DOI:
10.1039/D4BM00357H
(Paper)
Biomater. Sci., 2024,
12, 2705-2716
Macrophage membrane-camouflaged nanoclusters of ultrasmall iron oxide nanoparticles for precision glioma theranostics†
Received
9th March 2024
, Accepted 5th April 2024
First published on 9th April 2024
Abstract
Developing effective nanomedicines to cross the blood–brain barrier (BBB) for efficient glioma theranostics is still considered to be a challenging task. Here, we describe the development of macrophage membrane (MM)-coated nanoclusters (NCs) of ultrasmall iron oxide nanoparticles (USIO NPs) with dual pH- and reactive oxygen species (ROS)-responsivenesses for magnetic resonance (MR) imaging and chemotherapy/chemodynamic therapy (CDT) of orthotopic glioma. Surface citrate-stabilized USIO NPs were solvothermally synthesized, sequentially modified with ethylenediamine and phenylboronic acid, and cross-linked with gossypol to form gossypol-USIO NCs (G-USIO NCs), which were further coated with MMs. The prepared MM-coated G-USIO NCs (G-USIO@MM NCs) with a mean size of 99.9 nm display tumor microenvironment (TME)-responsive gossypol and Fe release to promote intracellular ROS production and glutathione consumption. With the MM-mediated BBB crossing and glioma targeting, the G-USIO@MM NCs can specifically inhibit orthotopic glioma in vivo through the gossypol-mediated chemotherapy and Fe-mediated CDT. Meanwhile, USIO NPs can be dissociated from the NCs under the TME, thus allowing for effective T1-weighted glioma MR imaging. The developed G-USIO@MM NCs with simple components and drug as a crosslinker are promising for glioma theranostics, and may be extended to tackle other cancer types.
Introduction
Glioma, a common type of primary tumor in the brain, originates from the glial cells of the brain.1 Glioma cells primarily infiltrate the brain and spinal cord and generally do not grow outside the central nervous system.2 Glioma can escape from the immune system through a variety of mechanisms, including the secretion of cytokines and the regulation of cell surface molecules. Moreover, the blood–brain barrier (BBB), a physiological barrier with a large number of capillaries,3 is composed of vascular endothelial cells, basement membrane, and astrocytes, which form tight junctions with other surrounding cells,4,5 hence the BBB can strictly control all substances entering or leaving the brain, and limit the treatment efficiency of therapeutic drugs for glioma.
Despite the continuous advancement of nanomedicines,6,7 there are still few accurate early diagnosis techniques for glioma, and magnetic resonance (MR) imaging has often been adopted for glioma diagnosis. The clinically used gadolinium (Gd) chelates are employed to enhance the contrast of MR imaging. However, the Gd-based contrast agents lack specificity, and the leaked free Gd3+ imposes a great burden on the kidneys of the human body,8 often causing systemic fibrosis of the kidneys particularly more common for patients with abnormal kidney function.9–12 Iron (Fe)-based nanomaterials have attracted great attention for MR imaging due to their super paramagnetic properties and excellent biosafety.13 For ultrasmall iron oxide nanoparticles (USIO NPs) with a size less than 5 nm, T1-weighted MR imaging property can be rendered,10 and nanoclusters (NCs) of USIO NPs formed through crosslinkers with different responsivenesses can be endowed with T2–T1 switchable MR imaging property for precision tumor imaging.14–17
Interestingly, Fe, a transition metal, can trigger Fenton reactions with excess hydrogen peroxide in tumor cells to generate hydroxyl radicals (˙OH) for chemodynamic therapy (CDT) of tumors.7,18,19 In addition, Fe-based nanomaterials have also been used as promising drug delivery systems. For instance, the hydrophobic drug dihydroartemisinin (DHA) can be loaded within Fe-based metal–organic-framework for tumor treatment through the responsive release of both DHA and Fe(III) ions.20 In particular, owing to the good biosafety, T1 MR imaging ability, CDT potential, and versatility in chemical modifications, USIO NPs have been regarded as one promising nanoplatform for tumor theranostics.21
For effective cancer theranostics, it is necessary to functionalize the USIO NPs with specific targeting ligands10 or cancer cell membranes (CCMs).15 For example, our group employed a microfluidic method to coat preformed NCs of cystamine-crosslinked USIO NPs with polydopamine that were further loaded with cisplatin and camouflaged with CCMs for theranostics of a murine breast cancer model.22 Emphatically, the nanoplatforms for glioma theranostics have to possess the ability of BBB penetration.23–25 To render nanoplatforms with BBB crossing ability, it is essential to modify their surface with the specific ligands (e.g., dermorphin and transferrin)26,27 or functional groups (e.g., hydroxyl groups).28,29 The surface modification usually takes multiple steps, posing difficulties for further clinical translation practices. Recently, the cell membrane camouflaging has been regarded as a new strategy render nanoplatforms with BBB crossing ability.30 Cell membranes contain containing a large number of specific membrane proteins, such as integrins, peripheral proteins, and lipid-anchored proteins.31 NPs camouflaged with cell membranes can be equipped with the characteristics of endogenous cells to have enhanced immune escape, prolonged blood circulation and homologous targeting.32,33 A previous study reported that the red blood cell membrane coating can effectively extend the blood circulation time of NPs.34 Interestingly, integrin-mediated cell adhesion effect on the surface of MMs allows them to cross the BBB.35–37 Thus, a biomimetic MM-coating strategy can endow nanoplatforms with the ability of BBB penetration. In a recent study, programmed cell death-1-overexpressed MMs were coated onto the surface of poly(lactic-co-glycolic acid) (PLGA)-based nanoplatform incorporated with rapamycin, and the created nanoplatform could penetrate BBB and target glioma for combined chemotherapy and immune checkpoint blockade-mediated immunotherapy.38 However, the potential adverse effects or harm should be considered due to the persistence of PLGA in the cranial cavity.
