DOI:
10.1039/D4BM00224E
(Minireview)
Biomater. Sci., 2024,
12, 6006-6018
Recent advances in nanogels for drug delivery and biomedical applications
Received
12th February 2024
, Accepted 26th June 2024
First published on 1st November 2024
Abstract
Nanotechnology has shown great promise for researchers to develop efficient nanocarriers for better therapy, imaging, and sustained release of drugs. The existing treatments are accompanied by serious toxicity limitations, leading to severe side effects, multiple drug resistance, and off-target activity. In this regard, nanogels have garnered significant attention for their multi-functional role combining advanced therapeutics with imaging in a single platform. Nanogels can be functionalized to target specific tissues which can improve the efficiency of drug delivery and other challenges associated with the existing nanocarriers. Translation of nanogel technology requires more exploration towards stability and enhanced efficiency. In this review, we present the advances and challenges related to nanogels for cancer therapy, ophthalmology, neurological disorders, tuberculosis, wound healing, and anti-viral applications. A perspective on recent research trends of nanogels for translation to clinics is also discussed.
1. Introduction
Despite the continuous efforts of nanotechnologists worldwide, nanocarriers still need to be wholly accepted in the clinical world due to their biosafety concerns. The utmost need in nanomedicine is the development of safe and biodegradable nanocarriers suitable for the biological and intracellular microenvironment without inducing side effects or immune responses. The sustained and targeted delivery of drugs utilizing nanocarriers has raised hope for better therapeutics and enhanced efficacy. In recent years, nanogel technology has witnessed significant advancements, with the development of nanomaterials exhibiting multifunctional imaging features and delivery of therapeutics for various diseases. In this regard, micro/nanogels that are soft tissue-like cross-linked hydrogel particles composed of polymeric chains have gained much attention.1,2 These nanocarriers possess unique, enhanced characteristics that make them ideal drug delivery systems. Nanogels have very high stability in physiological solutions and are non-toxic to the tissue microenvironment. Owing to their high strength and resemblance to human tissues, they can survive the shear forces and the presence of serum proteins in the bloodstream.3 Nanogels offer unique advantages over other carriers across the highly selective blood–brain barrier (BBB) for brain targeting and contributing to a higher surface-to-volume ratio, responsible for enhanced interaction with the environment and uniform distribution at the intended location for functionalization and bioconjugation with various ligands, bioactives, and DNA or RNA. Because of their porous structure and hydrophobic modifications that can restrict their swelling ability, nano-sized biomaterials can be excellent candidates as drug carrier systems, allowing a higher load of pharmaceutical agents to be incorporated within the 3D polymer matrix while protecting these from physiological degradation.
It is possible that nanogels can be modified to display lipophilic or hydrophilic properties, encapsulating drugs of varying affinities while interacting accordingly with the target tissues and cell membranes.4 Smart nanogels can be designed to react to external stimuli such as near-infrared (NIR) light,5 pH,6 temperature,7 and pressure.8 Recently, nanogels have been modified by adding nanofillers, forming composite materials with diverse, multifunctional characteristics. For example, carbon nanotubes,9 fullerenes,10 magnetic nanoparticles11,12 and plasmonics13 are added to nanogels to amplify their applications in image-guided therapy and on-demand drug release.14,15Table 1 summarizes the recent nanogel technology-based systems showing their multifunctional features useful in various diseases. In this article, we attempt to briefly discuss the advancements in nanogels specific to neurological disorders, cancer therapy, and anti-viral applications, among other settings. The prospects for their clinical application are also discussed briefly.
Table 1 Recent advancements in nanogels used for various diseases
Nanogel matrix materials |
Synthesis method |
Imaging agent |
Therapeutic delivered |
Advantages |
Disease |
Ref. |
Polysulfamide-based (poly(2-((2-(methacryloyloxy)ethyl)dimethylammonio)acetyl)(phenylsulfonyl)amide (PMEDAPA)) nanogels |
Aqueous precipitation polymerization |
Fluorescent dye Cy5 is modified PMEDAPA nanogels are used to visualize the behaviors of nanogels in vitro and in vivo |
Doxorubicin hydrochloride (DOX) |
On-demand drug release and microwave heating for improved cancer therapy |
Cancer therapy |
16–19
|
Dual enzyme lactate oxidase (LOD) and catalase (CAT) encapsulated into the self-assembled nanogels to fabricate responsive nanoprobe LOD/CAT-loaded nanogels (LCNGs). |
Supramolecular chemistry |
Enzyme substrates |
DOX |
Promising theranostic tool to target high lactate and hydrogen peroxide for ultrasound imaging (US) and cancer treatment |
Gold NGs (GAuNGs) composed of thermoresponsive linear poly(glycidyl ether) (tPG) chains cross-linked by small AuNPs. |
Thermo-nanoprecipitation |
NIR absorption capable GAuNGs |
DOX |
Enhancing the antitumoral effect of loaded drugs in the system |
Near infrared (NIR)-responsive CuS-loaded nanogels |
Reflux-precipitation polymerization |
CuS/Cy-7 |
DOX |
Combined with strong photothermal and chemotherapy effects. |
Zwitterionic nanogels based on poly(sulfobetaine methacrylate) (PSBMA) |
One-step reflux-precipitation polymerization |
— |
Levofloxacin (LEV) |
Surfactant-free and morphology controlled, extended drug delivery |
Ophthalmology applications |
20 and 21 |
Penetratin-complexed, redox-responsive hyaluronic acid-based nanogels |
EDC coupling protocol |
Carbon dots (CD) |
9-cis-Retinal |
First attempt to deliver the visual chromophore topically |
NIR activable biomimetic ARNGs@TMZ/ICG nanogels |
Cross-linking by pullulan and poly(deca-4,6-diynedioic acid) (PDDA) and loaded with temozolomide and indocyanine green (ICG) |
Green fluorescence labelled CD31 antibody used to observed penetration of nanogels into the tumor |
Temozolomide and indocyanine green (ICG) |
Suppresses the tumor growth in orthotopic GBM and GBM |
Neurological disorders |
22 and 23 |
A jelly formulation containing M. pruriens seed-extract nanogel |
Ionic gelation |
— |
Levodopa (L-dopa) |
Natural L-dopa from M. pruriens seed extract with the prominent antioxidant activity |
Sulfated nanogels (NGs) based on dendritic polyglycerol sulfate (dPGS) |
Inverse nanoprecipitation technique based on a bio-orthogonal click reaction. |
— |
Potent inhibitors for HS-binding viruses |
Nanogels are non-toxic and broad-spectrum, can multivalently interact with viral glycoproteins, shield virus surfaces, and efficiently block infection |
Anti-viral applications |
24–26
|
Sodium alginate and ammonium sulfite mixture forms carbonized nanogels (Alg@AS CNGs). |
One-step pyrolysis |
— |
Inhibit H1N1 infection |
Superior anti-viral, antioxidative, and anti-inflammatory activities. |
Poly(N-isopropylacrylamide) (polyNIPAm) nanogels |
Dispersion polymerisation |
— |
Lopinavir |
Method for tuning the release of lopinavir from in situ loading implants with high drug loadings. |
2. Nanogels for neurological disorders
Brain health is one of the essential parameters for the humankind. A 2016 report by WHO noted that epilepsy affects 50 million people globally, while 47.5 million individuals were affected by some form of dementia. Alzheimer's disease (AD) contributes to 60–70% of neurological disorders. Other standard neurological diseases include Parkinson's disease (PD), migraines, and multiple sclerosis.27 Treatment limitations include inadequate delivery systems, aging, systemic side effects, and primarily the BBB, that allows the passage of mainly small, hydrophobic molecules. The complexity of the CNS poses a significant challenge in disease treatment. Different cell types with detailed mechanisms contribute to the difficulty in the development of effective therapeutics that selectively target neuronal tissue. Specifically, the strict selectivity limits therapeutic agents from reaching their target location in the brain at effective concentrations sufficient for desirable results.28
Moreover, the agents that can cross the BBB are further challenged by efflux transporters, which prevent the accumulation of the drug in the brain and continue to hinder their efficacy.29 Owing to these limitations, more invasive treatment forms have been sought, posing significant risks for patients and challenging treatment practicality.30 Nanoscale systems, possessing advantageous size and modifiable characteristics, are a candidate with easy access through the neuroprotective barrier. Many therapeutic agents such as nucleic acids, proteins, polypeptides, and anti-sense drugs are incorporated in nanogels and used for neurological disorders.31 A lipopeptide nanoparticle was loaded with siRNA to target genetic disorders via gene silencing. In non-human primates, over 95% of the genes were silenced with a small dose of 0.3 mg kg−1 of the nanoparticle, which was significantly lower than the dose required from endothelial or immune cells to achieve similar effects. Moreover, the nanoparticle was well tolerated at doses up to 1 mg kg−1, 100 times the effective dose in rat models.32 Nanogels possess the characteristics required to carry the bioactive molecules and cross the BBB.33 An example is surface modification. Specific ligands may be added to the surface of nanogels for interaction with receptors, allowing for the nanogel’
s internalization via receptor-mediated transcytosis. Nanogels may also be hydrophobically modified with coatings that mimic the lipid bilayer, facilitating the integration between the two.34 A crucial property of an efficient nanogel carrier is its prolonged blood circulation inside the human body, which results in efficient accumulation in target tissues.35 This can be accomplished by synthesizing hydrogels encompassing liposomes, zwitterionic polymers, or polyethylene glycol (PEG), which increase biocompatibility and evade recognition by the immune system.36 Additionally, formulating nanogels with a lipid bilayer allows them to blend with membrane lipids of other cells, contributing to the possible evasion of efflux transporters. Molecules such as polyethylene glycol (PEG) can hinder the interaction between the nanogel and the transporter, reducing the amount of nanogel that is pumped out of the cell and increasing the overall intercellular concentration.37 A glycyrrhizic acid-zinc alginate nanogel loaded with albiflorin, an anti-inflammatory and antioxidant, resulted in the selective biodistribution of the drug and its accumulation at the target site in significantly higher loads than when compared to free drugs.38 To treat epilepsy, a sodium sulfonate nanogel loaded with phenytoin (PHT) and α-methyl-L-tryptophan successfully targeted the epileptic foci and released an anti-epileptic drug during an elevation in the reactive oxygen species (ROS) levels. Moreover, the nanogel inhibited hyperactive neural circuits (Fig. 1).39 The formulated nanogel highlights the synergistic opportunity to regulate apoptotic lesions.
