DOI:
10.1039/D3BM01151H
(Review Article)
Biomater. Sci., 2024,
12, 92-107
Microwave-assisted synthesis of composites based on titanium and hydroxyapatite for dental implantation
Received
10th July 2023
, Accepted 19th October 2023
First published on 26th October 2023
Abstract
Titanium (Ti) and its alloys are widely used in clinical practice. As they are not bioactive, hydroxyapatite (HA) is commonly used to modify them. This study offered a review of microwave-assisted synthesis of composites based on Ti and HA for dental implantation by exploring their interaction mechanisms with microwave and features of two main techniques, namely microwave coating and sintering, along with current challenges and potential solutions in the field. It was shown that microwave coating enables rapid deposition of HA, but suffers from problems such as uneven coating thickness, poor integrity and unstable composition of the products. They can be solved by creating interlayers, combining the spin coating technique, etc. Unlike microwave coating, microwave sintering can effectively modify the mechanical properties of the composites, despite the shortcomings of excessive elastic moduli and potential HA decomposition. These issues are expected to be addressed by adding alloying elements and employing appropriate materials as space holders and ion-doped HA for sintering.
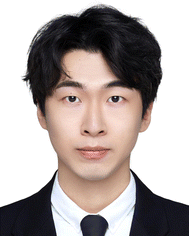 Shangyong Zuo | Shangyong Zuo received his B.E. degree in Materials Science and Engineering from Kunming University of Science and Technology as an outstanding graduate in 2021. Since 2021, he has been with Central South University to pursue his M.S. degree in Materials and Chemical Engineering. His current research project is microwave-assisted synthesis of titanium alloy-based dental materials. |
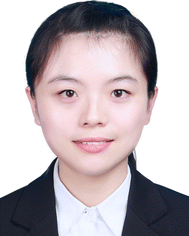 Qian Peng | Qian Peng, Associate Research Fellow of Xiangya College of Stomatology, Central South University, received her M.S. and Ph.D. degrees from Central South University in 2017 and 2020, respectively. She received the Hunan Provincial Science Fund for Excellent Young Scholars and the Youth Fund of the National Natural Science Foundation of China. Her main research areas are synthesis and applications of dental materials. |
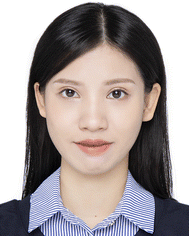 Ting Luo | Ting Luo graduated from Hunan University of Chinese Medicine. Since 2021, she has been pursuing her M.S. degree in Dentistry at Xiangya School of Stomatology, Central South University. Her current research project is microwave-assisted synthesis of titanium alloy-based dental materials. |
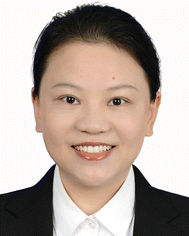 Yuehong Wang | Yuehong Wang, Associate Research Fellow of Xiangya College of Stomatology, Central South University, received her M.S. and Ph.D. degrees from Central South University in 2008 and 2011, respectively, and was jointly trained with Yale University during her Ph.D. study. She received the Youth Fund of the National Natural Science Foundation of China. Her main research area is dental implantation. |
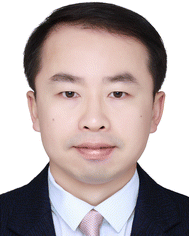 Zhiwei Peng | Zhiwei Peng, Professor of School of Mineral Processing and Bioengineering, Central South University, received his Ph.D. degree from Michigan Technological University in 2012. He is a recipient of 6 grants of the National Natural Science Foundation of China and the Hunan Provincial Science Fund for Distinguished Young Scholars. His main research areas are microwave metallurgy, ferrous metallurgy, and preparation of functional materials. |
1. Introduction
Titanium (Ti) is a kind of metal with high specific strength, superior corrosion resistance and excellent biocompatibility, having wide applications in clinical practice.1 Its alloys, such as Ti6Al4V, can overcome the inherent limitations of pure Ti to some extent due to their better properties,2 including higher corrosion resistance and greater compressive strength.3
Pure Ti and Ti6Al4V have common defects, such as bio-inertia, poor cell adhesion, and low adhesion strength to bone tissue. The elastic moduli of Ti and Ti alloys are 55–117 GPa, while that of human bone is only 14–20 GPa. This mismatch is responsible for stress shielding, which leads to bone resorption and poor adhesion between implants and autogenous bone.4,5 In clinical use, it is easy to cause implant crack or fracture, resulting in operation failure.6 Hence, it is necessary to modify them using bioactive materials, such as hydroxyapatite (HA).
HA is a significant inorganic component in natural bone. Synthetic HA is close to that in natural bone in terms of physical properties, chemical composition, and biological organization. Moreover, its structural stability and crystallinity can be higher than those found in natural bone.7,8 It is an irreplaceable bioactive component of bone repair and implant materials. However, its mechanical properties, especially bending strength, are poor. Thus, its use is not suitable for load-bearing parts of human body.9 Moreover, HA decomposes or reacts with Ti at high temperatures, producing TiO2, Ca3(PO4)2 (TCP), CaTiO3, Ti5P3 and CaO, which pose challenges to its high temperature processing such as powder sintering.10,11
Obviously, it is necessary to synthesize Ti/HA composites to combine the advantages of individual components. However, there is a natural incompatibility between the metal and ceramics, so the methods of combining them to form stable composite materials are crucial. The methods can be divided into two types. The first type is coating HA on the Ti substrate, which can take advantage of the good mechanical properties of the Ti substrate while isolating it from the body's internal environment to reduce substrate-induced cytotoxicity and to make the implant bioactive. The second type is based on powder metallurgy which combines Ti and HA by sintering of their powders to obtain composite implants with uniform chemical composition and physical properties. By using this method, the elastic modulus of the resulting implant can be reduced more effectively. As these methods require thermal energy input for producing composite materials, traditional resistance heating and unconventional heating techniques such as microwave heating have been used.
Many studies have confirmed that microwave heating could achieve better performance than conventional heating in materials synthesis and processing.12–15 Microwave energy is characterized by the volumetric thermal effect due to its large wavelength, which allows the energy to be absorbed in a large material volume (Fig. 1).16,17 Consequently, there is an outward heat transfer in microwave heating, which is contrary to that in conventional heating. Another feature of microwave energy is called the selective thermal effect because the heating efficiency depends directly on the electromagnetic properties, mainly permittivity and permeability, of the target substance.18 The heating performance of different substances usually varies substantially. In some cases, there are also non-thermal effects which can lower the required processing temperature and induce unexpected phase or microstructural changes under the same conditions as those in conventional heating.19 For the above reasons, microwave heating is widely used for processing of metal powders, which are strong microwave absorbers.20 Meanwhile, its use may restrain the decomposition of some inorganic materials, such as HA, with weak microwave responses. However, there are rare reviews on the preparation of composites based on Ti and HA by microwave coating or sintering for dental implantation.12
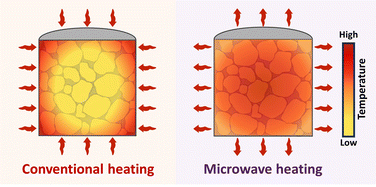 |
| Fig. 1 Comparison between conventional and microwave heating. | |
The aim of this study was to provide a detailed review of microwave-assisted synthesis of composites based on Ti and HA for dental implantation. Because of its similarity to Ti, the most popular titanium alloy, Ti6Al4V, was also considered. The outline of this review is shown in Fig. 2.