Here, we aimed to develop a carrier-free MM-coated gossypol-crosslinked USIO nanoclusters (G-USIO@MM NCs) to cross BBB for T1-weighted MR imaging and combinational chemotherapy/CDT of orthotopic glioma (Fig. 1). Firstly, USIO NPs with surface citric acid stabilization were solvothermally synthesized, surface functionalized with ethylenediamine (EDA), grafted with phenylboronic acid (PBA), crosslinked with gossypol to form NCs, and finally coated with the MMs to prepare the G-USIO@MM NCs. The formed G-USIO@MM NCs were exhaustively investigated to reveal their composition, structure, morphology, pH- and reactive oxygen species (ROS)-responsivenesses, ROS generation ability, and r1 relaxometry. We also explored their toxicity to C6 cells (a rat glioma cell line) and bEnd.3 cells (a murine brain microvascular endothelia cell line) in vitro. Lastly, the G-USIO@MM NCs were employed for T1 MR imaging and combined chemotherapy/CDT of an orthotopic glioma in a mouse model in vivo. The main innovation of the developed G-USIO@MM NCs can be illustrated as follows: (1) the G-USIO@MM NCs were designed and synthesized based on a carrier-free strategy using the drug as a crosslinker; (2) ROS-mediated breaking of the phenylborate ester (PBE) bonding enables G-USIO@MM NCs to responsively release gossypol and Fe, achieving combination chemotherapy and CDT; and (3) G-USIO@MM NCs can penetrate the BBB for accurate theranostics of orthotopic glioma.
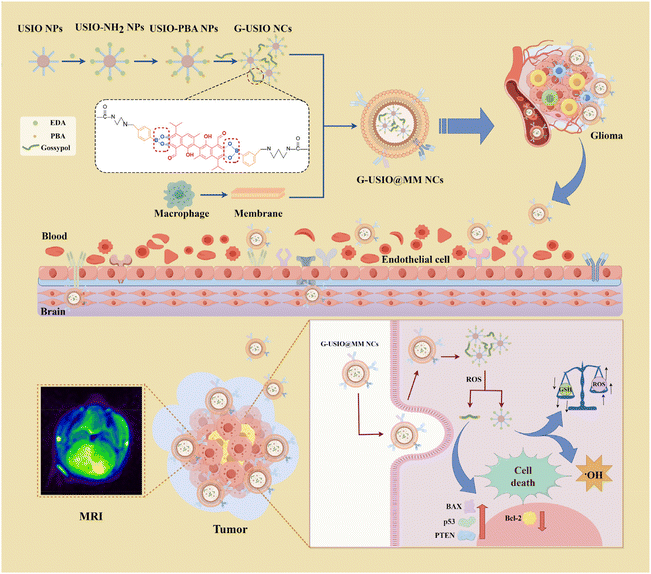 |
| Fig. 1 Preparation of BBB-crossing G-USIO@MM NCs for MR imaging and chemotherapy/CDT of orthotopic glioma. | |
Experimental section
Synthesis and characterization of G-USIO@MM NCs
The citric acid-stabilized USIO NPs were prepared according to our earlier work.39 To obtain the G-USIO NCs, USIO NPs were dispersed in water, activated by 1-(3-dimethylaminopropyl)-3-ethylcarbodiimide hydrochloride and N-hydroxysuccinimide, and reacted with EDA to generate aminated USIO NPs, which were further functionalized with 4-(bromomethyl) PBA. The created USIO-PBA NPs were subsequently crosslinked by gossypol through PBE bonding to get G-USIO NCs. Finally, the MM extracted from RAW264.7 cells were used to coat G-USIO NCs using an Avanti micro-extruder to acquire the G-USIO@MM NCs.
Cell culture and assays
C6 cells and bEnd.3 cells were regularly cultured, passaged and used for in vitro experiments, including cell viability and cellular uptake assays, intracellular depletion of GSH, intracellular ROS generation, intracellular LPO accumulation, cell apoptosis assay, and western blot (WB) assay. In vitro hemolysis assay was used to check the hemocompatibility of the materials.
Animal experiments
All animal experiments were carried out after approval by the Ethical Committee for Experimental Animal Care and Use of Donghua University (approval number: DHUEC-NSFC-2023-02) and also according to the policy of the National Ministry of Health of China. ICR mice (6–8 weeks) were used to establish orthotopic glioma model for in vivo experiments including T1-weighted MR imaging, tumor inhibition assessments, histological examinations, and tissue/organ biodistribution. Blood routine and blood biochemical analysis of healthy mice were additionally used to evaluate the biosafety of the G-USIO@MM NCs.
Results and discussion
Synthesis and characterization of G-USIO@MM NCs
The solvothermally synthesized USIO NPs with surface citric acid residues15 and a mean diameter of 3.0 nm (Fig. S1†) were aminated by EDA and further modified with PBA through a substitution reaction. Subsequently, catechol-containing gossypol was utilized as a crosslinker to form gossypol-loaded USIO NCs (G-USIO NCs) through a PBE bond formation. To achieve the optimal loading of gossypol, the formation of G-USIO NCs was carried out using different molar ratios of PBA for USIO-PBA NPs and gossypol (Table S1†). Under the optimal PBA/gossypol molar ratio at 1
:
5, the G-USIO NCs display ideal encapsulation efficiency (90.5%) and loading content (20.8%) of gossypol.
Then, the G-USIO NCs were coated with MMs to obtain the G-USIO@MM NCs via an Avanti mini extruder. The NCs before and after MM coating were observed by transmission electron microscopy (TEM). As can be seen in Fig. 2A and B, MM-free G-USIO NCs display a random clustered structure, while after MM coating, the G-USIO@MM NCs exhibit a clear spherical shape, indicating the successful MM coating. Furthermore, the presence of MM protein bands of G-USIO@MM NCs assayed through the sodium dodecyl sulfate-polyacrylamide gel electrophoresis (SDS-PAGE) also proved the MM coating on the surface of G-USIO NCs (Fig. S2†). Dynamic light scattering (DLS) data show that the hydrodynamic diameters of USIO NPs, USIO NPs-NH2, USIO NPs-PBA, G-USIO NCs and G-USIO@MM NCs are 32.3 ± 20.0, 105.8 ± 16.1, 154.7 ± 16.3, 195.2 ± 38.4, and 323.3 ± 17.0 nm, respectively (Table S2†). After modified by EDA and PBA, and crosslinked by gossypol, the hydrodynamic diameters of the NPs and NCs increase gradually. Meanwhile, these NPs and NCs show good water dispersibility (Fig. 2C). As shown in the Table S2† and Fig. 2D, the surface potential of citric acid-stabilized USIO NPs is −41.1 ± 1.4 mV, which is owing to the existence of surface citric acid residues. The surface potential of USIO-NH2 NPs increases to −16.4 ± 0.5 mV after amination, which can be ascribed to the consumption of carboxyl groups to form primary amines after EDA modification. Following further PBA modification, the USIO-PBA NPs display a decreased surface potential (−20.3 ± 0.9 mV), which can be attributed to the reduced amount of amine groups and the exposure of the PBA groups on the particle surface. After gossypol crosslinking, the G-USIO NCs show a high zeta potential (−11.0 ± 1.1 mV), possibly attributed to the reduced PBA exposure on the particle surface. The final product of G-USIO@MM NCs shows a further decreased surface potential of −26.4 ± 1.5 mV, validating the successful coating of negatively charged MMs.