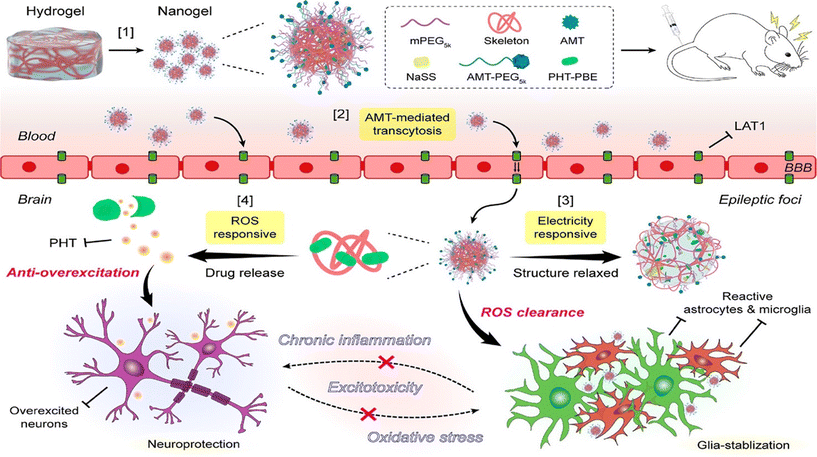 |
| Fig. 1 Structure of the nanogel and its mechanism of targeting epileptic foci and ROS/electricity induced drug release. Copyright under Creative Commons Attribution 4.0 from ref. 39. | |
Additionally, testing of ischemic stroke using glycol-conjugated urokinase nanogels protected the BBB, reducing severity and neurotoxicity at significant rates.40,41 The role of nanogels as diagnostic tools for brain diseases has also been researched. Gelatin nanogels loaded with gadolinium allow for excellent MRI contrast and can be quickly excreted via the renal system. These do not migrate across the BBB, a favorable property for the contrast agent to spare possible contamination with the brain parenchyma or cerebrospinal fluid (CSF), reducing the risk of gadolinium brain deposition.42
3. Nanogels for ophthalmology
Current treatment modalities for ophthalmic diseases are challenged by factors such as poor drug bioavailability, which can result from the quick drainage of traditional eye drops from the nasolacrimal system or tear turnover.43 Similarly, penetration barriers such as the corneal epithelium and blood-retinal barrier prevent drugs from reaching the deep ocular tissue.44 The short residence time of traditional eye treatments also poses a significant challenge to the field and requires frequent administration of eye therapeutics to maintain sufficient levels for desirable results. These factors can burden patients, leading to non-compliance and the non-attainment of optimal treatment results.45 Additionally, ophthalmic formulations can yield suboptimal results as, like many other treatments, targeted delivery is often not achieved.45 In this regard, nanogels are being extensively explored for eye disorders as they effectively entrap the drugs, protect them, and increase the drug residence on the ocular surface while promoting effective penetration in various eye compartments.46 Carbonized nanogels (CNGs) using the pyrolysis of lysine hydrochloride (Lys) have high biocompatibility, anti-inflammatory, and free radical scavenging properties. A single dose of the nanogel (50 μg mL−1) achieved the same therapeutic effect in vivo in less time than prescription cyclosporine eye drops at a concentration ten times higher (500 μg mL−1) (Fig. 2).47 The increased efficacy at lower concentrations indicates greater potency by lowering side effects, and reducing possible toxicities associated with traditional eye therapeutic agents.45 At the same time, this can improve patient compliance as less frequent dosing is required.
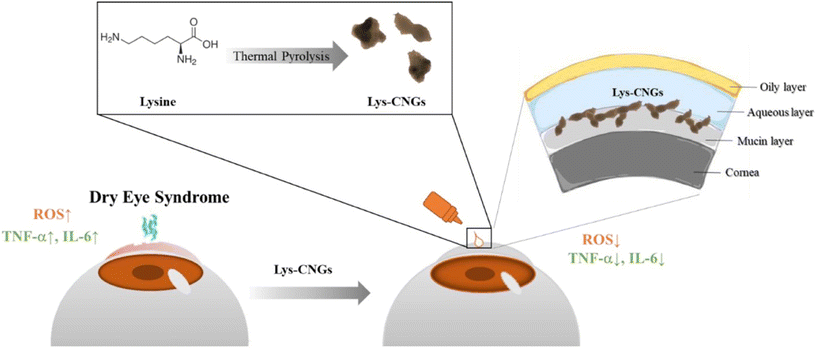 |
| Fig. 2 Schematic representation of the one-step synthesis of lysine-carbonized nanogels (Lys-CNGs) and their application for the treatment of dry eye disease through long-term mucoadhesion on the ocular surface to exert antioxidant and anti-inflammatory effects. Reprinted from ref. 47. Copyright (2022) with permission from Elsevier. | |
Pathological conditions of the cornea, like trauma and allograft rejection, result in increased alternative splicing of the CD44 isotype and increased receptor density.48 Hyaluronic acid (HA), a naturally occurring polysaccharide highly concentrated in joints, eyes, corneal epithelium, and endothelium, is known to interact with the CD44 receptors of cells and has been leveraged in this regard.48,49 A hyaluronan-cholesterol nanogel increased preocular retention of hydrophobic drugs such as dexamethasone and facilitated the permeation of tobramycin, a hydrophilic agent, showing effective encapsulation for treatment and further application in ophthalmology.46 A study showed self-assembled nanogel suspension MC-g-PNtBAm, methylcellulose-grafted-poly(N-tert-butylacrylamide), a drug delivery system for dexamethasone with prospects for ocular drug delivery and loaded drug potentially released for up to 1 week as opposed to 5 minutes for the free drug.50 Existing challenges associated with dexamethasone include but are not limited to poor solubility, which can be overcome by the encapsulation of the drug in the nanogels, which are modified to possess hydrophilic features, ensuring the even distribution of dexamethasone throughout the ocular surface.51,52 The extended-release profile provided by the nanogel encapsulation leads to a sustained release of the therapeutic agent, which can help prevent fluctuations in treatment that are known to yield inflammatory rebound. In this process, inflammation caused by the steroid worsens as the drug is abruptly discontinued.53,54 The sustained release can also contribute to patient compliance by reducing administration times.