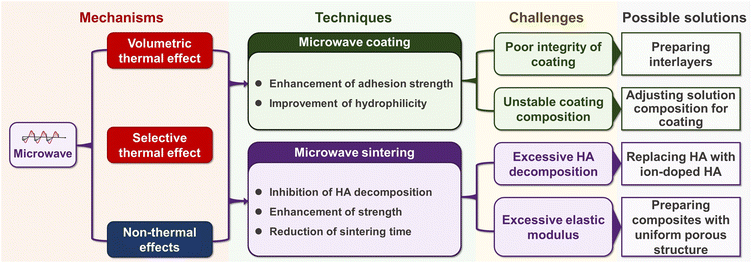 |
| Fig. 2 Outline of the present review. | |
2. Interactions between microwave and Ti, Ti6Al4V or HA
The interactions between metals or alloys and microwave are dependent on the microwave frequency (f, commonly 915 MHz and 2450 MHz), complex permeability (μ) and resistivity (σ).21 To show their comprehensive impacts, a parameter, microwave skin depth (δ), which is defined as the depth at which the microwave electric field intensity drops to 1/e of its surface value, is commonly used and determined using the following formula.22 |  | (1) |
For fine metal powders, such as Ti powder having a size of several tens of micrometers, their microwave skin depths are usually comparable to the powder size, which enables their effective microwave absorption and heating, eventually facilitating sintering.23
For Ti powder, after it is pressed into compacts, there will be a large number of pores or gaps between its particles, which can reduce the reflection of microwave.24,25 In some cases, a oxide layer is formed on the surface of Ti powder. It has a dielectric loss, which will also promote microwave coupling.26,27 Therefore, the Ti powder pressed into compacts can be effectively sintered by microwave. For example, Ti and Ti6Al4V powders having a particle size of less than 75 μm were used as the raw materials for preparing cylindrical compacts with a diameter of 56 mm after pressing at 430 MPa for sintering at 1300 °C for 30 min under microwave irradiation at 915 MHz. The densities of the sintered samples were found to be 94%–96% of the theoretical maximum density.28
Unlike Ti and its alloys, HA is a typical inorganic component. The measurement of complex permittivity (ε) of HA at about 2450 MHz showed that for HA compacts after pressing, the relative dielectric constant (ε′r) of the samples sintered at 1000 °C increased from 2.67 to 3.89 as the forming pressure increased from 3.04 MPa to 15.20 MPa.29 Its microwave absorption capability could also be determined using the microwave penetration depth (Dp), which is defined as the depth at which the microwave power drops to 1/e of its surface value. The parameter is expressed by the following formula for non-magnetic materials
|  | (2) |
where
λ0 is the microwave wavelength in the free space and
ε′′
r is the relative dielectric loss factor. At 3.04 MPa, the value of
Dp of HA at 1000 °C was found to be 208.63 cm, which decreased to 43.53 cm at 1200 °C. At 15.20 MPa, the value of
Dp at 1000 °C was 55.69 cm, which increased to 163.28 cm at 1200 °C. This opposite trend was due to the larger pore size of the compacts at lower pressure. With the increase of microwave sintering temperature, the pores shrunk due to increased diffusion, resulting in an increase of
ε′′
r. In contrast, at higher pressure, the pore size of the compacts decreased. As the temperature increased, the pores were aggregated under the effect of internal stress, resulting in a decrease of
ε′′
r.
The microwave absorption capabilities of the materials can also be revealed from their heating rates under the same microwave heating conditions, as shown in Table 1. The average heating rate of Ti was 50 °C min−1 during the whole process and the average heating rate of Ti6Al4V was with 44 °C min−1.30 HA required much more microwave power than the former two to achieve the same heating rate.31 It is inferred that the microwave absorption capabilities of Ti and Ti6Al4V powders were better than that of HA powder.
Table 1 Heating rates of different materials under microwave irradiation
Materiala |
Pressing pressure (MPa) |
Compact size (mm) |
Microwave power (W) |
Maximum temperature (°C) |
Heating rate (°C min−1) |
References |
Powder (<150 μm)
|
Ti |
100 |
∅20 × 25 |
300 W; increasing power by 50 W per min after 3 min |
1200 |
50 |
30
|
Ti6Al4V |
100 |
∅20 × 25 |
1200 |
44 |
HA |
220 |
∅10 |
1200 |
1200 |
50 |
31
|
For further comparison, microwave heating of Ti and Ti6Al4V powders with an average particle size of ∼17 μm and needle-like HA powders with a diameter of ∼20 μm and a length of ∼100 μm was performed in a microwave furnace with a SiC susceptor operated at 1000 W and 2450 MHz in argon. Fig. 3 shows their temperature rising curves. It was evident that Ti had a slightly higher heating rate than Ti6Al4V during the entire heating process. Both of them had much higher heating rates than HA before 17 min although the differences became implicit in the later period. The temperature of Ti6Al4V remained higher than that of HA until it heated up to 1050 °C. HA started to melt without decomposition under microwave irradiation at 1038 °C, which was lower than the melting point of HA (1570 °C).32 In other words, it is promising to realize selective heating of Ti and HA to promote the densification of composites considering their different heating rates in the microwave field.
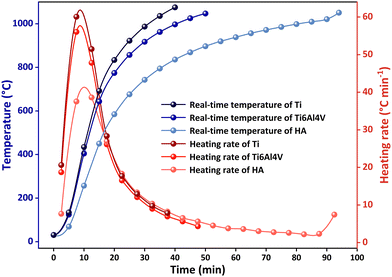 |
| Fig. 3 Temperature rising curves of Ti, Ti6Al4V and HA powders under microwave irradiation at 2450 MHz with the power of 1000 W. | |
3. Microwave-assisted synthesis of composites based on Ti and HA
3.1 Composites prepared by microwave coating
The preparation of HA coatings on Ti or Ti6Al4V surfaces often uses aqueous solutions or sols as media, which leads to a slow deposition process and poor crystallinity of the resulting coatings. Microwave radiation can accelerate the deposition process of HA and reduce the thermal mismatch between the coating and substrate during subsequent calcination because of its volumetric heating and selective heating features. It reduces the generation of cracks in the coating and therefore has great potential in the preparation of composites as dental materials.
3.1.1 Ti or Ti6Al4V/HA composites.
Compared with other techniques, the biomimetic coating technique is able to adjust the coating composition directly by controlling the composition of the corresponding solution. Also, it can avoid the generation of other heterogeneous phases.33 However, conventional biomimetic coating is slow and prone to bacterial growth in the solution, which leads to coating destruction.34 Microwave biomimetic coating can greatly reduce the coating time.35 It has been used to prepare spherical HA coatings in a solution with a Ca/P molar ratio of 1.67 at the optimal heating rate of 60 °C min−1 within 2 min, producing a coating with thickness of 56 μm.36 The microwave technique can also create a special morphology that is unavailable or difficult for conventional biomimetic coating techniques. For example, it produced a rod-like HA coating which had better hydrophilicity and biocompatibility than traditional spherical HA coating because the former could imitate the collagen fibers and resorption traps on the surface of human bone.13,37
In the microwave coating process, pretreatment is often conducted to improve the compatibility between the substrate and coating.38 Acid etching is used to increase the roughness of the substrate surface to enhance the mechanical adhesion between the substrate and HA coating.39 In contrast, alkali etching not only increases the roughness of the substrate surface, but also promotes the transformation of the substrate to the anatase phase, resulting in a lower phase transition activation energy and the generation of more Ti–OH groups, as shown in Fig. 4A.13,40 They will provide good locations for HA nucleation for its rapid coating and enhance the adhesion of the substrate to the HA coating through stronger chemical bonding. Therefore, alkali etching is commonly used in the process of coating HA on Ti or Ti6Al4V surfaces under microwave irradiation. It was found that alkali etching changed the traditional adhesion mode of pure HA coating to the substrate by immersing the substrate in 5 M NaOH solution at 80 °C for 1 h before heating to 600 °C for 1 h.13 Consequently, the density and uniformity of HA coating were enhanced. For improving the comprehensive properties, including antibacterial properties, of the resulting coatings, ion-doped HA can be coated on Ti or Ti6Al4V by microwave biomimetic coating.41,42 The doped HA also facilitates the adhesion of osteoblasts to the implant surface.43–45 For instance, Ag+ is the most commonly used dopant ion because of its antibacterial role.46–48 The study of the substitution behavior of HA in silver nitrate solution demonstrated that microwave could disturb the solvent layer around the ions through its non-thermal effect and significantly change the lattice parameters of HA, thus promoting the diffusion of Ag+ to the interior.17,47 With alkali-treated Ti6Al4V as the substrate, pure HA coatings were prepared under microwave irradiation at 1400 W for 4 min using a solution with a Ca/P molar ratio of 1.67 (0.14 mol L−1 Ca2+). In the same way, a pure silver-doped hydroxyapatite (Ag–HA) coating was prepared for the first time with 4 wt% AgNO3 in the coating solution, with a doping percentage of 12.74%, which showed a sterilization rate of over 99.9% without significant cytotoxicity using the undoped sample as a reference material. However, due to the flake-like surface morphology of Ag-HA, it is not advantageous from a biomimetic point of view.