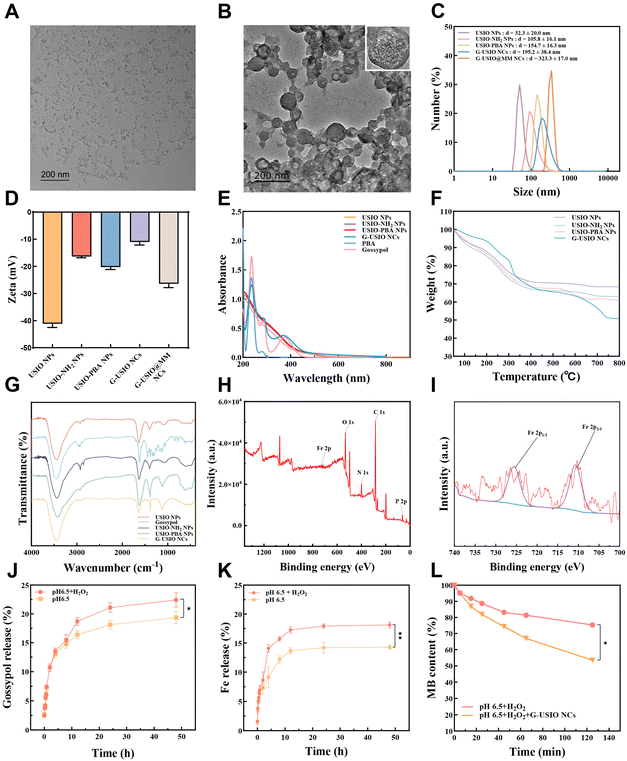 |
| Fig. 2 TEM images of G-USIO NCs (A) and G-USIO@MM NCs (B). Hydrodynamic size (C) and zeta potential (D) of different NPs or NCs. (E) UV-vis spectra of different NPs or NCs, PBA and gossypol. TGA curves (F) and FTIR spectra (G) of different materials. The XPS survey of G-USIO@MM NCs (H) and Fe 2p (I). Release curves of gossypol (J) and Fe (K) from the G-USIO@MM NCs (n = 3). (L) Degradation of MB by the generated ROS under different conditions. In parts J, K, and L, * is for p < 0.05 and ** is for p < 0.01, respectively. | |
Furthermore, the absorption peak at 380 nm can be found in the UV-vis spectrum of G-USIO NCs, confirming the successful gossypol loading (Fig. 2E). Thermal gravimetric analysis (TGA) data (Fig. 2F) show that the weight loss of USIO-NH2 NPs (37.0%) is higher than that of USIO NPs (31.7%) at 800 °C, indicating a surface modification of 5.3% of EDA. Through the weight losses of USIO-PBA NPs (39.1%) and G-USIO NCs (49.3%), one can deduce the PBA modification degree and the gossypol loading content to be 2.1% and 10.2%, respectively. In the Fourier transform infrared (FTIR) spectra (Fig. 2G), the peaks at 581 and 2874 cm−1 can be assigned to Fe–O and methylene of citric acid, respectively, while the gossypol benzene ring features can be seen at 779 and 1458 cm−1. In addition, the comprehensive X-ray photoelectron spectroscopy (XPS) spectrum (Fig. 2H) and the high-resolution XPS spectrum of Fe 2p (Fig. 2I) showcase the presence of C, N, P, O, Fe2+, and Fe3+. Taken together, all the employed characterizations confirmed the formation of G-USIO@MM NCs.
Release kinetics of Gossypol and Fe
The ROS-responsive release of gossypol and Fe(III) ions at pH 6.5 was next studied. Similar to our earlier study,22 the G-USIO@MM NCs can achieve a higher release amount of both gossypol (22.4%) and Fe (18.1%) under pH 6.5 and hydrogen peroxide (H2O2) after 24 h than those of the respective release of gossypol (19.4%) (p < 0.05) and Fe (14.3%) (p < 0.01) under pH 6.5 without H2O2 (Fig. 2J–K). This observation can be attributed to the potential disruption of PBE bonds in the presence of H2O2 under pH 6.5, resulting in faster dissociation of the clusters than under pH 6.5 without H2O2.
MB degradation
Methylene blue (MB) degraded by ˙OH was used to investigate the ˙OH generation of the G-USIO NCs (Fig. 2L and Fig. S3†). The MB degradation trend is very different in the presence and absence of G-USIO NCs. The G-USIO NCs seem to induce more significant degradation of MB than the case without G-USIO NCs (p < 0.05), which should be attributed to the Fenton/Fenton-like reaction between H2O2 and Fe2+/Fe3+ that are formed from the disintegrated G-USIO NCs under an acidic environment containing H2O2. This implies that the incorporated USIO NPs under the tumor microenvironment (TME), namely pH 6.5 and H2O2, enable the significant production of ˙OH, thus affording effective CDT of tumors.
Cytotoxicity and cellular uptake assays in vitro
The cytotoxicity of G-USIO@MM NCs was examined using C6 cells in order to prove their anticancer efficacy (Fig. S4†). For comparison, USIO NPs, G-USIO NCs, and free gossypol were also tested under the same conditions. Clearly, USIO NPs do not display apparent cytotoxicity. In contrast, both G-USIO and G-USIO@MM NCs incorporated with gossypol render the cells with decreased viability with the increase of Fe concentration (Fig. S4A†), similar to the case of free gossypol (Fig. S4B†). The gossypol half maximal inhibitory concentrations (IC50) for the G-USIO NCs, G-USIO@MM NCs, and free gossypol were measured to be 46.7, 32.4, and 1.0 μg mL−1, respectively. As can be seen in Fig. 3A, the USIO NPs only display slight toxicity to C6 cells, likely attributed to the single CDT effect. The G-USIO NCs and G-USIO@MM NCs with the combination chemotherapy (gossypol) and CDT (USIO NPs) exhibit a better anticancer activity than USIO NPs (30, 40, and 50 μg mL−1, p < 0.001) (Fig. 3A and Fig. S5†). In the G-USIO@MM NCs group, the cell viability decreases more significantly than that in the G-USIO NCs group (p < 0.001), which could be ascribed to the MM-mediated targeting effect on C6 cells since MM have overexpressed integrin α4 and β1, thus increasing the accumulation of the G-USIO@MM NCs in C6 cells. Moreover, in comparison to free gossypol, the G-USIO NCs display similar dose-dependent cytotoxicity, while free gossypol shows a better cytotoxicity than G-USIO NCs at each studied concentration except 10.4 μg mL−1 (Fig. S6†, p < 0.05). This means that the G-USIO NCs need a certain time period to release gossypol to exert the dominant chemotherapy effect, and this in vitro therapeutic effect of the NCs cannot be comparable to the in vivo data.