Similarly, these nanomaterials can cross the blood–retinal barrier to treat refractive errors via contact lens application for diseases such as cataracts and glaucoma, for which single time usage of timolol-loaded nanogels decreased the intraocular pressure for 48 hours. Less than 5% of conventional timolol eye drops can penetrate the eye to manage glaucoma properly.55 Controlled management of intraocular pressure helps reduce the disease progression rate, which could lead to vision loss if the pressure is not maintained within the normal range over time.56
4. Nanogels for wound healing applications
Nanogels have emerged as a promising nanomaterial for wound healing applications owing to their unique properties. High water content and tunable physical and chemical properties make them perfect candidate for wound healing application. One of the greatest challenges in wound healing is the management of possible infections, which may often involve antibiotic-resistant bacteria. Poor tissue perfusion may also be experienced in chronic wounds, leading to poor healing. An important parameter of wound healing is appropriate tensile strength, which indicates the mechanical stability and integrity of the tissue. A higher value in tissue repair coordinates with tissue less prone to additional injury or to experience wound dehiscence. Enhanced therapeutic outcomes were seen with synthesized silver nano gels, which yielded significantly higher tensile strength compared to the market drug Silverex. This result was achieved by applying silver nanogel at a significantly lower concentration than Silverex.57 Owing to their colloidal stability, nanogels provide an excellent platform for classical topical treatment for wound healing. The retention of water offered by the nanocarrier provides a balanced, moist environment, crucial for optimal cell conduction and proliferation, leading to tissue regeneration. At the same time, nanogels can remove excess moisture from the wound site, promoting biofilm growth or leading to maceration.58,59 Common biopolymers used to develop nanogels for wound healing applications include silk fibroin, alginate, chitosan, collagen, and hyaluronic acid. These biopolymers have intrinsic anti-inflammatory and antibacterial properties, which add to the wound-healing application.60–62 Scarless wound healing is one of the critical clinical challenges, leading to cosmetic concerns. In this regard, nanogels can be leveraged to encapsulate anti-inflammatory agents and growth factors to regulate cell proliferation and migration, collagen modulators to control remodeling, and angiogenic factors to enhance vascularization for optimal tissue repair. Such encapsulation can provide a sustained release of these agents.63 A recent study by Chen et al. reported HA-modified and verteporfin (VP)-loaded polylactic acid nanogel (VP-PLA) for scar-less wound healing (Fig. 3). The nanogel effectively inhibited yes-associated protein (YAP) expression, which is ideal as YAP activity is linked to fibrosis development. Simultaneously, the nanogel promoted the expression of proliferating cell nuclear antigen (PCNA), a cell proliferation marker, while enhancing migratory rates of fibroblasts, both of which contribute to tissue repair. Interestingly, the study demonstrated that the PLA component of the nanogel did not play a direct role in YAP inhibition, indicating that the materials used for the nanogel synthesis were sufficient to achieve the intended results.64 Another innovation in biomaterials used for wound healing involves using Paccai eruval nanogels. These studies reveal enhancement of collagen synthesis and wound healing with the nanogel treatment. The nanogel demonstrated a shorter epithelialization period of up to 14 days, which indicates a faster wound healing rate, lower than the rate obtained under traditional care, which may last up to 3 weeks.65 Antimicrobial silver nanogels have also been tested in wound management, where enhanced wound healing was seen on incision, excision, and burns. The gel successfully inhibited bacterial growth with no adverse effects. This finding suggests that silver nanogel is a candidate for wound dressing, capable of arresting bacterial growth at the surface level and preventing further infection.57 A favorable wound closing rate on histological examination was seen with chitosan-lysate nanogel, deeming it a good candidate for wound healing. The nanogel recovered cisplatin-induced damage to tubular cells, reducing injury from 10% to 1%.66 Moreover, nanogels can also be loaded with anesthetics such as lidocaine, where a loading capacity of 99.9% can be obtained along with 93% drug release with no signs of erythematous skin, demonstrating its potential for continuous therapeutic regimen with no allergic reactions. Additionally, the stability of the product can be maintained for six months, drawing attention to the secureness of nanogels after a considerable shelf life, which leaves room for further enhancement upon formulation.67 Wound healing is limited by biofilm and foreign bodies in wounds, which can contribute to treatment resistance and increased risk of infection.68 Patient non-compliance during wound care may also lead to progress impediment.69 Hence, not only do nanogels offer a great advantage given their drug loading capacity, scaffold function, and customizable properties that allow for the management of various wound conditions, but their nano-scale size alone allows for biofilm penetration with the opportunity for additional modification to yield high bacterial cell selectivity,70 overcoming the existing local challenges as opposed to commonly used treatments such as mupirocin or silver sulfadiazine, which are significantly limited in their antibacterial capacity in the presence of biofilm.71 This characteristic also presents an escape from the requirement for debridement for proper wound management, potentially significantly advancing the healing rate via improved regimen adherence. Thus, by minimizing the instances of wound debridement, nanogels, as an alternative, provide a continuous healing environment to support tissue regeneration in a less demanding, facilitated manner.72
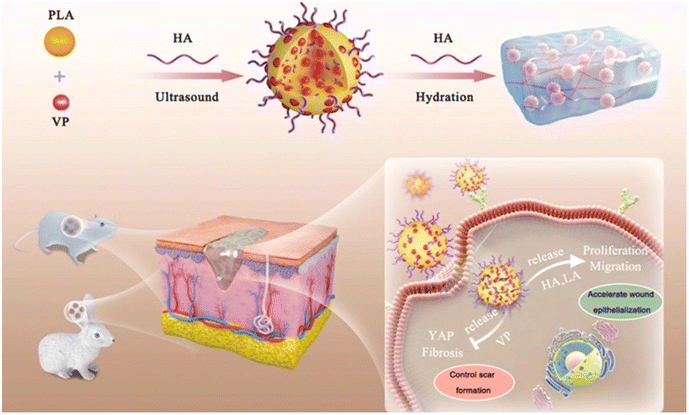 |
| Fig. 3 Schematic illustration of HA/VP-PLA promoting scarless wound healing by accelerating re-epithelization and controlling scar formation. Reprinted with permission from ref. 64. Copyright (2023) Licensed Under a Creative Commons Attribution 4.0 International License. | |
5. Nanogels for tuberculosis
Tuberculosis (TB) is a contagious respiratory disease caused by Mycobacterium tuberculosis (M. Tb). The increase in this disease worldwide is due to drug-resistant strains in TB, the long duration of disease treatment, high dosages of drugs leading to patient non-adherence, and the development of resistant strains that fail the therapy, known as multidrug resistance (MDR) and extensive drug resistance (XDR).73 The common side effects of existing long-term treatment are associated with hepato-toxicity, allergic reactions, gastrointestinal disease, etc.74 Nanomedicine has shown great potential in addressing these limitations. A recent study demonstrated an injectable amphiphilic hydrogel (TB-gel) that can entrap a cocktail of four anti-tubercular drugs, viz., isoniazid, rifampicin, pyrazinamide, and ethambutol. This study showed that by encapsulating the drugs in the nanogel, half of the dose was sufficient to achieve the same results as the free drug.75 Hyaluronic acid-based nanogels have also been explored for TB treatment. A study suggested the potential of LLKKK18 (antimicrobial peptide) loaded into hyaluronic acid nanogels for TB treatment, with the additional effect of reducing proteolytic degradation (Fig. 4). When LLKKK18 was loaded onto the nanogel, the bacterial concentration in the lungs was significantly reduced by 10-fold. Moreover, the concentration of the highly virulent bacterial strain known as M. avium, 25291, was significantly reduced when treated with both the nanogel alone and the loaded nanogel by approximately one-half, suggesting that the properties of the nanogel alone are sufficient to combat bacteria.76 Nanogels can enhance the controlled release of anti-TB drugs and are sensitive to the microenvironment of granuloma and macrophages and pH. Theranostic nanogels that penetrate like a laser to deep tissue and do not damage healthy tissues are needed. The nanogels, which can have efficient ROS scavenging activity, heat generation, and long excitation wavelengths, can effectively target and penetrate the diseased site of the lungs.77 A carboxymethyl chitosan nanogel cross-linked with genipin yielded long-term release of loaded isoniazid and rifampin, both