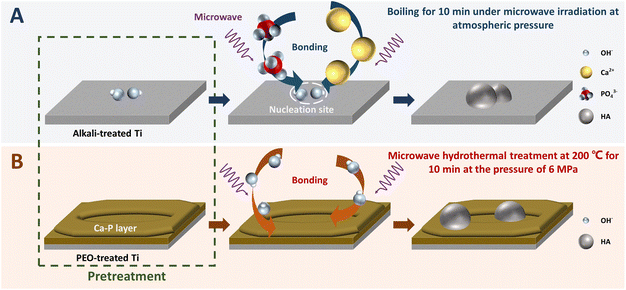 |
| Fig. 4 (A) Mechanism of alkali etching for induction of HA nucleation. (B) Mechanism of microwave hydrothermal (MH) treatment in NaOH solution. | |
3.1.2 Ti or Ti6Al4V/TiO2/HA composites.
A third component may be added for enhancing the properties of the composites composed of Ti or Ti6Al4V and HA or modified HA (e.g., Sr and F-doped HA, namely SrFHA). Recently, Ti6Al4V/SrFHA composite implants were prepared by the microwave biomimetic coating technique with a molar ratio of ions in the coating solution of Ca/Sr/P/F = 4/1/3/1 at 50 °C. The results showed that a TiO2 interlayer appeared between SrFHA and Ti6Al4V after calcination, which increased the adhesion strength between the coating and substrate from 0.68 MPa to 2.41 MPa.49 It proved the promoting role of the TiO2 interlayer in the compatibility between the coating and substrate to some extent. Compared with conventional biomimetic coating, the coatings prepared by the sol–gel technique had higher adhesion strength at relatively high TiO2 fractions.38 It was shown that with TiO2 as an interlayer, a coating with adhesion strength of 37.5 MPa was prepared by the sol–gel technique followed by conventional sintering at 800 °C for 1 h.50 By using the same sol-gel technique, however, the corresponding sintering time under microwave irradiation for producing a TiO2/HA/TCP composite coating, which was initially prepared by mixing TiO2 particles into the calcium phosphate (Ca–P) sol (mass ratio of the solute of 1.5/1) coated on Ti6Al4V, could be shortened to 10 min at 800 °C with the coating adhesion strength of 34 MPa.16 The adhesion strength was believed to be controlled by the reaction between TiO2 and HA at high temperatures which produced gas that affected the coating integrity.
3.1.3 Ti/Ca–P/HA composites.
The Ca–P compounds are usually coated onto the titanium substrate to ensure sufficient adhesion strength of the resulting coating.51 However, its poor crystallinity is not favorable for maintaining biocompatibility.52 Microwave hydrothermal (MH) technique is used for converting amorphous Ca–P compounds to highly crystalline HA coatings.53 It can be divided into two main types. The first type is performed in solutions containing Ca2+ and PO43−, using the amorphous Ca–P coating as the dominant region for nucleation to induce HA deposition.54 However, the formed gradient coatings are often too thick (>100 μm) and easily flake off.55,56 The second type is based on the use of Ca2+ and PO43− released by the degradation of the original coating as a raw material for the new HA coating, reacting with OH− to form HA. Therefore, the coating thickness decreases gradually as the MH process proceeds. It was reported that after MH treatment of a porous plasma electrolytic oxidation (PEO) coating on a Ti substrate at 200 °C for 10 min with NaOH concentration of 0.01 mol L−1, the adhesion strength of the coating reached 47.5 MPa.15 Its mechanism is shown in Fig. 4B. By PEO-MH treatment, uniformly doped hexagonal prismatic Si-HA nanorods were obtained.15,57 The nanorods were flexible and deformed at an angle of up to 90° under load, which allowed implants prepared by the coating method to achieve a lower elastic modulus. The morphology could also be tuned by varying the concentration of NaOH solution. As the concentration of NaOH solution increased from 0.01 mol L−1 to 0.5 mol L−1, the nanorods gradually transformed into petal-like crystals.57 Its effect on the osseointegration process was also evaluated by animal experiments. Fig. 5A shows the micro-CT colored images of tibias of New Zealand rabbits containing Ti, Ti with PEO coating, and Ti with PEO-MH coating. For the untreated Ti, its interface with new bone tissue (green color) was invaded by soft tissue (red color), which resulted in poor adhesion. For Ti with PEO coating and Ti with PEO-MH coating, their invasion degrees were reduced considerably, showing their better osseointegration.
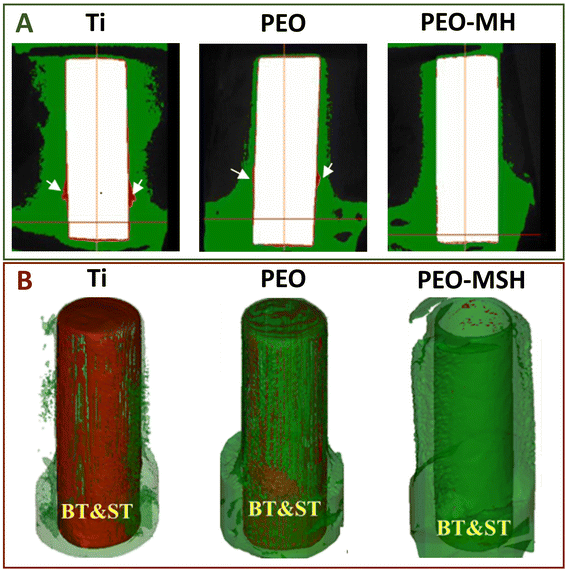 |
| Fig. 5 (A) Micro-CT colored images of tibias of New Zealand rabbits containing Ti, Ti with PEO coating, and Ti with PEO-MH coating after 16 weeks of healing. Reproduced from ref. 15 with permission from American Chemical Society, copyright 2019. (B) Micro-CT colored 3D reconstruction images of tibias of New Zealand rabbits containing Ti, Ti with PEO coating, and Ti with PEO-MSH coating after 16 weeks of healing. Reproduced from ref. 41 with permission from Elsevier, copyright 2020. Note: the red part and the part indicated by white arrows: soft tissue, BT: bone tissue and ST: soft tissue. | |
Based on the MH technique, the microwave steam–hydrothermal (MSH) technique was developed.41 The duration of MSH treatment significantly affected the surface morphology of the sample, with the diameter and length of the nanorods decreased as the treatment time increased from 10 min to 60 min (Fig. 6) which enhanced the coating density. The push-out force of Ti with PEO-MSH coating was 180 N, which was higher than that of Ti with PEO-MH coating (150 N), showing its enhanced adhesion strength to the substrate. The corresponding in vivo biological properties have been evaluated in animal studies (Fig. 5B). However, there were potential risks of non-uniform coating thickness and unstable composition after treatment, which led to substrate exposure.46,54 It was caused by the insufficient concentration of Ca2+and PO43− generated by the degradation of amorphous Ca–P in the solution alone. Further adjustments are required for optimizing the solution composition for the treatment (Table 2).