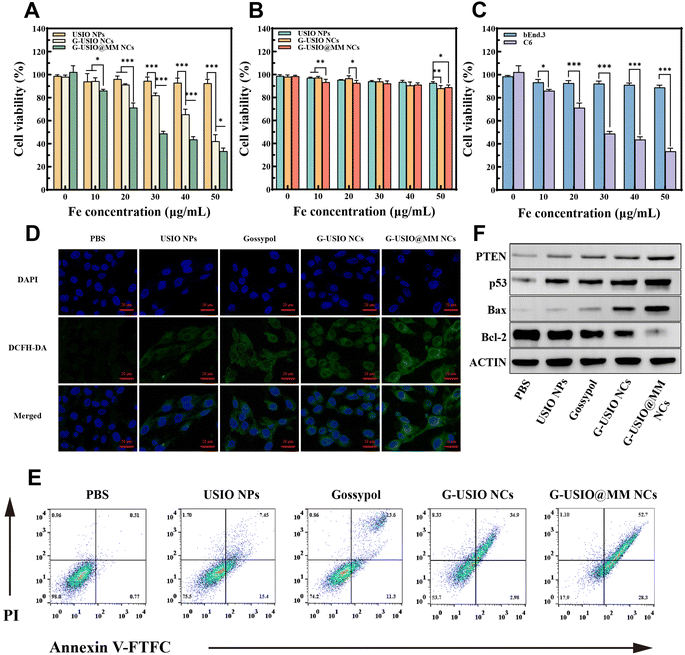 |
| Fig. 3
In vitro CCK-8 viability assay of C6 cells (A) and bEnd.3 cells (B) after treatment with different NPs or NCs at varying Fe concentrations for 24 h (n = 6). (C) Cell viability assay of bEnd.3 and C6 cells treated with G-USIO@MM NCs for 24 h (n = 6). (D) CLSM images of intracellular ROS (green) generation in C6 cells after 24 h treatment in different groups (scale bar = 20 μm). (E) Apoptosis of C6 cells treated with different materials for 24 h. (F) Expression levels of apoptosis-associated protein after treated for 24 h using ACTIN as a reference. In parts A, B and C, * is for p < 0.05, ** is for p < 0.01 and *** is for p < 0.001, respectively (n = 3). | |
Additionally, the cell viability of bEnd.3 cells (the main component of BBB) after different treatments was assessed to investigate the cytocompatibility and targeting effect of the G-USIO@MM NCs (Fig. 3B). All NPs and NCs display little toxicity to bEnd.3 cells even some significant difference can be found between groups, implying the great selectivity of the designed nanomedicine that is responsive to TME. The selectivity of the G-USIO@MM NCs was further compared to cancer cells (Fig. 3C). The viability of bEnd.3 cells is significantly higher than that of C6 cells at the same Fe concentrations (20, 30, 40, and 50 μg mL−1, p < 0.001), which should be due to the much lower amount of Fe and free gossypol released under the physiological condition for bEnd.3 cells, which also cannot be targeted by MMs coated as well.
To confirm the MM coating-rendered enhanced cellular uptake of NCs in vitro, the cellular uptake of G-USIO@MM NCs by C6 cells was quantified by ICP-OES analysis of Fe content in cells (Fig. S7†). Cells treated with all materials except phosphate buffered saline (PBS) exhibit a concentration-dependent cellular Fe uptake. Among all groups, the group of G-USIO@MM NCs displays the highest cellular Fe uptake especially at high Fe concentrations (p < 0.001 for [Fe] = 30 μg mL−1 or above), which is likely due to the targeting effect by integrin α4 and β1 on the MMs towards C6 cells.40 Notably, the NCs display a better cellular Fe uptake than single USIO NPs, in good agreement with the literature.15
ROS generation in vitro
The intracellular ROS production capacity after C6 cells were treated with different materials was detected by confocal laser scanning microscopy (CLSM) using 2,7-dichlorodihydrofluorescein diacetate (DCFH-DA) as a probe in vitro (Fig. 3D and Fig. S8†). The strongest green fluorescence intensity can be observed in C6 cells treated with the G-USIO@MM NCs among all groups. This indicates that the G-USIO@MM NCs enable the production of the highest ROS level among all groups at the same Fe concentration (30 μg mL−1, and the corresponding free gossypol concentration was 6.2 μg mL−1), likely attributed to the combination of Fe-catalyzed Fenton/Fenton-like reactions and gossypol-mediated ROS generation, and the MM-mediated enhanced cellular uptake of the NCs as well.
GSH depletion in vitro
The intracellular GSH level was further detected using a GSH/GSSG Kit after C6 cells were differently treated. As displayed in Fig. S9†, the content of intracellular GSH decreases significantly, indicating that GSH was oxidized by the generated ROS. Compared with the PBS group, the GSH content in the USIO NPs group significantly reduces (p < 0.001), which can be attributed to the released Fe3+/Fe2+-mediated consumption of GSH. Compared with the single treatment (gossypol or USIO NPs), the increased GSH consumption after treatment with G-USIO and G-USIO@MM NCs might be ascribed to the combination effects of both gossypol and USIO NPs to lead to enhanced ROS production. The GSH consumption in the group of G-USIO@MM NCs (54.9%) is higher than in the group of G-USIO NCs (52%), which should be due to the MM-mediated cancer cell targeting effect.
Intracellular LPO accumulation and cell apoptosis
It has been reported that the depletion of GSH results in lipid peroxide (LPO) accumulation that can induce cell death.41 The LPO accumulation and apoptosis of C6 cells were next explored after different treatments. The shift of red to green fluorescence indicates the LPO accumulation in cells. In comparison with the PBS group, the green fluorescence intensity (LPO) increases in all other experimental groups (p < 0.001), and the corresponding red fluorescence intensity (unoxidized lipids) decreases (Fig. S10†). The G-USIO@MM NCs group shows the highest green fluorescence intensity (p < 0.001) and the lowest red fluorescence intensity among all groups. This should be attributed to the targeted uptake of G-USIO@MM NCs by C6 cells, resulting in the highest ROS production (Fig. S8†) and subsequent the greatest LPO accumulation.