anti-TB drugs, with a concentration of up to 60% in the lungs and displayed a secondary antibacterial effect.78 A similar drug release pattern and antibacterial properties were seen from poly(methacrylic acid) nanogel loaded with rifampin and isoniazid when treating intestinal TB, including slighter cytotoxicity than when administering the drugs freely.79 These findings shed light on the ability of nanogels to treat not only several diseases but the same disease manifesting in different tissues with varying microenvironments, emphasizing the diverse and efficient role of the nanomaterial. Ultimately, the ability of nano gels to deliver drugs precisely and incredibly concentrated with lower doses than free drugs, may guarantee advantages over existing therapeutics that lead to the above-stated resistant strains, which critically hamper disease control and have led to the poor efficacy of TB vaccinations in adults.73 With the inability of treatments to effectively penetrate alveolar macrophages80 and pulmonary barriers for targeted drug delivery, nanogels may prove beneficial in this regard, with electrostatic solid interactions and increased residence time in mucosal surfaces present in the lungs81,82 as well as access to alveolar macrophages due to size and modification of physiochemical parameters.83
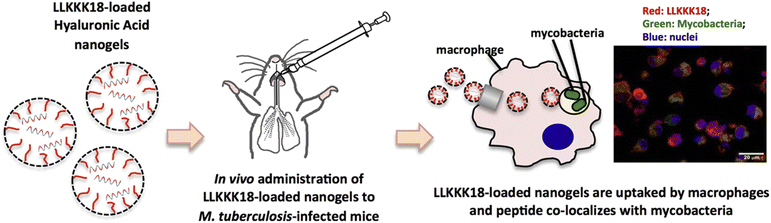 |
| Fig. 4 A lung-targeted inhalational nanogel powder for the sustained release of isoniazid/rifampin against multidrug-resistant Mycobacterium tuberculosis with lower in vivo toxicity. Reprinted from ref. 76. Copyright (2016) with permission from Elsevier. | |
6. Nanogels for anti-viral applications
Over the decades, infectious disease management has been a global challenge for healthcare workers. Continuous efforts have been made to protect and promote human health from the global threat caused by pandemics.84,85 The pandemic caused due to viral infections is the most hazardous and a global burden. Most importantly, the recent outbreak caused by COVID-19 has alarmed researchers worldwide to intesify work on viral diseases.86 In this context, nanomedicine has put forward indispensable technologies for medicine that address precision in therapeutics, offer rapid nano-based testing tools, and targeted interventions for better and large-scale disease management.87–89 Nanogels are deployed to manage severe limitations such as poor lymph node targeting and SARS-CoV-2 spike protein (S-RBD) uptake by antigen-presenting cells, which prevents effective immune responses. A reversible nanogel (S-RBD-NG) was designed with S-RBD protein, which can act as a pro-antigen with enhanced targeting ability for lymph nodes, dendritic cells, and macrophage accumulations.90 A recent study demonstrated the inhibitory effect of poly(N-vinyl caprolactam) nanogels against HIV-1 infection. These nanogels inhibit viral replication in TZM.bl target cells.91 The current emphasis is to develop broad-spectrum anti-viral agents, which will complement strategies involving targeted therapies and can be deployed when an infectious pandemic arises.92 In recent years, nanogel-based therapeutics have shown great potential in drug delivery and imaging applications.24,93 Due to the mandatory parasitic nature, viruses use host cell machinery, making anti-viral strategies more challenging. Non-toxic approaches with minor side effects, a simple dosing schedule, and minimal drug resistance are required. Our group has shown that an autofluorescent nanogel can act as a pre-exposure prophylaxis agent (PrEP) against HIV.94,95 These studies can have a global impact as they are designed using non-toxic, organic materials and straightforward techniques. Thus, nanogels are promising future candidates for anti-viral applications.96,97
7. Nanogels for cancer therapy
Cancer treatments have advanced for patients preferring therapies with negligible side effects. Existing chemotherapeutic agents and current radiation therapies are limited by challenges such as non-selectivity, causing damage to healthy tissues and resulting in severe side effects, poor treatment outcomes, and increased mortality rates in cancer patients.98 Nanogels have high stability and drug encapsulation efficiency. These nanocarriers can potentially deliver the drug directly to the tumor site. Natural biopolymer-based micro/nanogel systems have a structural resemblance to human tissues, biocompatibility, biodegradability, and stability characteristics. Micro/nano gel technology possesses the potential to address the pitfalls of existing therapies due to their improved permeation and high cellular uptake, thus minimizing the toxicity to healthy cells.99 Additionally, the drug-releasing response of nanogels to external stimuli contributes to their massive potential for cancer therapy.100 pH-responsive nanogels use a pH gradient between the tumor tissue microenvironment and normal tissues to release the anti-cancer drug at the tumor site.6 An interesting study showed that injectable immunotherapeutic nanogel could reshape the tumor microenvironment, preventing metastasis and recurrence. It was discovered that pre-exposure to nanoparticles before treatment with a lower radiation dose resulted in significant inhibition of distant tumors compared to lower dose radiation alone, which only minimally inhibited the primary tumor. When antibody anti-CTLA-4 was loaded onto the nanogel in a mouse model, total tumor growth suppression was seen in 40% of the mice. A significantly higher population of CD8+ and CD4+ T cells was also seen in this group.101 This study also demonstrated that immunomodulatory drugs’ local and extended release of over one week from the nanogel could deplete the immunosuppressive cells. With the challenges of drugs accessing the brain because of the BBB, cancers like glioblastoma are difficult to treat. However, phosphorylcholine nanogels have been shown to effectively pass through the BBB given their similarities to the cell membrane, assuring their long-term accumulation in glioblastoma tissue and inhibiting malignant cells with minimal side effects.102 These materials offer a promising future with significantly extended survival rates for patients presenting distal tumor growth.103 Ultimately, these are efficient nanocarriers to target malignant cells and can degrade in safe by-products. Perhaps one of the most explored functions of nanogels in cancer treatment is its ability to improve tumor imaging, allowing for more precise therapy. Here, insulin nanogels with creatinine-functionalized carbon dots, PEGylation, and Herceptin were synthesized for the treatment of HER2-positive breast cancer, revealing successful uptake of the nanogel by cancer cells, increased apoptotic levels, and tumor growth inhibition demonstrated with in vivo mouse models. Moreover, the nanogel showed fluorescence, which allowed for tracking of treatment and disease progress, emphasizing its role as a potential theranostic tool (Fig. 5).104
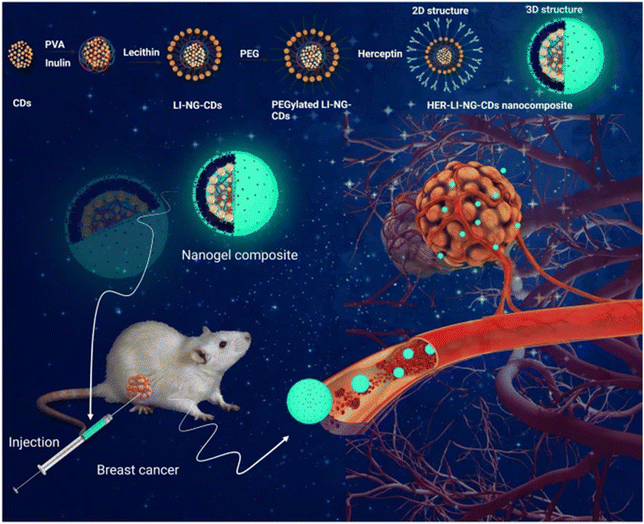 |
| Fig. 5 A multifunctional bioresponsive and fluorescence active nanogel composite for breast cancer therapy and bioimaging. Copyright under Creative Commons Attribution 4.0 from ref. 104. | |
Similarly, PEGylated nanogels containing gold nanoparticles (AuNPs) are being explored for photothermal cancer therapy as researchers found the heat generation feature from AuNPs helpful in selective and non-invasive cancer photothermal therapy, significantly sparing healthy cells.105 Thermoresponsive nanogels are also being developed for multimodal photoacoustic imaging and combinational photothermal therapy. Polymer polypyrrole (PPY) based nanogels have shown long-term stability and water dispersibility, which ensures the proper distribution of the nanogels within the body, which is crucial for consistent therapeutic availability. With their excellent photothermal transducing ability, nanogels can absorb light across specific wavelengths that selectively damage cells by tuning the light source parameters. This means that nanogels could be selectively activated in target tissues. Furthermore, such an advantage opens the way to personalized treatment plans, as therapies can be adjusted depending on the needs of each patient.106 Similarly, these play an essential role in photoacoustic imaging, where locally absorbed light converts into heat for acoustic wave-based imaging with significantly higher contrast. The ability of the nanogel to provide images with higher contrast facilitates the differentiation between healthy and diseased tissues for a more precise analysis. Together, these can allow for a more accurate detection of tumors.107
8. A perspective on recent research trends