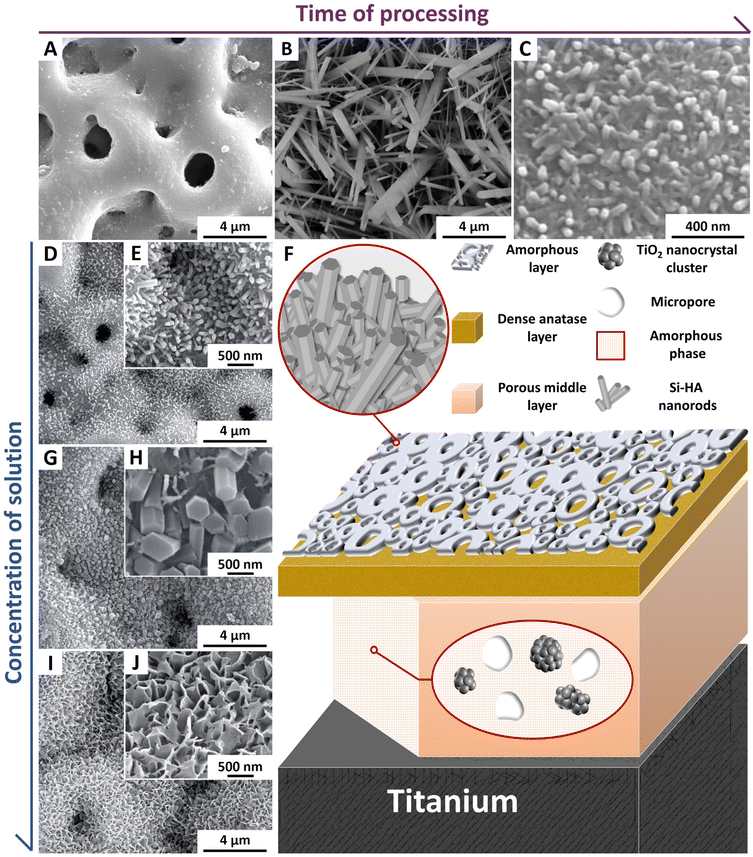 |
| Fig. 6 (A–C) SEM images of PEO coatings after MSH treatment for 0 min, 10 min and 60 min, respectively. Reproduced from ref. 41 with permission from Elsevier, copyright 2020. (D, E and G–J) SEM images of PEO coating after 10 min of MH treatment with different NaOH concentrations in the precursor solution. Reproduced from ref. 57 with permission from the authors, copyright 2021. (F) Schematic illustration of sample microstructure. | |
Table 2 Properties of the composites prepared by different microwave coating techniques
Composition |
Substrate |
Composition of solution or sol |
Pretreatment |
Microwave processing parameters |
pH of coating solution or sol |
Ca/P molar ratio of coating solution or sol |
Product properties |
References |
Vickers microhardness (HV) |
Contact angle (°) |
Interfacial adhesion strength (MPa) |
Coating morphology |
n/r-not reported |
Ti or Ti6Al4V/HA |
Ti |
Ca(NO3)2·4H2O + (NH4)2HPO4 |
— |
2 min |
n/r |
1.67 |
n/r |
79 (water) |
n/r |
Globular morphology |
36
|
900 W |
Ti6Al4V |
Ca(NO3)2·4H2O + Na2HPO4·12H2O |
Alkali etching; heat treatment |
10 min |
6.50 |
3 |
n/r |
n/r |
n/r |
Plate-like morphology |
13
|
900 W |
4 |
n/r |
n/r |
n/r |
Rod-like morphology |
Ti6Al4V |
Ca(NO3)2·4H2O + NaH2PO4 +NaHCO3 |
Alkali etching; heat treatment |
4 min |
n/r |
1.67 |
n/r |
20.0 (water) |
n/r |
Flake-like morphology |
19
|
1200 W |
Ti6Al4V |
CaCl2 + (NH4)2HPO4 |
— |
5 min |
n/r |
1.67 |
460.5 |
42.1 (SBF) |
n/r |
Globular morphology |
33
|
— |
3Ti6Al4V /TiO2/HA |
Ti6Al4V |
CaCl2 + SrCl2 + NaH2PO4 + NH4F + NaHCO3 + standard SBF |
Acid etching; alkali etching; heat treatment |
5 min |
n/r |
1.67 (Sr/P + Ca/P) |
329 |
7.24 (water) |
2.41 |
Rod-like morphology |
49
|
50 °C |
Ti6Al4V |
CaCO3 + CaHPO4·2H2O |
Laser ablation; alkali etching |
10 min |
4.00 |
1.67 |
301.9 |
22.4 (water) |
34.6 |
Globular morphology |
16
|
800 °C |
Ti/Ca–P/HA |
Ti |
NaOH |
PEO treatment |
10 min |
11.4 (NaOHaq, 0.01 M) |
— |
n/r |
9.3 (water) |
47.5 |
Rod-like morphology |
15
|
200 °C |
3.2 Composites prepared by microwave sintering
HA tends to decompose above 1300 °C.58,59 Ti and its oxide, TiO2, react with HA during the sintering process, lowering the decomposition temperature of HA.60 The rapid low-temperature microwave sintering helps in avoiding HA decomposition.61
3.2.1 Ti/HA composites.
In Ti/HA composites prepared by microwave sintering, there were some micro-pores with a size of only 5–30 μm. These pores were concentrated at the interfaces between HA and Ti powder particles, exerting a negative impact on the product strength. It can be explained by the Kirkendall effect.62,63 Additionally, some phase transformations, such as decomposition of HA, will lead to the formation of micro-pores.54 It was reported that the compressive strength of implants with macro-pores (>100 μm) decreased less with increasing porosity compared to the implants with micro-pores.5,62,64,65 The in situ synthesis of HA by microwave sintering contributed to the formation of macroporous structure of Ti/HA composites. After in situ microwave sintering of Ti powder (85 wt%), CaHPO4·2H2O powder (4.2 wt%) and CaCO3 powder (10.8 wt%) at 1 kW for 10 min and then at 2 kW for 5 min,66 the resulting composite contained HA (7.6 vol%), TCP (2.8 vol%) and other phosphates. During the process, gases were generated, leading to interconnections between some pores (Fig. 7A). Owing to uniform microwave sintering, the composite showed only minor deviations of pore distribution and thus high compressive strength (212.6 MPa).
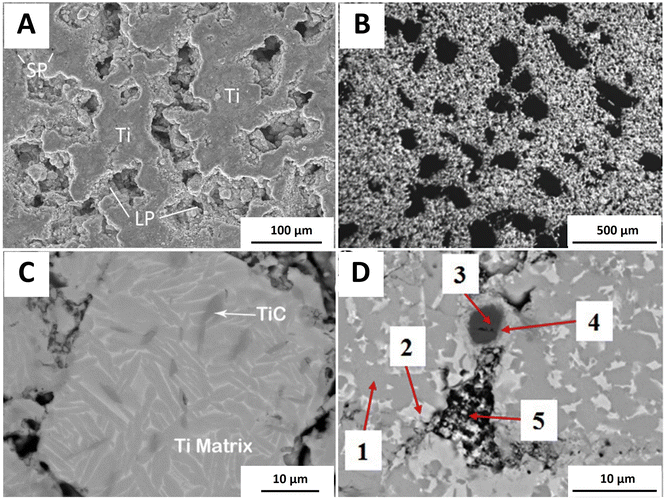 |
| Fig. 7 (A) Porous structure obtained by microwave-assisted in situ synthesis technique. Reproduced from ref. 66 with permission from Elsevier, copyright 2015. SP: small pore and LP: large pore. (B) Porous structure obtained by the microwave-assisted space holder technique. Reproduced from ref. 63 with permission from Elsevier, copyright 2020. (C) Formation of irregularly shaped TiC in the Ti matrix. Reproduced from ref. 71 with permission from Elsevier, copyright 2013. (D) Microscopic morphology of boronized Ti6Al4V/HA composites. 1: α-Ti, 2: β-Ti, 3: TiB2, 4: TiB, and 5: HA + TCP. Reproduced from ref. 60 with permission from Elsevier, copyright 2020. | |
The composite was observed to deposit significantly after 3 days of SBF immersion, which proved its excellent apatite induction ability. The deposits were concentrated in the pore sites due to the presence of large amounts of HA and TCP phases, whose degradation led to the deposition of Ca2+ and PO43− in the surrounding areas of the pores. Note that TCP could be degraded faster in vivo compared to HA. For better illustration of its biocompatibility, the effect of TCP in the composite on cell mineralization and osteogenesis should be further explored.
3.2.2 Ti/Nb/HA composites.
Nb can be added to produce new Ti-based composites as dental implants with lower elastic moduli, e.g., Ti/Nb/HA composite. Its combination with the space holder technique is more effective.67 Because the location of macro-pores is determined before sintering, the space holder technique combined with microwave sintering is able to avoid pore segregation to the maximum extent. The pore structure and distribution obtained by the microwave-assisted space holder technique are shown in Fig. 7B. Ti powder (≤25 μm), Nb powder (≤25 μm), and HA powder (≤2 μm) with a mass ratio of 5/3/2 were used as raw materials and pressed into ∅20 × 10 cylindrical compacts at 300 MPa for microwave sintering at 1400 W for 5 min.5 The composites with different porosities were prepared by controlling the addition of urea as a space holder. The average compressive strength of the composites with porosities of 18–20% and 53–57% was 395 MPa and 205 MPa, with compressive moduli of 29 GPa and 15 GPa, respectively, showing the same proportional decrease in compressive strength and compressive modulus with increasing porosity. It further demonstrated that the average pore size after microwave sintering was larger than the urea particle size when it was smaller than 125 μm. However, the average pore size after microwave sintering was smaller than the urea particle size when it was larger than 250 μm. In order to regulate the product pore size precisely, this phenomenon needs to be explored.
In the potentiodynamic polarization test in SBF, the corrosion current density decreased from 3.75 μA cm−2 to 1.05 μA cm−2 as the HA addition increased from 0 wt% to 20 wt%, indicating the excellent corrosion resistance of HA in the composites due to its excellent bioactivity.