Cell apoptosis assays were conducted to investigate the anticancer effect of NCs (Fig. 3E and Fig. S11†). C6 cells treated with USIO NPs show a total apoptosis and necrosis rate of 24.5%, validating the CDT performance of USIO NPs.39,42,43 In addition, the total apoptosis and necrosis rate of C6 cells treated with free gossypol is 25.8%, showing the tendency of gossypol to induce cancer cell apoptosis, in agreement with the literature.44 The sum of apoptotic and necrosis rate of G-USIO@MM NCs group is greater than that of G-USIO NCs group (p < 0.001), which should be ascribed to the MM-assisted specific targeting of NCs to C6 cells.
Furthermore, WB assay was used to examine the expression levels of apoptosis-associated proteins (PTEN, p53, BAX, and Bcl-2) in C6 cells after different treatments to identify the underlying therapeutic mechanism (Fig. 3F and Fig. S12†). Cells in the G-USIO@MM NCs group show the most significant upregulation of tumor suppressor protein p53/PTEN and proapoptotic protein Bax and downregulation of anti-apoptotic protein Bcl-2 among all groups (p < 0.001), indicating the most significant activation of the proapoptotic pathwa.45 This again can be attributed to the MM coating-facilitated specific uptake of NCs by C6 cells that can induce the combination chemotherapy and CDT.
T
1-Weighted MR imaging
Subsequently, T1 MR relaxometry was carried out to validate the potential of the G-USIO@MM NCs for MR imaging at pH 6.5 with or without H2O2 (Fig. 4A). The MR signal intensity of the G-USIO@MM NCs increases proportionally with the increase of Fe concentration at pH 6.5 in the presence and absence of H2O2. Furthermore, the r1 relaxivity of G-USIO@MM NCs at pH 6.5 with H2O2 was estimated to be 0.9 mM−1 s−1, much larger than that at pH 6.5 without H2O2 (0.5 mM−1 s−1). This is likely due to the fast disintegration of the NCs and the release of Fe from the G-USIO@MM NCs to form single USIO NPs and Fe(II)/Fe(III) ions. The above results reveal the great potential of the G-USIO@MM NCs for T1-weighted MR imaging applications.
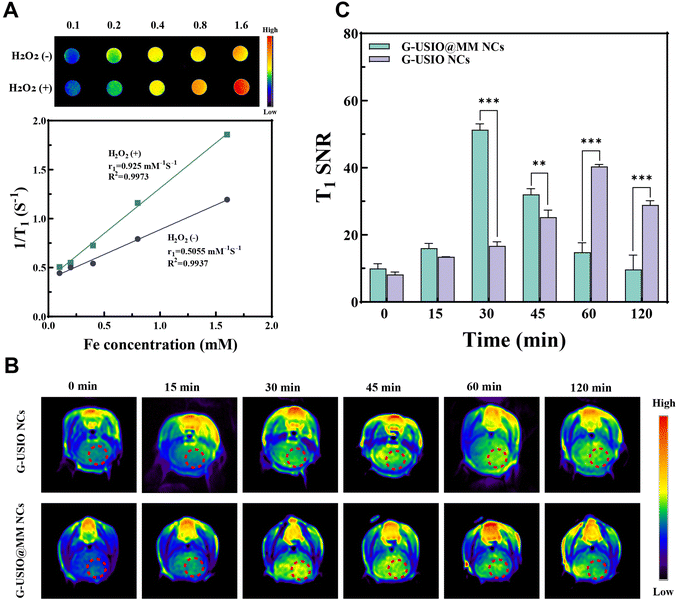 |
| Fig. 4 (A) T1-Weighted MR images and the corresponding plots of 1/T1 of G-USIO@MM NCs versus Fe concentration at pH 6.5 with and without H2O2. T1-Weighted MR images (B) and corresponding signal-to-noise ratio (SNR) of the orthotopic glioma (C) before and at different time points postinjection of G-USIO NCs or G-USIO@MM NCs. In part C, ** is for p < 0.01 and *** is for p < 0.001, respectively (n = 3). | |
Next, we examined the ability of G-USIO@MM NCs to cross BBB for T1-weighted MR imaging of orthotopic glioma in a mouse model in vivo. The G-USIO NCs or G-USIO@MM NCs were injected through the tail vein, then the coronal MR images (Fig. 4B) and SNR (Fig. 4C) of the mouse brain at different time points were obtained and calculated. The MR signal intensity of glioma increases over time after injection of G-USIO@MM NCs and reaches the peak value at 30 min postinjection, and then decreases at later time points. The glioma MR signal intensity in the G-USIO@MM NCs group is remarkably higher than that in the G-USIO NCs group at the same time points (30 min and later time points, p < 0.01). These data demonstrate that the G-USIO@MM NCs can cross the BBB for enhanced MR imaging of orthotopic glioma due to the MM coating.
Combination therapy of orthotopic glioma
We next evaluated the combined therapeutic efficacy of G-USIO@MM NCs in an orthotopic glioma model (Fig. 5A). The therapeutic effects were assessed through real-time monitoring of the tumor volume change via T1-weighted MR imaging (Fig. 5B). The quantitative data of relative tumor volume (V/V0) show that the glioma growth is inhibited to different degrees after different treatments (Fig. 5C). Notably, the G-USIO@MM NCs group shows a more significant inhibitory effect on tumor growth than the other groups (p < 0.01), which should be attributed to the combination of USIO NPs-mediate CDT and gossypol-mediated chemotherapy and the BBB crossing and glioma targeting ability endowed with the MMs, in agreement with the literature.1 The mouse body weight change (Fig. 5D) shows that the tumor-bearing mice lose weight over time in all groups due to the progression of glioma. Apparently, the weight losses of tumor-bearing mice in the G-USIO@MM NCs and G-USIO NCs groups are significantly lower than those in the other groups (p < 0.001), which could be owing to the good biosafety and tumor treatment effect of both materials. Moreover, the significant body weight loss in the free gossypol group indicates its potential toxic side effects in vivo.