It is evident that nanogels possess unique features to be functionalized and are highly biocompatible with the human microenvironment. The stability of nanogels is the most critical parameter, allowing prolonged and sustained drug release. A more exciting hybrid that recently gained attention is the exosome hybrid. Nanogels can act as excellent carriers for exosomes to cells in intact state. Exosomes contain miRNA inside them, released from diseased cells, and can be used as a biomarker for various diseases. Thus, functional exosomes can be delivered inside the cells using nanogels and have a high potential in therapeutics with better efficacy.108 Advancements include the development of magnetic nanogels and exosome hybrids that can be magnetically navigated for intracellular delivery, enabling precise targeting of tumors and minimizing damage to healthy tissue.109
Vascular embolization, commonly used to block tumor-feeding vessels, is challenged by poor intraoperative imaging, which may result in vessel recanalization. A thermosensitive poly(N-isopropyl acrylamide)-co-acrylic acid (NIPAM-co-AA) nanogel was tested in vivo as a real-time imaging embolization material and proved to avoid mis-embolization and eventual recanalization.110
Gene editing therapy utilizing a nanogel system is a new emerging technology with valuable prospects. A fascinating study explored anion-cationic nanogel in which DNA chains were grafted onto polycaprolactone and cross-linked via nucleic acid hybridization technique with DNA linkers. Loading the single guide RNA and Cas9 protein complex (Cas9/sgRNA) onto the nanogel provided a protective delivery method for the gene editing system as it showed stability against enzymatic digestion. Furthermore, the tool is also able to yield higher cellular uptake.111 One of the main challenges in gene editing technology lies in off-target effects. Targeting the incorrect region of a gene may result in the disruption of genes essential to humans, leading to the loss of vital functions. With the precision that nanogels have proved to possess in various studies, gene editing specificity may be achieved, highlighting the importance of the biomaterial being pursued in this regard.112 Features like injectability and self-strengthening enable the sustained release of genes like antagomir-21 (Fig. 6). These reduce local inflammation and play an essential role in maintaining extracellular matrix (ECM) metabolic balance and restoring tissue function, while simultaneously promoting intervertebral disc degeneration (IDD) repair.113 It is likely that the substitution of viral vectors for nanogels for the application of gene therapy and vaccinations may be the response to the immunogenicity concerns of carriers like adenovirus. The attractive concept of a non-viral vector with potentially high efficacy might lead to the amenability of patients to take necessary vaccines, overcoming the hesitance and often resistance from individuals raising concerns about introducing a virus to the body.114,115
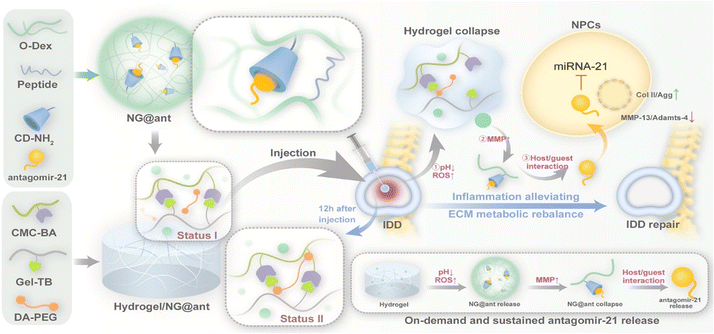 |
| Fig. 6 Illustration of the design of hydrogels, along with their mechanisms which promote intervertebral disc degeneration (IDD) repair. Reprinted from ref. 113. Copyright (2023) with permission from Elsevier. | |
The wound-healing aspect of nanogels has a huge translational application. 3D bioprinting, one of the newer technological advances, has been explored for its tissue engineering capabilities and the advantages it may bring for patients requiring organ transplants, including skin grafts. However, limitations are posed by the materials, referred to as bioink, used to create the 3D models, as these need to be carefully chosen to provide appropriate mechanical strength and durable functionality. A peptide nanogel with a self-assembling sequence forming 3D nanofibrous networks demonstrated that dermal and epidermal cells, fibroblasts, and keratinocytes proliferated effectively within the scaffold and were mitotically active. Moreover, a vascularized skin co-culture was successfully produced from the scaffold.116
9. Challenges and solutions in nanogel technology: overcoming limitations for advanced biomedical applications
The advent of a safe and stable nanogel system must be explored further to address the challenges and limitations of current medicine. However, though nanotechnology holds great promise, many challenges remain to guarantee its application in a clinical setting, such as optimal biocompatibility and low toxicity profile. A careful selection and modification of techniques should be employed to enhance the properties of the nanogels. For instance, zwitterionic hydrogels formed using phosphorylcholine and sulfobetaine can mimic the cellular membrane and reduce nonspecific protein adsorption, ensuring that the nanogels are not aggregated and retain their functionality in the biological fluids, enhancing their compatibility with the body.117 Similarly, the biodegradability of nanogels is of concern when considering their clinical translation. Recently, various cross-linking methods have been tested for this purpose. An example is the incorporation of cleavable linkages which allows the hydrogel to undergo biodegradation at the pH of the cellular environment or under specific enzymatic activities. Once the specific targeted therapy is completed, the linkages in the nanogels are degraded under specific environments to minimize the toxicity. The introduction of cleavable sites allows for the degradation of the nanogel system into smaller, non-toxic fragments for safe clearance,118 a crucial aspect of nanomedicine since the extended presence of nanogels may lead to accumulation in body tissues and trigger inflammatory responses. In this regard, the clearance pathway of nanogels needs to be evaluated in detail. For instance, a particle size limitation exists for renal clearance. Particles with size larger than 8 nm face challenges in being filtered properly throughglomerular capillaries. Furthermore, positively charged particles have demonstrated to have a more rapid filtration rate than their counterparts, which results from the attraction with the negatively charged groups, namely glycosaminoglycans, and proteoglycans, on the glomerular basement membrane.119,120 The materials used for nanogel synthesis should be considered carefully, as these may activate immune responses, leading to complications such as tissue damage or systemic toxicities.121 Additionally, nanogels may undergo alterations in their physical or chemical compositions due to storage, which can result in reduced therapeutic efficacy. Hence, techniques that can improve the shelf-life of nanogels have been gaining much attention in recent years. Lyophilization of nanogels, for instance, has been shown to optimize the stability of nanogels long-term by removing excess water from the nanogel, which could otherwise lead to microbial growth or degradation by hydrolysis or oxidation.122,123 Similarly, the reproducibility of nanogels and their scalability may also raise concerns about their prolonged clinical use. A homogeneous dispersion of colloidal lignin particles (CLPs) was continuously formed using static mixers, resulting in high CLP yields of up to 95%, highlighting its industrial application.124 Research on nanotechnological applications for medicine has greatly advanced, and the delivery of the nanogels into the body in a safe and efficient manner should continue to be pursued.
Data availability
No primary research results, software or code have been included and no new data was generated or analysed as part of this review.
Conflicts of interest
This work has been granted the U.S. Patent on Micro/Nano Magnetic Hydrogels with Autofluorescence for Therapeutic and Diagnostic Applications US Patent App. 15/907, 703, 2019, with Arti Vashist and Madhavan Nair as inventors.
Acknowledgements
AA and MN are grateful for support from the National Institutes of Health (NIH) grants DA042706, DA052271, and DA034547. NK thanks the H&N Wertheim Research Pilot Project from Florida International University (FIU) for the support, and AV is thankful for help from the FIU Foundation via grant number AWD0011094. Arti Vashist is also thankful for the support from the Young Investigator Pilot Award (YIPA) by AIDS and Cancer Specimen Resources sponsored by the National Cancer Institute (NCI).
References
- J. K. Oh, R. Drumright, D. J. Siegwart and K. Matyjaszewski, Prog. Polym. Sci., 2008, 33, 448–477 Search PubMed.
- Y. Yang, T. Zhao, Q. Chen, Y. Li, Z. Xiao, Y. Xiang, B. Wang, Y. Qiu, S. Tu and Y. Jiang, Adv. Sci., 2022, 9, 2202797 Search PubMed.
- X. Ma, S.-J. Li, Y. Liu, T. Zhang, P. Xue, Y. Kang, Z.-J. Sun and Z. Xu, Chem. Soc. Rev., 2022, 51, 5136–5174 Search PubMed.