3.2.3 Ti6Al4V/TiC/HA composites.
The necessary time for microwave sintering can be further reduced by adding a microwave susceptor that enhances microwave absorption in the raw materials. Multi-walled carbon nanotubes (MWCNTs) are commonly used as microwave susceptors for synthesis of biomaterials.68,69 Ti6Al4V/TiC/HA composites were prepared from Ti6Al4V powder (78.7 vol%), MWCNTs (13.4 vol%) and HA powder (7.9 vol%) by pressing their mixture (∅15 × 22 mm) at 200 MPa for subsequent microwave sintering at 1400 W for 2 min, with the maximum sample temperature of 1620 °C.70,71 The porosity was about 26% and the compressive strength was 93.2 MPa, with compressive modulus of 2.9 GPa. Although the modulus was reduced, the compressive strength also declined significantly, which was probably due to the thermal mismatch between Ti6Al4V and HA exacerbated by the excessively high heating rate. In addition, as shown in Fig. 7C, adding MWCNTs caused the formation of irregularly shaped TiC in the Ti matrix, which might cause cytotoxicity, demanding further investigation.
3.2.4 Boronized Ti6Al4V/HA composites.
The coefficient of thermal expansion of TiB is between those of Ti6Al4V and HA. It was reported that the boronized Ti6Al4V/HA composite could be prepared by microwave sintering at 1050 °C for 30 min under an Ar atmosphere using Ti6Al4V powder of 15–50 μm, TiB2 powder of 4–8 μm, and needle-like HA with a diameter less than 20 μm and a length less than 100 μm as raw materials.12,60,72 Its micromorphology is shown in Fig. 7D. The main HA phase was preserved after sintering and distributed at the interstitial positions of Ti6Al4V particles. Moreover, an obvious TiB overlayer was formed on the surfaces of TiB2 particles, which connected the Ti6Al4V particles separated by HA, reducing the thermal mismatch and preventing the formation of cracks. The TiB2/TiB particles acted as a secondary phase in the substrate and hindered the dislocation movement to form the reinforcing phase. The composite prepared by sintering with the additions of 5 wt% TiB2 and HA had compressive strength of 351.10 MPa, Vickers microhardness of 466.39 HV and compressive modulus of 39.2 GPa. It should be pointed out that there was only partial transformation of TiB2 to TiB during the sintering process. With higher transfromaton percentage of TiB2, the composite strength is expected to be further enhanced. In addition, by creating more porous structure, even lower composite modulus is achievable.
The bioactivity of implant materials can be tested with human osteosarcoma cells (MG-63). Short filopodia were observed on the MG-63 cells adhered to the previously mentioned Ti/Nb/HA composite (Fig. 8A). In contrast, long filopodia were formed on the boronized Ti6Al4V/HA composite with a clear tendency to connect into whole sheets and extend toward the pore interior (blue arrows in Fig. 8B), thus promoting cell adhesion on the surface. This was because the addition of other alloying elements such as Nb tended to destroy the biocompatibility of Ti or Ti6Al4V, while boronized Ti6Al4V still maintained the performance. The differentiation ability of the cells is usually characterized by alkaline phosphatase (ALP) activity assay. The higher degree of purple staining proves the higher ALP activity. The degree of mineralization of cells can be characterized by using alizarin red to stain the mineralized nodules of the cells, in which a higher degree of red staining proves the better mineralization ability of cells on the material. According to Fig. 8C and D, boronization was not favorable for cell osteogenic differentiation, while the addition of HA had a significant promoting effect on the differentiation. The push-out strength of the composite was tested 12 weeks after implantation into the rabbit femur. The boronized Ti6Al4V/HA composite had the highest push-out force (3.19 MPa), which was consistent with the changing pattern of cellular osteogenic differentiation ability revealed in Fig. 8.73 Obviously, the relatively low in situ conversion of TiB2 might have a negative impact on the mechanical properties and even biocompatibility of the composite. This problem needs to be overcome and deserves more attention (Table 3).
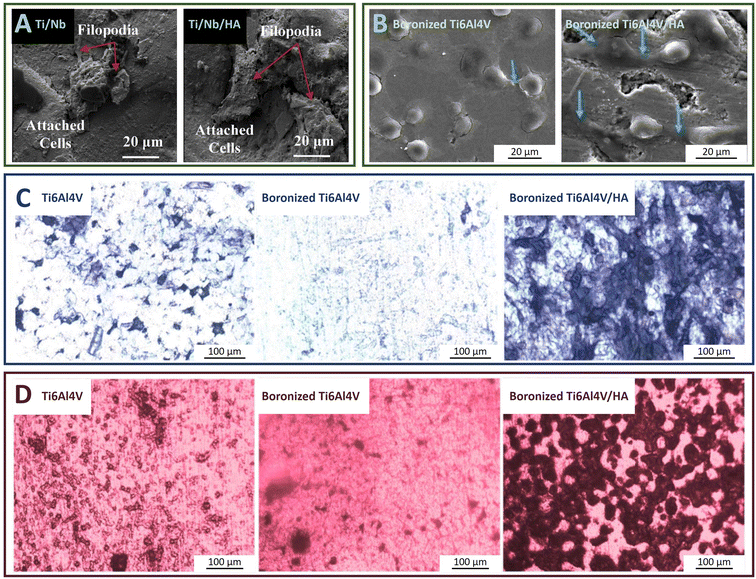 |
| Fig. 8 (A) Cell adhesion patterns on the surfaces of Ti/Nb and Ti/Nb/HA composites after 24 h of MG-63 cell culture. Reproduced from ref. 5 with permission from Elsevier, copyright 2020. (B) Cell adhesion patterns on the surfaces of boronized Ti6Al4V and boronized Ti6Al4V/HA composite after 12 h of MG-63 cell culture; (C) ALP staining of MG-63 cells cultured on the surfaces of Ti6Al4V, boronized Ti6Al4V and boronized Ti6Al4V/HA composite for 7 d; (D) Alizarin red staining of MG-63 cells cultured on the surfaces of Ti6Al4V, boronized Ti6Al4V and boronized Ti6Al4V/HA composite for 14 d. Reproduced from ref. 73 with permission from the authors, copyright 2022. | |
Table 3 Properties of the composites prepared by different microwave sintering techniques
Raw materials |
Pressing parameters |
Atmosphere |
Sintering parameters |
Product properties |
Use of space holder materials |
References |
Porosity (%) |
Compressive strength (MPa) |
Elastic modulus (GPa) |
Vickers microhardness (HV) |
Density (g cm−3) |
Ti (85 wt%) + CaHPO4·2H2O (10.8 wt%) + CaCO3 (4.2 wt%) |
226 MPa |
Air |
1000 W, 10 min; 2000 W, 5 min |
26.2 |
212.6 |
12.5 |
n/r |
n/r |
No |
66
|
120 s |
Ti (50 wt%) + Nb (30 wt%) + HA (20 wt%) |
300 MPa |
Air |
1400 W, 5 min |
53–57 |
205 |
15 |
n/r |
n/r |
Yes |
5
|
Ti6Al4V (78.7 vol%) + MWCNTs (13.4 vol%) + HA (7.9 vol%) |
200 MPa |
Air |
1400 W, 2 min |
27.8 |
93.2 |
2.9 |
556.8 |
2.9 |
No |
70
|
200 s |
Ti6Al4V (90 wt%) + HA (5 wt%) + TiB2 (5 wt%) |
900 MPa |
Argon |
1050 °C, 30 min |
11.3 |
351.1 |
39.2 |
462.8 |
4.13 |
No |
60 and 72 |
90 s |
4. Discussion
Microwave heating has several advantages over traditional heating in preparing composites based on Ti and HA as dental implants. Firstly, microwave energy provides energy undulation for nucleation, which accelerates the nucleation rate and refines the crystal grains. Secondly, it has the potential to activate doping of ions in HA for preparing composite implants.74 Thirdly, it can promote diffusion of ions, reducing the necessary temperature and time for sintering. Fourthly, microwave energy enables selective heating of composite components with different microwave absorption capabilities.
For improving the bioactivity of dental implants, microwave coating can be improved. As shown in Fig. 9, for microwave biomimetic coating, pretreatment generally increases the deposition efficiency and densifies the coating with morphological modulation. However, it is difficult to solve the problem of coating cracking which causes poor coating strength and even peeling. It was reported that grafting polydopamine (PDA)/casein phosphopeptide (CPP) bilayers on the Ti surface was useful for enhancing adhesion strength with prevention of cracking.75,76 This approach may also be used in conjunction with microwave biomimetic coating to further improve the performance of HA coatings.
