinated Surfactants for Droplet Microfluidics, RSC Adv., 2018, 8(23), 12960–12974 RSC.
- P. Vulto, S. Podszun, P. Meyer, C. Hermann, A. Manz and G. A. Urban, Phaseguides: a paradigm shift in microfluidic priming and emptying, Lab Chip, 2011, 11(9), 1596–1602 RSC.
- E. Brouzes, T. Kruse, R. Kimmerling and H. H. Strey, Rapid and continuous magnetic separation in droplet microfluidic devices, Lab Chip, 2015, 15(3), 908–919 RSC.
- C. Masciullo, A. Sonato, F. Romanato and M. Cecchini, Perfluoropolyether (PFPE) Intermediate Molds for High-Resolution Thermal Nanoimprint Lithography, Nanomaterials, 2018, 8(8), 609 CrossRef.
- J. F. Huggett, S. Cowen and C. A. Foy, Considerations for digital PCR as an accurate molecular diagnostic tool, Clin. Chem., 2015, 61(1), 79–88 CrossRef CAS PubMed.
|
This journal is © The Royal Society of Chemistry 2024 |
Click here to see how this site uses Cookies. View our privacy policy here.