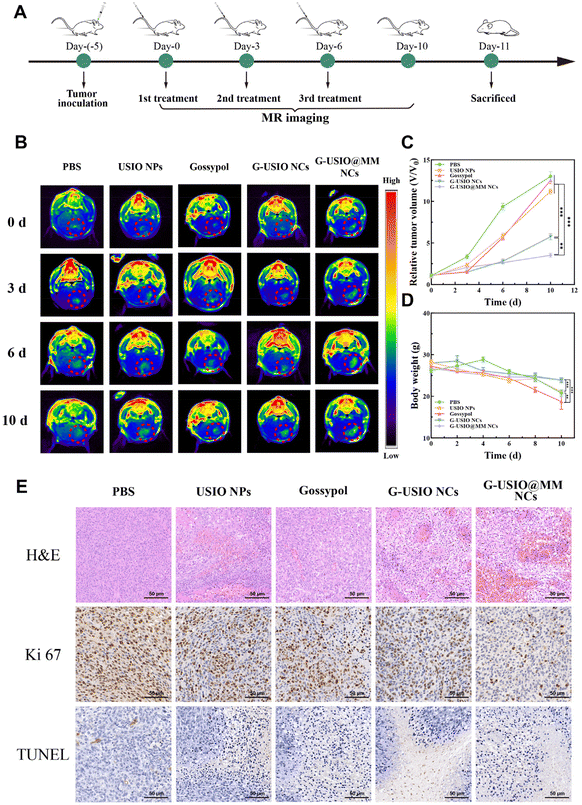 |
| Fig. 5 (A) Schematic illustration of the in vivo treatment process. (B) Representative MR images of brain glioma in different treatment groups after intravenous injection of Magnevist®. The relative tumor volume (C) and body weight (D) of glioma-bearing mice after different treatments. (E) The H&E, Ki 67, and TUNEL staining images of tumor tissues (scale bar = 50 μm). In parts C and D, ** is for p < 0.01 and *** is for p < 0.001, respectively (n = 3). | |
To further evaluate the therapeutic effects, the tumor-bearing mice were sacrificed to obtain the glioma tissue for hematoxylin and eosin (H&E), Ki67, and TdT-mediated dUTP nick-end labeling (TUNEL) staining assays, respectively (Fig. 5E). Necrosis, apoptosis and proliferation inhibition of tumor cells can be seen in the glioma slices for all groups except the PBS group. Among all groups, the treatment with the G-USIO@MM NCs leads to distinct inhibition of tumor cell proliferation and significant tumor cell necrosis/apoptosis in vivo, due to their best efficacy in BBB penetration and combination therapy, in consistence with the tumor treatment data (Fig. 5B and C).
Biosafety evaluation in vivo
To evaluate the biosafety of G-USIO@MM NCs, we collected the major organs of tumor-bearing mice at 10 days, and performed H&E staining (Fig. S13†). No obvious pathologic abnormality and toxic side effects can be found in all the main organs such as heart, liver, spleen, lung, and kidney in different groups including the group of G-USIO@MM NCs, indicating that all materials studied are quite safe. Furthermore, we studied the pathological condition of brain in healthy mice after intravenous injection of the G-USIO@MM NCs via H&E staining (Fig. 6A). No significant histological damage can be observed, which is the same as the PBS group. Overall, the prepared G-USIO@MM NCs have negligible systemic toxicity in vivo.
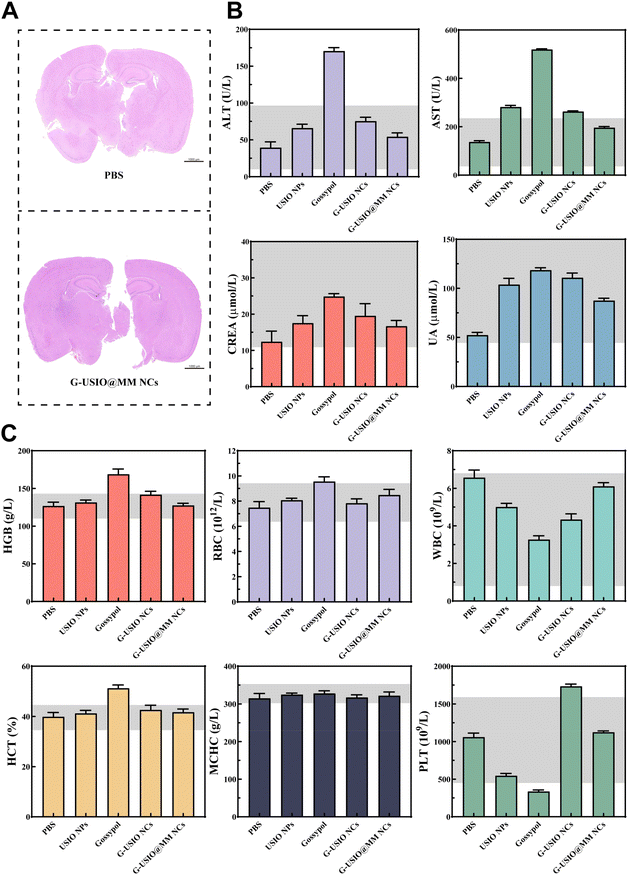 |
| Fig. 6 (A) H&E staining images of the brain tissues obtained from the healthy mice treated with PBS and G-USIO@MM NCs on the 11th day postinjection. The blood biochemical (B) and blood routine (C) indexes after healthy mice were injected with different materials and sacrificed at 14 days postinjection. The blood biochemical data include liver function indices (alanine aminotransferase (ALT), aspartate aminotransferase (AST)) and kidney function indices (creatinine (CREA) and uric acid (UA)). The blood routines involve blood levels of hemoglobin (HGB), red blood cells (RBCs), white blood cells (WBCs), hematocrit (HCT), mean corpuscular hemoglobin concentration (MCHC) and platelet (PLT). Light grey areas represent the normal ranges of different biosafety indicators. Data are shown as mean ± SD (n = 3). | |
The biodistribution behavior of G-USIO@MM NCs in major organs was investigated through ICP-OES analysis of Fe contents at different time points postinjection. As shown in Fig. S14†, the Fe contents in the brain of G-USIO@MM NCs group were significantly higher than that of G-USIO NCs group due to the BBB crossing and targeting specificity rendered by the MM coating, and the highest brain Fe level in the G-USIO@MM NCs group can be found at 1 h postinjection. Moreover, a large amount of the Fe was taken up by the spleen and peaked at 12 h postinjection for both groups of G-USIO and G-USIO@MM NCs. With the extension of time postinjection, the Fe contents decrease gradually in all the vital organs, implying that the developed G-USIO@MM NCs can be gradually metabolized and excreted from the body, further indicating the good biosafety profile of the G-USIO@MM NCs in vivo.
Finally, the biocompatibility of G-USIO@MM NCs was explored through hemolysis, blood routine, and blood biochemistry tests. The blood of the healthy mice was extracted to isolate red blood cells (RBCs), and the hemocompatibility of G-USIO@MM NCs was evaluated through hemolysis assay in vitro (Fig. S15†). Clearly, the hemolysis rates of red blood cells (RBCs) treated with the G-USIO@MM NCs at different concentrations are all far below the threshold value of 5.0%, indicating their favorable hemocompatibility.