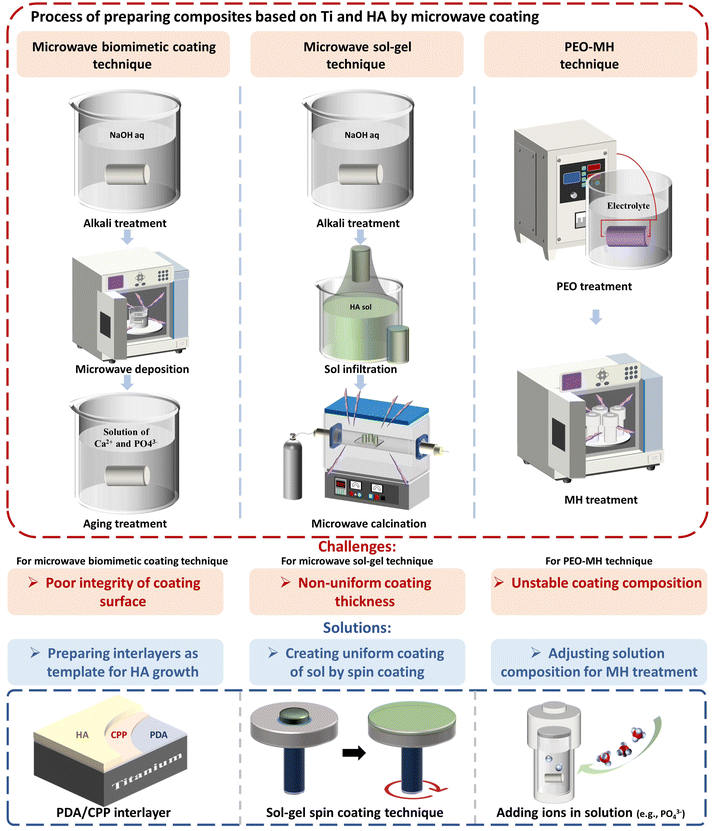 |
| Fig. 9 Processes, challenges and solutions for preparation of composites based on Ti and HA by microwave coating. | |
The microwave sol–gel technique has advantages in the preparation of ultra-thin coatings. Although microwave allows rapid completion of transformation of sol to coating, it is not enough to solve the thermal mismatch between the coating and substrate. TiO2 as a sol component can enhance the adhesion strength of the coating to the substrate, but the large contact area between TiO2 and HA often induces their mutual reaction. Therefore, TiO2 should serve as an interlayer and other techniques, such as spin coating, can be applied together to obtain uniform HA coating with an appropriate thickness.
Traditional HA doping may have poor results due to excessive lattice distortion energy or low ion diffusion efficiency. In these cases, microwave can activate the doping process and thus improve the coating performance. Fluorine-doped hydroxyapatite (FHA) is a bioceramic with antibacterial function and better thermal stability, so it is promising to be used in the sol–gel technique to inhibit the reaction with the TiO2 interlayer. Highly biomimetic FHA coatings have been prepared on the surface of magnesium alloy using the microwave technique. However, they were rarely prepared on the surface of Ti implants, which demands further research.37
Among all microwave coating techniques for preparing the composites, the PEO-MH technique can be used to produce coating with very high adhesion strength to the substrate via solution reactions.77 However, Ca2+ and PO43− obtained from degradation of the original Ca–P coating often produce HA with a unstable Ca/P molar ratio. Therefore, in the PEO-MH treatment, it is useful to add ions properly to the solution to make its composition during the reaction process close to the stoichiometric ratio of HA. The key point is to combine the advantages of microwave biomimetic coating and PEO-MH technique to ensure the high adhesion strength of coating with stable chemical composition and biocompatibility.
For meeting the mechanical specifications of dental implants, the Ti and HA-based composites prepared by microwave sintering have to be improved. The challenges and solutions for microwave sintering are shown in Fig. 10. In order to maintain the microhardness, Ti or Ti alloy (423–449 HV) often serves as the main phase. To obtain compressive strength greater than that of human cortical bone (170–193 MPa), thermal decomposition of HA should be inhibited in microwave sintering. For achieving an elastic modulus closer to that of human bone (14–20 GPa), implants with porous structure are desired.4,70
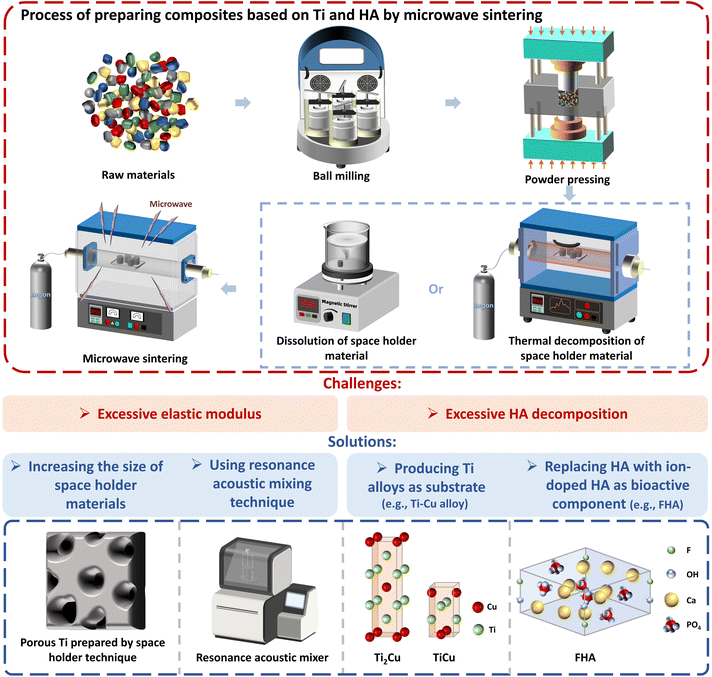 |
| Fig. 10 Processes, challenges and solutions for preparation of composites based on Ti and HA by microwave sintering. | |
The technique of in situ microwave sintering is accompanied by phase transformation at high temperatures, which enables a stable chemical bonding between Ti and HA but causes serious decomposition of HA, leading to lower bioactivity. Moreover, the segregation of pores can create weak surfaces, negatively affecting the strength. The space holder technique is more favorable to obtain a homogeneous porous structure. However, in its application to microwave sintering, the strain of the composite in the elastic deformation region is small. Hence, the elastic modulus cannot be effectively reduced, probably due to the small size of the obtained pores. Therefore, the particle size of space holder materials should be optimized. Some other space holder materials, such as NH4HCO3 and starch, can be examined.78,79 In addition, resonance acoustic mixing is recommended to achieve uniform mixing and de-agglomeration of particles without changing the size and shape of space holder materials.80,81
The decomposition of HA can be effectively restrained by taking advantage of selective thermal effect of microwave. In order to retain more HA in the implant product, it is necessary to further reduce the sintering temperature which can be achieved by alloying. For example, producing Ti/Cu alloys as the substrate may be helpful because of their low melting points (below 1000 °C) and superior antibacterial abilities.82–84 Another approach is to apply ion-doped HA with high thermal stability, such as FHA, for sintering.
Among the composite implants prepared by microwave sintering, the boronized Ti6Al4V/HA composite showed compressive strength far exceeding the requirements for clinical applications, which confirmed the advantage of TiB as an intermediate phase. However, there is a potential cytotoxicity of excessive boron.85,86 Further attention should be devoted to the effect of its addition on the biological properties of the implant. Finer TiB2 powders having better microwave absorptivity may be used to facilitate relevant reactions with less addition. Their larger surface area is conducive to their preferred reactions with Ti6Al4V, with inhibition of the decomposition of HA simultaneously, thus improving the mechanical properties and biocompatibility of the resulting composite.
Preparing dental implants is not a simple biomimetic process because the implants should imitate biological autologous tissues, which is still difficult. The microwave coating method is aimed at solving the problem of bioactivity and biocompatibility, while the microwave sintering method is mainly applied to solve the problems concerning mechanical properties. Both methods demand more efforts for exerting their own benefits in preparing the composites.
5. Conclusions
This paper reviewed the preparation of composites based on Ti and HA as dental implants by microwave coating and sintering. Based on the analysis of the advantages and disadvantages of various preparation methods with consideration of mechanical properties, interfacial adhesion and biological properties of the resulting composites, the main bottlenecks and corresponding solutions for preparation of high-quality composites were discussed. The microwave coating method is aimed at solving the problem of cytotoxicity caused by alloying elements and the bio-inert nature of the metal surface. In order to ensure the integrity of the coating during the implantation procedure, it is necessary to increase the adhesion strength between the coating and metal substrate with precise control of the coating thickness by improving the coating method, preparing interlayers, and adjusting the solution composition for coating. The microwave sintering method is considered a promising solution for inhibiting decomposition of HA during the sintering process. Alloying of the substrate and ion doping of HA are expected to further restrain the decomposition of HA in the process. Further reduction of elastic modulus of the composites can be achieved by utilizing the space holder technique and the resonance acoustic mixing technique. With the improvement of microwave coating and sintering methods, the obtained novel composites with superior properties will find more applications in dental implantation.