- I. Neamtu, A. G. Rusu, A. Diaconu, L. E. Nita and A. P. Chiriac, Drug Delivery, 2017, 24, 539–557 CrossRef CAS PubMed.
- L. Yu, A. Dong, R. Guo, M. Yang, L. Deng and J. Zhang, ACS Biomater. Sci. Eng., 2018, 4, 2424–2434 Search PubMed.
- Z. Li, J. Huang and J. Wu, Biomater. Sci., 2021, 9, 574–589 Search PubMed.
- D. Shi, H. Zhang, H. Zhang, L. Li, S. Li, Y. Zhao, C. Zheng, G. Nie and X. Yang, Chem. Eng. J., 2022, 433, 134357 Search PubMed.
- S. Hajebi, N. Rabiee, M. Bagherzadeh, S. Ahmadi, M. Rabiee, H. Roghani-Mamaqani, M. Tahriri, L. Tayebi and M. R. Hamblin, Acta Biomater., 2019, 92, 1–18 CrossRef CAS PubMed.
- A. Seyfoori, S. Sarfarazijami and S. Seyyed Ebrahimi, Artif. Cells, Nanomed., Biotechnol., 2019, 47, 1437–1443 Search PubMed.
- X. Hong, X. Xu, Z. Liu, S. Liu, J. Yu, M. Wu, Y. Ma and Q. Shuai, Nanotechnology, 2021, 32, 465701 CrossRef CAS PubMed.
- Y. Zou, D. Li, Y. Wang, Z. Ouyang, Y. Peng, H. Tomás, J. Xia, J. Rodrigues, M. Shen and X. Shi, Bioconjugate Chem., 2020, 31, 907–915 Search PubMed.
- Y. Cao, Z. Mao, Y. He, Y. Kuang, M. Liu, Y. Zhou, Y. Zhang and R. Pei, ACS Appl. Mater. Interfaces, 2020, 12, 26973–26981 Search PubMed.
- F. Howaili, E. Özliseli, B. Küçüktürkmen, S. M. Razavi, M. Sadeghizadeh and J. M. Rosenholm, Front. Chem., 2021, 8, 602941 Search PubMed.
- X. Lan, L. Huo, S. Li, J. Wang and W. Cai, Eur. J. Nucl. Med. Mol. Imaging, 2022, 49, 2455–2461 Search PubMed.
- J. Bai, H. Zhang, Z. Yang, P. Li, B. Liu, D. Li, S. Liang, Q. Wang, Z. Li and J. Zhang, J. Controlled Release, 2022, 352, 673–684 Search PubMed.
- S. Peng, H. Wang, W. Zhao, Y. Xin, Y. Liu, X. Yu, M. Zhan, S. Shen and L. Lu, Adv. Funct. Mater., 2020, 30, 2001832 CrossRef CAS.
- Q. Wu, Q. Zhang, T. Yu, X. Wang, C. Jia, Z. Zhao and J. Zhao, ACS Appl. Bio Mater., 2021, 4, 4244–4253 CrossRef CAS PubMed.
- J. Bergueiro, E. A. Glitscher and M. Calderón, Biomater. Adv., 2022, 137, 212842 CrossRef CAS PubMed.
- Z. Zhang, X. Zhang, Y. Ding, P. Long, J. Guo and C. Wang, Macromol. Biosci., 2019, 19, e1800416 Search PubMed.
- Z. Wang, X. Li, X. Zhang, R. Sheng, Q. Lin, W. Song and L. Hao, Nanomaterials, 2021, 11, 2328 Search PubMed.
- A. M. Laradji, A. V. Kolesnikov, B. B. Karakoçak, V. J. Kefalov and N. Ravi, ACS Omega, 2021, 6, 6172–6184 CrossRef CAS PubMed.
- D. Zhang, S. Tian, Y. Liu, M. Zheng, X. Yang, Y. Zou, B. Shi and L. Luo, Nat. Commun., 2022, 13, 6835 CrossRef CAS PubMed.
- C. Chittasupho, S. Tadtong, S. Vorarat, W. Imaram, S. Athikomkulchai, W. Samee, V. Sareedenchai, T. Thongnopkoon, S. Okonogi and N. Kamkaen, Pharmaceutics, 2022, 14, 1079 CrossRef CAS PubMed.
- P. Dey, T. Bergmann, J. L. Cuellar-Camacho, S. Ehrmann, M. S. Chowdhury, M. Zhang, I. Dahmani, R. Haag and W. Azab, ACS Nano, 2018, 12, 6429–6442 Search PubMed.
- H.-Y. Lin, K.-L. Luo, J.-Y. Mao, C.-J. Lin, C.-Y. Wang, L. Panny, S.-Y. Chen, S.-C. Lin, C.-C. Huang, S. G. Harroun, R. Y. L. Wang and C.-J. Wu, Chem. Eng. J., 2023, 458, 141429 CrossRef CAS.
- A. R. Town, J. Taylor, K. Dawson, E. Niezabitowska, N. M. Elbaz, A. Corker, E. Garcia-Tuñón and T. O. McDonald, J. Mater. Chem. B, 2019, 7, 373–383 RSC.
-
W. H. Organization, Neurological disorders: public health challenges, World Health Organization, 2006 Search PubMed.
- D. Wu, Q. Chen, X. Chen, F. Han, Z. Chen and Y. Wang, Signal Transduction Targeted Ther., 2023, 8, 217 CrossRef PubMed.
- M. Demeule, A. Régina, J. Jodoin, A. Laplante, C. Dagenais, F. Berthelet, A. Moghrabi and R. Béliveau, Vasc. Pharmacol., 2002, 38, 339–348 CrossRef CAS PubMed.
- M. Markowicz-Piasecka, P. Darłak, A. Markiewicz, J. Sikora, S. K. Adla, S. Bagina and K. M. Huttunen, Eur. J. Pharm. Biopharm., 2022, 181, 249–262 CrossRef CAS PubMed.
- J. D. Torres-Vanegas, J. C. Cruz and L. H. Reyes, Pharmaceutics, 2021, 13, 428 CrossRef CAS PubMed.
- Y. Dong, K. T. Love, J. R. Dorkin, S. Sirirungruang, Y. Zhang, D. Chen, R. L. Bogorad, H. Yin, Y. Chen and A. J. Vegas, Proc. Natl. Acad. Sci. U. S. A., 2014, 111, 3955–3960 CrossRef CAS PubMed.
- Y. Zhang, Z. Zou, S. Liu, S. Miao and H. Liu, Front. Bioeng. Biotechnol., 2022, 10, 954470 CrossRef PubMed.
- V. Manimaran, R. Nivetha, T. Tamilanban, J. Narayanan, S. Vetriselvan, N. K. Fuloria, S. V. Chinni, M. Sekar, S. Fuloria and L. S. Wong, Front. Mol. Biosci., 2023, 10 Search PubMed.
- D. Eckmann, R. Composto, A. Tsourkas and V. Muzykantov, J. Mater. Chem. B, 2014, 2, 8085–8097 RSC.
- P. Eslami, F. Rossi and S. Fedeli, Pharmaceutics, 2019, 11, 71 CrossRef CAS PubMed.
- J.-W. Kang, H.-J. Cho, H. J. Lee, H.-E. Jin and H.-J. Maeng, Int. J. Biol. Macromol., 2019, 125, 61–71 CrossRef CAS PubMed.
- Y.-B. Chen, T. Qiao, Y.-Q. Wang, Y.-L. Cui and Q.-S. Wang, Mater. Des., 2022, 219, 110741 CrossRef CAS.
- Z. Zhou, K. Li, Y. Guo, P. Liu, Q. Chen, H. Fan, T. Sun and C. Jiang, ACS Nano, 2023, 17, 7847–7864 CrossRef CAS PubMed.
- D. Nan, H. Jin, D. Yang, W. Yu, J. Jia, Z. Yu, H. Tan, Y. Sun, H. Hao and X. Qu, Transl. Stroke Res., 2021, 12, 844–857 CrossRef CAS PubMed.
- W. Cui, R. Liu, H. Jin, P. Lv, Y. Sun, X. Men, S. Yang, X. Qu, Z. Yang and Y. Huang, J. Controlled Release, 2016, 225, 53–63 CrossRef CAS PubMed.
- A. Kimura, J.-I. Jo, F. Yoshida, Z. Hong, Y. Tabata, A. Sumiyoshi, M. Taguchi and I. Aoki, Acta Biomater., 2021, 125, 290–299 CrossRef CAS PubMed.