The blood of the healthy mice after different treatments for 14 days was collected for biochemistry (Fig. 6B) and blood routine (Fig. 6C) analyses. The indexes of blood routine and blood biochemistry are all in the normal ranges, suggesting that the G-USIO@MM NCs do not cause inflammation, and liver/kidney injury at 14 days postinjection. However, free gossypol seems to cause the parameters of blood routine (HGB and HCT) and blood biochemistry (ALT and AST) anomalies, suggesting that free gossypol induces liver damage. Overall, our data suggest that the designed G-USIO@MM NCs possess a good biosafety profile in different aspects, thus having a great translation potential.
Conclusions
We developed MM-camouflaged gossypol-crosslinked USIO NCs (G-USIO@MM NCs) for BBB crossing and targeted T1 MR imaging and combined orthotopic glioma chemotherapy/CDT. Single USIO NPs surface modified with PBA can be crosslinked by an anticancer drug gossypol to form the G-USIO NCs through PBE bonding with dual pH- and ROS-responsivenesses, which can be further coated with MM. The developed G-USIO@MM NCs show excellent colloidal stability under physiological conditions, dual pH- and ROS-responsivenesses, BBB crossing ability, and glioma targeting specificity, and can simultaneously release gossypol for chemotherapy and USIO NPs or Fe(II)/Fe(III) for both T1-weighted MR imaging and CDT under the TME condition. The collaborative therapy enables the generation of intracellular ROS and the consumption of GSH to effectively promote tumor cell apoptosis and necrosis. The developed G-USIO@MM NCs may be considered as a promising theranostic nanoplatform for BBB crossing and glioma theranostics.
Author contributions
Bin Zhang: methodology, investigation, data curation, validation, formal analysis, writing – original draft. Rui Yang: methodology, investigation, data curation, formal analysis, writing – review & editing. Hongwei Yu: methodology, investigation, data curation. Yamin Peng: methodology, investigation. Haoyu Huang: methodology, investigation. Meera Moydeen Abdul Hameed: validation, formal analysis. Han Wang: supervision, resources. Guixiang Zhang: supervision, resources. Mohamed EL-Newehy: validation, formal analysis. Mingwu Shen: supervision, resources, funding acquisition, project administration. Xiangyang Shi: conceptualization, supervision, resources, funding acquisition, project administration, writing – review & editing. Shaojun Peng: supervision, resources.
Conflicts of interest
The authors declare no competing financial interest.
Acknowledgements
This research has been financially supported by the National Natural Science Foundation of China (U23A2096, 52350710203 and 82172073), the Science and Technology Commission of Shanghai Municipality (23520712500, 21490711500, and 20DZ2254900), the National Key R&D Program (2022YFE0196900), and the Shanghai Education Commission through the leading talent program. This project was also supported by Researchers Supporting Project Number (RSPD2024R769), King Saud University, Riyadh, Saudi Arabia. X. S. greatly acknowledges the support by the Fundação para a Ciência e a Tecnologia (FCT) with Portuguese Government funds through the CQM Base Fund - UIDB/00674/2020 (DOI: 10.54499/UIDB/00674/2020) and Programmatic Fund - UIDP/00674/2020 (DOI: 10.54499/UIDP/00674/2020).
References
- T. Xiao, M. He, F. Xu, Y. Fan, B. Jia, M. Shen, H. Wang and X. Shi, ACS Nano, 2021, 15, 20377–20390 CrossRef CAS PubMed.
- H. S. Venkatesh, W. Morishita, A. C. Geraghty, D. Silverbush, S. M. Gillespie, M. Arzt, L. T. Tam, C. Espenel, A. Ponnuswami, L. Ni, P. J. Woo, K. R. Taylor, A. Agarwal, A. Regev, D. Brang, H. Vogel, S. Hervey-Jumper, D. E. Bergles, M. L. Suvà, R. C. Malenka and M. Monje, Nature, 2019, 573, 539–545 CrossRef CAS PubMed.
- Y. Ma, J. Zhang, Y. Rui, J. Rolle, T. Xu, Z. Qian, Y. Gu and S. Li, Biomaterials, 2021, 268, 120564 CrossRef CAS PubMed.
- C. Wang, B. Wu, Y. Wu, X. Song, S. Zhang and Z. Liu, Adv. Funct. Mater., 2020, 30, 1909369 CrossRef CAS.
- J. Lai, G. Deng, Z. Sun, X. Peng, J. Li, P. Gong, P. Zhang and L. Cai, Biomaterials, 2019, 211, 48–56 CrossRef CAS PubMed.
- L. L. Zheng, B. Hu, D. Y. Zhao, W. J. Liu, Q. Liu, Y. Y. Huang and S. B. Ruan, Chin. Chem. Lett., 2024, 35, 108647 CrossRef CAS.
- Z. Sun and Y. Hou, BMEMat, 2023, 1, e12012 CrossRef.
- T. Grobner, Nephrol., Dial., Transplant., 2006, 21, 1745–1745 CrossRef CAS.
- A. Oussalah, V. Laurent, O. Bruot, A. Bressenot, M. A. Bigard, D. Régent and L. Peyrin-Biroulet, Gut, 2010, 59, 1056–1065 CrossRef PubMed.
- Y. Luo, J. Yang, Y. Yan, J. Li, M. Shen, G. Zhang, S. Mignani and X. Shi, Nanoscale, 2015, 7, 14538–14546 RSC.
- W. A. High, R. A. Ayers and S. E. Cowper, J. Am. Acad. Dermatol., 2007, 56, 710–712 CrossRef PubMed.
- J. L. Abraham and C. Thakral, Eur. J. Radiol., 2008, 66, 200–207 CrossRef PubMed.
- H. Gu, S. W. Lee, J. Carnicelli, T. Zhang and D. Ren, Nat. Commun., 2020, 11, 2211 CrossRef CAS PubMed.
- D. Ma, M. Shi, X. Li, J. Zhang, Y. Fan, K. Sun, T. Jiang, C. Peng and X. Shi, Bioconjugate Chem., 2020, 31, 352–359 CrossRef CAS PubMed.
- L. Jia, X. Li, H. Liu, J. Xia, X. Shi and M. Shen, Nano Today, 2021, 36, 101022 CrossRef CAS.
- G. Yang, Y. Liu, J. Chen, J. Ding and X. Chen, Acc. Mater. Res., 2022, 3, 1232–1247 CrossRef CAS.