Conflicts of interest
There are no conflicts to declare.
Acknowledgements
The work was partially sponsored by the National Natural Science Foundation of China under Grant 82201113, the Hunan Provincial Natural Science Foundation of China under Grants 2023JJ20088 and 2021JJ40905, and the Hunan Provincial Health Commission Research Program under Grant 202108052067.
References
- L. Sun, X. Chen, K. Ma, R. Chen, Y. Mao, R. Chao, H. Wang, B. Yu, J. Wang and S. Zhang, Adv. Healthcare Mater., 2023, 12, 2202620 CrossRef CAS PubMed.
- S. S. D. Carter, L. Barbe, M. Tanje and G. Mestres, Biomater. Sci., 2020, 8, 6309–6321 RSC.
- M. C. D. Bino, W. A. Euridice, R. V. Gelamo, N. B. Leite, M. V. da Silva, M. R. de Siervo, M. R. Pinto, P. A. D. Buranello and J. A. Moreto, Appl. Surf. Sci., 2021, 557, 149739 CrossRef.
- J. Guzman, R. D. Nobre, E. R. Nunes, D. L. Bayerlein, R. B. Falcao, E. Sallica-Leva, J. B. F. Neto, H. R. Oliveira, V. L. Chastinet and F. J. G. Landgraf, J. Mater. Res. Technol., 2021, 10, 1372–1381 CrossRef CAS.
- C. Prakash, S. Singh, S. Ramakrishna, G. Królczyk and C. H. Le, J. Alloys Compd., 2020, 824, 153774 CrossRef CAS.
- P. Aprile, D. Letourneur and T. Simon-Yarza, Adv. Healthcare Mater., 2020, 9, 2000707 CrossRef CAS.
- D. Xu, Y. Wan, Z. Li, C. Wang, Q. Zou, C. Du and Y. Wang, Biomater. Sci., 2020, 8, 3286–3300 RSC.
- P. H. Oliveira, L. A. B. Santana, N. S. Ferreira, S. Sharifi-Asl, T. Shokuhfar, R. Shahbazian-Yassar, G. M. L. Dalmonico, J. Werckmann, M. Farina and E. A. dos Santos, Ceram. Int., 2020, 46, 10585–10597 CrossRef CAS.
- M. Y. Zakaria, M. I. Ramli, A. Sulong, N. Muhamad and M. H. Ismail, J. Mater. Res. Technol., 2021, 12, 478–486 CrossRef CAS.
- J. Nie, J. Zhou, X. Huan, L. Wang, G. Liu and J. Cheng, Ceram. Int., 2019, 45, 13647–13655 CrossRef CAS.
- T. Yi, C. Zhou, L. Ma, L. Wu, X. Xu, L. Gu, Y. Fan, G. Xian, H. Fan and X. Zhang, J. Tissue Eng. Regener. Med., 2020, 14, 486–496 CrossRef CAS PubMed.
- Q. Peng, X. Bin, H. Pan, Y. Wang, Z. Peng and Z. Tang, ACS Omega, 2020, 5, 11629–11636 CrossRef CAS PubMed.
- F. Zhang, S. Cai, G. Xu, F. Wang, N. Yu, R. Ling and X. Wu, Ceram. Int., 2016, 42, 18466–18473 CrossRef CAS.
- Q. Du, F. Wei, S. Wang, S. Cheng, Y. Wang, B. Li, D. Jia and Y. Zhou, Appl. Surf. Sci., 2019, 487, 708–718 CrossRef CAS.
- D. Wei, Q. Du, S. Wang, S. Cheng, Y. Wang, B. Li, D. Jia and Y. Zhou, ACS Biomater. Sci. Eng., 2019, 5, 4244–4262 CrossRef CAS PubMed.
- M. T. Choy, K. W. Yeung, L. Chen, C. Y. Tang, G. C. P. Tsui and W. C. Law, Surf. Coat. Technol., 2017, 330, 92–101 CrossRef CAS.
- Z. Peng and J. Hwang, Int. Mater. Rev., 2015, 60, 30–63 CrossRef CAS.
- P. Sikder, N. Koju, Y. Ren, V. K. Goel, T. Phares, B. Lin and S. B. Bhaduri, Surf. Coat. Technol., 2018, 342, 105–116 CrossRef CAS.
- C. Manière, G. Lee, T. Zahrah and E. A. Olevsky, Acta Mater., 2018, 147, 24–34 CrossRef.
- H. Li, Z. Zhao, C. Xiouras, G. D. Stefanidis, X. Li and X. Gao, Renewable Sustainable Energy Rev., 2019, 114, 109316 CrossRef CAS.
- S. Luo, M. Yan, G. B. Schaffer and M. Qia, Metall. Mater. Trans. A, 2011, 42, 2466–2474 CrossRef CAS.
- N. Clar, N. Jones and A. Porch, Sens. Actuators, A, 2017, 259, 137–143 CrossRef.
- F. Xu, Y. Xiao, X. Hu, B. Dong, W. Liu and Y. Li, J. Instrum., 2016, 11, C02074 CrossRef.
- K. I. Rybakov and M. N. Buyanova, Scr. Mater., 2018, 149, 108–111 CrossRef CAS.
- K. I. Rybakov and I. I. Volkovskaya, Ceram. Int., 2019, 45, 9567–9572 CrossRef CAS.
- H. Tang, Z. Peng, F. Gu, L. Ye, J. Hwang, M. Rao, G. Li and T. Jiang, Ceram. Int., 2020, 46, 6828–6837 CrossRef CAS.
- Y. Zhang, D. K. Agrawal, J. Cheng and T. Slawecki, IEEE Trans. Microwave Theory Tech., 2018, 66, 2107–2115 Search PubMed.
- S. Raynova, M. A. Imam, F. Yang and L. Bolzoni, J. Manuf. Process., 2019, 39, 52–57 CrossRef.
- R. Augustine, U. G. Kalappura and K. T. Mathew, Microw. Opt. Technol. Lett., 2008, 50, 2931–2934 CrossRef.
- S. Luo, C. Guan, Y. Yang, G. B. Schaffer and M. Qian, Metall. Mater. Trans. A, 2013, 44, 1842–1851 CrossRef CAS.
- D. S. Seo, K. H. Hwang and J. K. Lee, J. Nanosci. Nanotechnol., 2008, 8, 944–948 CrossRef CAS PubMed.
- R. B. Heimann, Surf. Coat. Technol., 2016, 301, 1–5 CrossRef CAS.
- B. Liu, W. Yu, G. Xiao, C. Chen and Y. Lu, Surf. Coat. Technol., 2021, 413, 127093 CrossRef CAS.
- E. Palierse, C. Helary, J. M. Krafft, I. Genois, S. Masse, G. Laurent, M. I. A. Echazu, M. Selmane, S. Casale, L. Valentin, A. Miche, B. C. L. Chan, C. B. S. Lau, M. Ip, M. F. Desimone, T. Coradin and C. Jolivalt, Mater. Sci. Eng., C, 2021, 118, 111537 CrossRef CAS PubMed.
- S. Panda, B. P. Behera, S. K. Bhutia, C. K. Biswas and S. Paul, J. Alloys Compd., 2022, 918, 165662 CrossRef CAS.
- K. T. Arul, J. R. Ramya, K. R. Karthikeyan and S. N. Kalkura, Mater. Lett., 2014, 135, 191–194 CrossRef CAS.
- S. Shen, S. Cai, X. Bao, P. Xu, Y. Li, S. Jiang, S. Jiang and G. Xu, Chem. Eng. J., 2018, 339, 7–13 CrossRef CAS.
- A. Jaafar, C. Hecker, P. Arki and Y. Joseph, Bioengineering, 2020, 7, 127 CrossRef CAS PubMed.
- R. kohler, K. Sowards and H. Medina, J. Manuf. Process., 2020, 59, 113–121 CrossRef.
- J. Xu, J. Zhang, Y. Shi, J. Tang, D. Huang, M. Yan and M. S. Dargusch, Materials, 2022, 15, 1749 CrossRef CAS.
- Q. Du, D. Wei, S. Cheng, Y. Wang, B. Li, D. Jia and Y. Zhou, Appl. Surf. Sci., 2021, 539, 148153 CrossRef CAS.