- N. H. Bennett, H. R. Chinnery, L. E. Downie, L. J. Hill and L. M. Grover, Adv. Funct. Mater., 2020, 30, 1908476 CrossRef CAS.
- E. Mannermaa, K.-S. Vellonen and A. Urtti, Adv. Drug Delivery Rev., 2006, 58, 1136–1163 CrossRef CAS PubMed.
- P. Agarwal, J. P. Craig and I. D. Rupenthal, Pharmaceutics, 2021, 13, 207 CrossRef CAS PubMed.
- N. Zoratto, L. Forcina, R. Matassa, L. Mosca, G. Familiari, A. Musarò, M. Mattei, T. Coviello, C. Di Meo and P. Matricardi, Pharmaceutics, 2021, 13, 1781 CrossRef CAS PubMed.
- P.-H. Lin, H.-J. Jian, Y.-J. Li, Y.-F. Huang, A. Anand, C.-C. Huang, H.-J. Lin and J.-Y. Lai, Acta Biomater., 2022, 141, 140–150 Search PubMed.
- M. Guter and M. Breunig, Eur. J. Pharm. Biopharm., 2017, 113, 34–49 CrossRef CAS PubMed.
- F. Bongiovì, G. Di Prima, F. S. Palumbo, M. Licciardi, G. Pitarresi and G. Giammona, Macromol. Biosci., 2017, 17, 1700261 CrossRef PubMed.
- M. Jamard and H. D. Sheardown, Bioengineering, 2018, 5, 39 Search PubMed.
- V. S. Madamsetty, R. Mohammadinejad, I. Uzieliene, N. Nabavi, A. Dehshahri, J. García-Couce, S. Tavakol, S. Moghassemi, A. Dadashzadeh and P. Makvandi, ACS Biomater. Sci. Eng., 2022, 8, 1763–1790 Search PubMed.
- J. Urbańska, A. Karewicz and M. Nowakowska, Life Sci., 2014, 96, 1–6 CrossRef PubMed.
- D. A. Jabs, J. T. Rosenbaum, C. S. Foster, G. N. Holland, G. J. Jaffe, J. S. Louie, R. B. Nussenblatt, E. R. Stiehm, H. Tessler and R. N. Van Gelder, Am. J. Ophthalmol., 2000, 130, 492–513 CrossRef CAS PubMed.
- D. Hazirolan, N. Stübiger and U. Pleyer, Acta Ophthalmol., 2013, 91, 297–306 CrossRef CAS PubMed.
- J. C. Cuggino, L. I. Tártara, L. M. Gugliotta, S. D. Palma and C. I. Alvarez Igarzabal, Mater. Sci. Eng., C, 2021, 118, 111383 CrossRef CAS PubMed.
- M. Li, N. Yuan, X. Chen, Y. Lu, H. Gong, L. Qian, J. Wu, S. Zhang, S. Shipp and I. M. Andolina, EBioMedicine, 2019, 44, 554–562 CrossRef PubMed.
- S. Gaikwad, S. Birla, A. P. Ingle, A. Gade, P. Ingle, P. Golińska and M. Rai, Front. Microbiol., 2022, 13, 881404 CrossRef PubMed.
- C. K. Field and M. D. Kerstein, Am. J. Surg., 1994, 167, S2–S6 CrossRef PubMed.
- B. S. Atiyeh, J. Ioannovich, C. A. Al-Amm and K. A. El-Musa, Curr. Pharm. Biotechnol., 2002, 3, 179–195 Search PubMed.
- H. Nosrati, M. Heydari, Z. Tootiaei, S. Ganjbar and M. Khodaei, J. Drug Delivery Sci. Technol., 2023, 104516 Search PubMed.
- R. Eivazzadeh-Keihan, F. Ganjali, H. A. M. Aliabadi, A. Maleki, S. Pouri, M. Mahdavi, A. E. Shalan and S. Lanceros-Méndez, J. Nanostruct. Chem., 2023, 13, 103–113 CrossRef CAS.
- N. Yuan, K. Shao, S. Huang and C. Chen, Int. J. Biol. Macromol., 2023, 124321 CrossRef CAS PubMed.
- S. Zhou, M. Xie, J. Su, B. Cai, J. Li and K. Zhang, J. Tissue Eng., 2023, 14, 20417314231185848 Search PubMed.
- K. Chen, Y. Liu, X. Liu, Y. Guo, J. Liu, J. Ding, Z. Zhang, X. Ni and Y. Chen, J. Nanobiotechnol., 2023, 21, 241 Search PubMed.
- M. Sakthiganapathi, G. P. Yoganandam and V. Gopal, Pharm. Nanotechnol., 2023, 11, 70–81 CrossRef CAS PubMed.
- Y. Ashoori, M. Mohkam, R. Heidari, S. N. Abootalebi, S. M. Mousavi, S. A. Hashemi, N. Golkar and A. Gholami, BioMed Res. Int., 2020, 2020, 1–9 Search PubMed.
- A. Ali, A. Ali, M. A. Rahman, M. H. Warsi, M. Yusuf and P. Alam, Gels, 2022, 8, 466 CrossRef CAS PubMed.
- P. Monika, M. N. Chandraprabha, A. Rangarajan, P. V. Waiker and K. N. Chidambara Murthy, Front. Nutr., 2022, 8, 791899 CrossRef PubMed.
- C. Moffatt, S. Murray, V. Keeley and A. Aubeeluck, Int. Wound J., 2017, 14, 1305–1312 CrossRef PubMed.
- F. A. Al-Wrafy, A. A. Al-Gheethi, S. K. Ponnusamy, E. A. Noman and S. A. Fattah, Chemosphere, 2022, 288, 132603 CrossRef CAS PubMed.
- K. Razdan, J. Garcia-Lara, V. Sinha and K. K. Singh, Drug Discovery Today, 2022, 27, 2137–2150 CrossRef CAS PubMed.
- M. A. Grimaudo, A. Concheiro and C. Alvarez-Lorenzo, J. Controlled Release, 2019, 313, 148–160 CrossRef CAS PubMed.
- M. Kumar, T. Virmani, G. Kumar, R. Deshmukh, A. Sharma, S. Duarte, P. Brandão and P. Fonte, Pharmaceuticals, 2023, 16, 1360 Search PubMed.
- J. J. Saukkonen, K. Powell and J. A. Jereb, Am. J. Respir. Crit. Care Med., 2012, 185, 598–599 Search PubMed.
- S. Pal, V. Soni, S. Kumar, S. K. Jha, N. Medatwal, K. Rana, P. Yadav, D. Mehta, D. Jain, P. Sharma, R. Kar, A. Srivastava, V. S. Patil, U. Dasgupta, V. K. Nandicoori and A. Bajaj, Nanoscale, 2021, 13, 13225–13230 RSC.
- J. P. Silva, C. Gonçalves, C. Costa, J. Sousa, R. Silva-Gomes, A. G. Castro, J. Pedrosa, R. Appelberg and F. M. Gama, J. Controlled Release, 2016, 235, 112–124 Search PubMed.
- W. Wang, H. Guo, S. Lin, X. Xiao, Y. Liu, Y. Wang and D. Zhou, Biosafety Health, 2022, 4, 258–268 CrossRef.
- T. Wu, W. Liao, W. Wang, J. Zhou, W. Tan, W. Xiang, J. Zhang, L. Guo, T. Chen, D. Ma, W. Yu and X. Cai, Carbohydr. Polym., 2018, 197, 403–413 CrossRef CAS PubMed.
- T. Chen, Q. Li, L. Guo, L. Yu, Z. Li, H. Guo, H. Li, M. Zhao, L. Chen, X. Chen, Q. Zhong, L. Zhou and T. Wu, Mater. Sci. Eng., C, 2016, 58, 659–665 CrossRef CAS PubMed.
- Z. Huang, S. N. Kłodzińska, F. Wan and H. M. Nielsen, Drug Delivery Transl. Res., 2021, 11, 1634–1654 CrossRef CAS PubMed.
- J. Wang, M. Viola, C. Migliorini, L. Paoletti, S. Arpicco, C. Di Meo and P. Matricardi, Pharmaceutics, 2023, 15, 2508 CrossRef CAS PubMed.
- J. C. Cuggino, E. R. O. Blanco, L. M. Gugliotta, C. I. A. Igarzabal and M. Calderón, J. Controlled Release, 2019, 307, 221–246 CrossRef CAS PubMed.