- J. Chen, Z. Jiang, Y. S. Zhang, J. Ding and X. Chen, Appl. Phys. Rev., 2021, 8, 041321 CAS.
- C. Zhang, W. Bu, D. Ni, S. Zhang, Q. Li, Z. Yao, J. Zhang, H. Yao, Z. Wang and J. Shi, Angew. Chem., Int. Ed., 2016, 55, 2101–2106 CrossRef CAS PubMed.
- X. Ma, Y. Wang, X.-L. Liu, H. Ma, G. Li, Y. Li, F. Gao, M. Peng, H. M. Fan and X.-J. Liang, Nanoscale Horiz., 2019, 4, 1450–1459 RSC.
- D. Wang, J. Zhou, R. Chen, R. Shi, G. Xia, S. Zhou, Z. Liu, N. Zhang, H. Wang, Z. Guo and Q. Chen, Biomaterials, 2016, 107, 88–101 CrossRef CAS PubMed.
- Y. Gao, X. Shi and M. Shen, ACS Appl. Mater. Interfaces, 2021, 13, 45119–45129 CrossRef CAS PubMed.
- R. Yang, Z. Ouyang, H. Guo, J. Qu, J. Xia, M. Shen and X. Shi, Nano Today, 2022, 46, 101615 CrossRef CAS.
- M. Wu, W. Chen, Y. Chen, H. Zhang, C. Liu, Z. Deng, Z. Sheng, J. Chen, X. Liu, F. Yan and H. Zheng, Adv. Sci., 2018, 5, 1700474 CrossRef PubMed.
- P. Hu, H. Mo, S. Song, J. Wu, J. Li and J. Shen, J. Mater. Chem. B, 2023, 11, 4799–4807 RSC.
- L. D. Carlos, Light: Sci. Appl., 2022, 11, 149 CrossRef CAS PubMed.
- C. Song, Z. Ouyang, Y. Gao, H. Guo, S. Wang, D. Wang, J. Xia, M. Shen and X. Shi, Nano Today, 2021, 41, 101325 CrossRef CAS.
- H. He, Y. Li, X.-R. Jia, J. Du, X. Ying, W.-L. Lu, J.-N. Lou and Y. Wei, Biomaterials, 2011, 32, 478–487 CrossRef CAS PubMed.
- F. Zhang, P. Mastorakos, M. K. Mishra, A. Mangraviti, L. Hwang, J. Zhou, J. Hanes, H. Brem, A. Olivi, B. Tyler and R. M. Kannan, Biomaterials, 2015, 52, 507–516 CrossRef CAS PubMed.
- J. Wang, Z. Wang, G. Zhang, J. Rodrigues, H. Tomas, X. Shi and M. Shen, Biomater. Sci., 2024, 12, 1346–1356 RSC.
- H. Liu, Y. Han, T. Wang, H. Zhang, Q. Xu, J. Yuan and Z. Li, J. Am. Chem. Soc., 2020, 142, 21730–21742 CrossRef CAS PubMed.
- L. Chen, W. Hong, W. Ren, T. Xu, Z. Qian and Z. He, Signal Transduction Targeted Ther., 2021, 6, 225 CrossRef PubMed.
- J. Li, X. Zhen, Y. Lyu, Y. Jiang, J. Huang and K. Pu, ACS Nano, 2018, 12, 8520–8530 CrossRef CAS PubMed.
- Y. Guo, Z. Wang, X. Shi and M. Shen, Exploration, 2022, 2, 20210171 CrossRef PubMed.
- J. Su, H. Sun, Q. Meng, Q. Yin, S. Tang, P. Zhang, Y. Chen, Z. Zhang, H. Yu and Y. Li, Adv. Funct. Mater., 2016, 26, 1243–1252 CrossRef CAS.
- J. Si, S. Shao, Y. Shen and K. Wang, Small, 2016, 12, 5108–5119 CrossRef CAS PubMed.
- K. Ley, C. Laudanna, M. I. Cybulsky and S. Nourshargh, Nat. Rev. Immunol., 2007, 7, 678–689 CrossRef CAS PubMed.
- H. Cao, Z. Dan, X. He, Z. Zhang, H. Yu, Q. Yin and Y. Li, ACS Nano, 2016, 10, 7738–7748 CrossRef CAS PubMed.
- T. Yin, Q. Fan, F. Hu, X. Ma, Y. Yin, B. Wang, L. Kuang, X. Hu, B. Xu and Y. Wang, Nano Lett., 2022, 22, 6606–6614 CrossRef CAS PubMed.
- R. Yang, R. Wu, B. Zhang, H. Guo, J. Qu, J. Xia, G. Zhang, M. Shen and X. Shi, Adv. Sens. Res., 2023, 2, 2200089 CrossRef CAS.
- S. Quader, X. Liu, Y. Chen, P. Mi, T. Chida, T. Ishii, Y. Miura, N. Nishiyama, H. Cabral and K. Kataoka, J. Controlled Release, 2017, 258, 56–66 CrossRef CAS PubMed.
- X.-X. Yang, X. Xu, M.-F. Wang, H.-Z. Xu, X.-C. Peng, N. Han, T.-T. Yu, L.-G. Li, Q.-R. Li, X. Chen, Y. Wen and T.-F. Li, J. Nanobiotechnol., 2022, 20, 230 CrossRef CAS PubMed.
- Z. Shen, T. Liu, Y. Li, J. Lau, Z. Yang, W. Fan, Z. Zhou, C. Shi, C. Ke, V. I. Bregadze, S. K. Mandal, Y. Liu, Z. Li, T. Xue, G. Zhu, J. Munasinghe, G. Niu, A. Wu and X. Chen, ACS Nano, 2018, 12, 11355–11365 CrossRef CAS PubMed.
- K. Luo, J. Zhao, C. Jia, Y. Chen, Z. Zhang, J. Zhang, M. Huang and S. Wang, ACS Appl. Mater. Interfaces, 2020, 12, 22650–22660 CrossRef CAS PubMed.
- H. Zhu, C. Huang, J. Di, Z. Chang, K. Li, S. Zhang, X. Li and D. Wu, ACS Nano, 2023, 17, 12544–12562 CrossRef CAS PubMed.
- Y. Zeng, H. Liu, J. Ma, K. Li, P. Chang, C. Wang, L. Li, D. Chen, C. Liu, N. Li, W. Zhan and Y. Zhan, Small, 2023, 19, 2300104 CrossRef CAS PubMed.
|
This journal is © The Royal Society of Chemistry 2024 |
Click here to see how this site uses Cookies. View our privacy policy here.