- H. Zhou, S. Kong, Y. Pan, Z. Zhang and L. Deng, Mater. Sci. Eng., C, 2015, 56, 174–180 CrossRef CAS PubMed.
- P. Keikhosravani, H. Maleki-Ghaleh, A. K. Khosrowshahi, M. Bodaghi, Z. Dargahi, M. Kavanlouei, P. Khademi-Azandehi, A. Fallah, Y. Beygi-Khosrowshahi and M. H. Siadati, Int. J. Mol. Sci., 2021, 22, 9214 CrossRef CAS.
- P. Sikder, C. R. Grice, B. Lin, V. K. Goel and S. B. Bhaduri, ACS Biomater. Sci. Eng., 2018, 4, 2767–2783 CrossRef CAS.
- C. Wetzel, M. Lonneman and C. Wu, Eur. J. Med. Chem., 2021, 209, 112931 CrossRef CAS PubMed.
- A. S. Khan and M. Awais, Coatings, 2020, 10, 090880 Search PubMed.
- F. B. Simões, L. M. da Silva, T. O. Fonseca, L. E. Almeida and E. A. dos Santos, Ceram. Int., 2018, 44, 16960–16971 CrossRef.
- J. Cizek, V. Brozek, T. Chraska, F. Lukac, J. Medricky, R. Musalek, T. Tesar, F. Siska, Z. Antos, J. Cupera, M. Matejkova, Z. Spotz, S. Houdkova and M. Kverka, J. Therm. Spray Technol., 2018, 27, 1333–1343 CrossRef CAS.
- G. Zhu, Q. Peng, T. Luo, H. Pan, Y. Wang and Z. Peng, Materials, 2022, 15, 6206 CrossRef CAS PubMed.
- Y. Ergün and M. S. Başpınar, Int. J. Hydrogen Energy, 2017, 42, 20420–20429 CrossRef.
- A. T. Guner and C. Meran, Int. J. Surf. Sci. Eng., 2019, 13, 237–262 CrossRef CAS.
- V. Muller, T. Pagnier, S. Tadier, L. Gremillard, M. Jobbagy and E. Djurado, Appl. Surf. Sci., 2021, 541, 148162 CrossRef.
- L. Xia, Y. Xie, B. Fang, X. Wang and K. Lin, Chem. Eng. J., 2018, 347, 711–720 CrossRef CAS.
- Y. Su, K. Li, J. Wang, W. Xie, X. Zhu and C. Wang, Composites, Part B, 2019, 176, 107205 CrossRef CAS.
- W. S. W. Harun, R. I. M. Asri, J. Alias, F. H. Zulkifli, K. Kadirgama, S. A. C. Ghani and J. H. M. Shariffuddin, Ceram. Int., 2018, 44, 1250–1268 CrossRef CAS.
- M. Qadir, Y. Li, K. Munir and C. Wen, Crit. Rev. Solid State Mater. Sci., 2018, 43, 392–416 CrossRef CAS.
- L. Chen, J. Ren, N. Hu, Q. Du and D. Wei, RSC Adv., 2021, 11, 7305–7317 RSC.
- N. V. Bulina, S. V. Makarova, S. G. Baev, A. A. Matvienko, K. B. Gerasimov, O. A. Logutenko and V. S. Bystrov, Minerals, 2021, 11, 1310 CrossRef CAS.
- A. Tampieri, A. Ruffini, A. Ballardini, M. Montesi, S. Panseri, F. Salamanna, M. Fini and S. Sprio, Biomater. Sci., 2019, 7, 307–321 RSC.
- Q. Peng, X. Bin, H. Xuxing, Y. Wang, Z. Peng and Z. Tang, J. Alloys Compd., 2020, 825, 153102 CrossRef CAS.
- H. Yao, C. Yang, Q. Yang, X. Hu, M. Zhang, X. Bai, H. Wang and Q. Chen, Mater. Chem. Phys., 2020, 241, 122340 CrossRef CAS.
- J. Xu, J. Zhang, L. Bao, T. Lai, J. Luo and Y. Zheng, Sci. China Mater., 2017, 61, 545–556 CrossRef.
- S. Tao, J. Xu, L. Yuan, J. Luo and Y. Zheng, J. Alloys Compd., 2020, 812, 152142 CrossRef CAS.
- C. Prakash, S. Singh, A. Basak, G. Krolczyk, A. Pramanik, L. Lamberti and C. I. Pruncu, J. Mater. Res. Technol., 2020, 9, 242–252 CrossRef CAS.
- L. Zhang, Z. He, Y. Zhang, Y. Jiang and R. Zhou, Mater. Sci. Eng., C, 2016, 67, 104–114 CrossRef CAS PubMed.
- M. T. Choy, C. Y. Tang, L. Chen, W. C. Law, C. P. Tsui and W. W. Lu, Composites, Part B, 2015, 83, 50–57 CrossRef CAS.
- T. K. Jung, H. Matsumoto, T. Abumiya, N. Masahashi, M. S. Kim and S. Hanada, Mater. Sci. Forum, 2010, 631, 205–210 Search PubMed.
- B. H. K. Lopes, R. C. Portes, M. A. do Amaral, D. E. Florez-Vergara, A. M. Gama, V. A. Silva, S. F. Quirino and M. R. Baldan, J. Mater. Res. Technol., 2020, 9, 2369–2375 CrossRef CAS.
- S. Pramanik, R. Konwarh, N. Barua, A. K. Buragohain and N. Karak, Biomater. Sci., 2014, 2, 192–202 RSC.
- M. T. Choy, C. Y. Tang, L. Chen, C. T. Wong and C. P. Tsui, Mater. Sci. Eng., C, 2014, 42, 746–756 CrossRef CAS PubMed.
- C. Y. Tang, C. T. Wong, L. N. Zhang, M. T. Choy, T. W. Chow, K. C. Chan, T. M. Yue and Q. Chen, J. Alloys Compd., 2013, 557, 67–72 CrossRef CAS.
- Q. Peng, Z. Tang, Y. Wang and Z. Peng, Ceram. Int., 2019, 45, 24684–24690 CrossRef CAS.
- Z. Ding, Q. Peng, J. Zuo, Y. Wang, H. Zhou and Z. Tang, Materials, 2022, 15, 4985 CrossRef CAS PubMed.
- S. Christe, J. C. G. E. da Silva and L. P. da Silva, Materials, 2020, 13, 504 CrossRef CAS PubMed.
- M. Wu, T. Wang, J. Zhang, H. Qian, R. Miao and X. Yang, Mater. Lett., 2017, 206, 56–59 CrossRef CAS.
- J. Zhang, T. Wang, C. Tang, Q. Wang, H. Qian and R. Miao, J. Inorg. Mater., 2017, 32, 1264–1268 CrossRef.
- T. Huang, H. Wang, Z. Zhang, K. Feng and L. Xiang, Biomater. Sci., 2022, 10, 6656–6674 RSC.
- Q. Feng, X. Wu, Y. Guo, J. She and Y. Xiang, Mater. Trans., 2018, 59, 922–926 CrossRef CAS.
- Y. Torres, B. Begines, A. M. Beltran and A. R. Boccaccini, Surf. Coat. Technol., 2021, 421, 127366 CrossRef CAS.
- B. Liu and X. Wang, Powder Technol., 2022, 396, 754–764 CrossRef CAS.
- M. R. Akbarpour, H. M. Mirabad, A. Hemmat and H. S. Kim, Prog. Mater. Sci., 2022, 127, 100933 CrossRef CAS.
- P. Zhang, C. An, J. Mu, F. Cui, W. Cheng, B. Ye and J. Wang, J. Mater. Res., 2022, 37, 2793–2802 CrossRef CAS.
- D. Cai, M. Bao, X. Wang, L. Yang, G. Qin, R. Wang, D. Chen and E. Zhang, Rare Met., 2019, 38, 503–511 CrossRef CAS.
- N. Xu, J. Fu, L. Zhao, P. Chu and K. Huo, Adv. Healthcare Mater., 2020, 9, 2000681 CrossRef CAS PubMed.
- A. Merlo, V. R. S. S. Mokkapati, S. Pandit and I. Mijakovic, Biomater. Sci., 2018, 6, 2298–2311 RSC.
- J. A. Otte, N. Soro, N. Yang, J. Zou and M. S. Dargusch, Mater. Sci. Eng., A, 2022, 845, 143249 CrossRef CAS.
|
This journal is © The Royal Society of Chemistry 2024 |
Click here to see how this site uses Cookies. View our privacy policy here.