- W.-H. Lee, C.-Y. Loo, D. Traini and P. M. Young, Expert Opin. Drug Delivery, 2015, 12, 1009–1026 CrossRef CAS PubMed.
- V. Pereira, Y. Temouri, S. Patnaik and K. Mellahi, Acad. Manage. Perspect., 2020, 34, 480–492 CrossRef.
- M. L. Cohen, Nature, 2000, 406, 762–767 CrossRef CAS PubMed.
- E. Nicastri, N. Petrosillo, T. Ascoli Bartoli, L. Lepore, A. Mondi, F. Palmieri, G. D'Offizi, L. Marchioni, S. Murachelli, G. Ippolito and A. Antinori, Infect. Dis. Rep., 2020, 12, 3–9 Search PubMed.
- P. Hunziker, Eur. J. Nanomed., 2016, 8, 113–114 CrossRef.
- M. Germain, F. Caputo, S. Metcalfe, G. Tosi, K. Spring, A. K. Åslund, A. Pottier, R. Schiffelers, A. Ceccaldi and R. Schmid, J. Controlled Release, 2020, 326, 164–171 CrossRef CAS PubMed.
- D. Ho, S. R. Quake, E. R. McCabe, W. J. Chng, E. K. Chow, X. Ding, B. D. Gelb, G. S. Ginsburg, J. Hassenstab and C.-M. Ho, Trends Biotechnol., 2020, 38, 497–518 CrossRef CAS PubMed.
- L. Chen, B. Liu, P. Sun, W. Wang, S. Luo, W. Zhang, Y. Yang, Z. Wang, J. Lin and P. R. Chen, Small, 2020, 16, e2004237 CrossRef PubMed.
- M. A. Macchione, C. Guerrero-Beltrán, A. P. Rosso, E. M. Euti, M. Martinelli, M. C. Strumia and M. Á. Muñoz-Fernández, Sci. Rep., 2019, 9, 5732 CrossRef PubMed.
- A. Adalja and T. Inglesby, Expert Rev. Anti–Infect. Ther., 2019, 17, 467–470 CrossRef CAS PubMed.
- L. Chambre, A. Degirmenci, R. Sanyal and A. Sanyal, Bioconjugate Chem., 2018, 29, 1885–1896 CrossRef CAS PubMed.
-
M. Nair, A. Raymond and A. Vashist, US Pat., 17615940, 2002 Search PubMed.
-
A. Vashist, A. Kaushik and N. Madhavan, US Pat., 10344100, 2019 Search PubMed.
- S. A. Ferreira, F. M. Gama and M. Vilanova, Nanomedicine, 2013, 9, 159–173 CrossRef CAS PubMed.
- X. Lv, M. Yuan, Y. Pei, C. Liu, X. Wang, L. Wu, D. Cheng, X. Ma and X. Sun, J. Agric. Food Chem., 2021, 69, 4992–5002 CrossRef CAS PubMed.
- W. M. van den Boogaard, D. S. Komninos and W. P. Vermeij, Cancers, 2022, 14, 627 CrossRef CAS PubMed.
- M. Chehelgerdi, M. Chehelgerdi, O. Q. B. Allela, R. D. C. Pecho, N. Jayasankar, D. P. Rao, T. Thamaraikani, M. Vasanthan, P. Viktor and N. Lakshmaiya, Mol. Cancer, 2023, 22, 169 CrossRef PubMed.
- X. Luo, S. Wu, M. Xiao, H. Gu, H. Zhang, J. Chen, Y. Liu, C. Zhang and J. Zhang, Int. J. Nanomed., 2023, 18, 2589–2621 CrossRef CAS PubMed.
- C. Song, H. Phuengkham, Y. S. Kim, V. V. Dinh, I. Lee, I. W. Shin, H. S. Shin, S. M. Jin, S. H. Um and H. Lee, Nat. Commun., 2019, 10, 1–15 Search PubMed.
- S. Peng, B. Ouyang, Y. Xin, W. Zhao, S. Shen, M. Zhan and L. Lu, Acta Pharm. Sin. B, 2021, 11, 560–571 CrossRef CAS PubMed.
- D. Zhang, S. Tian, Y. Liu, M. Zheng, X. Yang, Y. Zou, B. Shi and L. Luo, Nat. Commun., 2022, 13, 6835 CrossRef CAS PubMed.
- M. Ghomi, E. N. Zare, H. Alidadi, N. Pourreza, A. Sheini, N. Rabiee, V. Mattoli, X. Chen and P. Makvandi, Adv. Compos. Hybrid Mater., 2023, 6, 51 CrossRef CAS.
- T. Nakamura, A. Tamura, H. Murotani, M. Oishi, Y. Jinji, K. Matsuishi and Y. Nagasaki, Nanoscale, 2010, 2, 739–746 RSC.
- L. E. Theune, J. Buchmann, S. Wedepohl, M. Molina, J. Laufer and M. Calderón, J. Controlled Release, 2019, 311–312, 147–161 CrossRef CAS PubMed.
- A. C. V. Doughty, A. R. Hoover, E. Layton, C. K. Murray, E. W. Howard and W. R. Chen, Materials, 2019, 12, 779 CrossRef CAS PubMed.
- S.-I. Sawada, Y. T. Sato, R. Kawasaki, J.-I. Yasuoka, R. Mizuta, Y. Sasaki and K. Akiyoshi, Biomater. Sci., 2020, 8, 619–630 RSC.
- R. Mizuta, Y. Sasaki, R. Kawasaki, K. Katagiri, S.-I. Sawada, S.-A. Mukai and K. Akiyoshi, Bioconjugate Chem., 2019, 30, 2150–2155 CrossRef CAS PubMed.
- H. Zhou, W. Xie, A. Guo, B. Chen, S. Hu, M. Zheng, H. Yu, H. Tian and L. Li, Des. Monomers Polym., 2023, 26, 31–44 CrossRef CAS PubMed.
- F. Ding, X. Huang, X. Gao, M. Xie, G. Pan, Q. Li, J. Song, X. Zhu and C. Zhang, Nanoscale, 2019, 11, 17211–17215 RSC.
- W. Liu, L. Li, J. Jiang, M. Wu and P. Lin, Precis. Clin. Med., 2021, 4, 179–191 CrossRef PubMed.
- Y. Wang, C. Zheng, Y. Wu, B. Zhang, C. Hu, C. Guo, Q. Kong and Y. Wang, Composites, Part B, 2023, 250, 110469 CrossRef CAS.
- M. Ramamoorth and A. Narvekar, J. Clin. Diagn. Res., 2015, 9, Ge01–Ge06 Search PubMed.
- L. Salerno, L. Craxì, E. Amodio and G. Lo Coco, Vaccines, 2021, 9, 927 Search PubMed.
- W. T. Arab, H. H. Susapto, D. Alhattab and C. A. E. Hauser, J. Tissue Eng., 2022, 13, 20417314221111868 CrossRef PubMed.
- W. Li, K. Chu and L. Liu, Langmuir, 2018, 35, 1369–1378 CrossRef PubMed.
- X. Zhang, S. Malhotra, M. Molina and R. Haag, Chem. Soc. Rev., 2015, 44, 1948–1973 RSC.
- M. Longmire, P. L. Choyke and H. Kobayashi, Nanomedicine, 2008, 3(5), 703–717 Search PubMed.
- K. J. McCarthy and D. J. Wassenhove-McCarthy, Microsc. Microanal., 2012, 18, 3–21 CrossRef CAS PubMed.
- L. Xuan, Z. Ju, M. Skonieczna, P. K. Zhou and R. Huang, MedComm, 2023, 4, e327 CrossRef CAS PubMed.
- T. Ojha, V. Pathak, N. Drude, M. Weiler, D. Rommel, S. Rütten, B. Geinitz, M. J. Van Steenbergen, G. Storm and F. Kiessling, Pharmaceutics, 2019, 11, 433 Search PubMed.
- M. S. Gatto and W. Najahi-Missaoui, Int. J. Mol. Sci., 2023, 24, 14041 CrossRef CAS PubMed.
- R. P. B. Ashok, Y. Xiao, K. Lintinen, P. Oinas, M. A. Kostiainen and M. Österberg, Colloids Surf., A, 2020, 587, 124228 CrossRef.
|
This journal is © The Royal Society of Chemistry 2024 |
Click here to see how this site uses Cookies. View our privacy policy here.