DOI:
10.1039/D4BM00848K
(Review Article)
Biomater. Sci., 2024,
12, 4875-4902
Advances in implants and bone graft types for lumbar spinal fusion surgery†
Received
25th June 2024
, Accepted 14th August 2024
First published on 20th August 2024
Abstract
The increasing prevalence of spinal disorders worldwide necessitates advanced treatments, particularly interbody fusion for severe cases that are unresponsive to non-surgical interventions. This procedure, especially 360° lumbar interbody fusion, employs an interbody cage, pedicle screw-and-rod instrumentation, and autologous bone graft (ABG) to enhance spinal stability and promote fusion. Despite significant advancements, a persistent 10% incidence of non-union continues to result in compromised patient outcomes and escalated healthcare costs. Innovations in lumbar stabilisation seek to mimic the properties of natural bone, with evolving implant materials like titanium (Ti) and polyetheretherketone (PEEK) and their composites offering new prospects. Additionally, biomimetic cages featuring precisely engineered porosities and interconnectivity have gained traction, as they enhance osteogenic differentiation, support osteogenesis, and alleviate stress-shielding. However, the limitations of ABG, such as harvesting morbidities and limited fusion capacity, have spurred the exploration of sophisticated solutions involving advanced bone graft substitutes. Currently, demineralised bone matrix and ceramics are in clinical use, forming the basis for future investigations into novel bone graft substitutes. Bioglass, a promising newcomer, is under investigation despite its observed rapid absorption and the potential for foreign body reactions in preclinical studies. Its clinical applicability remains under scrutiny, with ongoing research addressing challenges related to burst release and appropriate dosing. Conversely, the well-documented favourable osteogenic potential of growth factors remains encouraging, with current efforts focused on modulating their release dynamics to minimise complications. In this evidence-based narrative review, we provide a comprehensive overview of the evolving landscape of non-degradable spinal implants and bone graft substitutes, emphasising their applications in lumbar spinal fusion surgery. We highlight the necessity for continued research to improve clinical outcomes and enhance patient well-being.
1. Introduction
Spinal fusion, a surgical procedure designed to stabilise the spine and alleviate nerve compression by fusing two or more vertebrae, is essential for managing a spectrum of degenerative spinal conditions. This intervention becomes necessary when non-surgical treatments for spinal pathologies, encompassing degenerative disc disease, spondylolisthesis, spinal fractures, spinal stenosis, and spondylolysis, prove inadequate. Notably, these spinal disease entities contribute to more than half of the musculoskeletal diseases in the United States (US) (51.7% or 15.4 million incidents),1 increasing by more than 60% in the last two decades.2 The most significant growth rate is observed in the demographic aged 65 and over, which is attributed to factors including an ageing population, changing lifestyles – amongst others marked by a higher incidence of obesity – and improved patient awareness of fusion procedures.2,3 However, spine surgery revision rates of 10% to 20% are a significant challenge for patients, surgeons, and the healthcare system in general.4–7
The challenges of lumbar spinal fusion surgery are rooted in the anatomical exposure of adjacent, highly vulnerable structures, and the difficulty of translating recent advancements in its essential components from research into clinical practice. These components include spinal instrumentation for posterior lumbar stabilisation, spinal cages for lumbar interbody fusion, and bone grafts aimed at facilitating spinal fusion (Fig. 1). Thus, to have a thorough understanding of these inherent challenges necessitates a comprehensive exploration into the historical developments of lumbar spinal fusion,8 while also concentrating on its primary components: spinal instrumentation, intervertebral body fusion devices and bone grafts. Drawing on both historical and contemporary evidence, this narrative review seeks to provide a comprehensive overview and critical analysis of the most significant developments, which are crucial for guiding future research and enhancing the clinical success of lumbar spinal fusion.
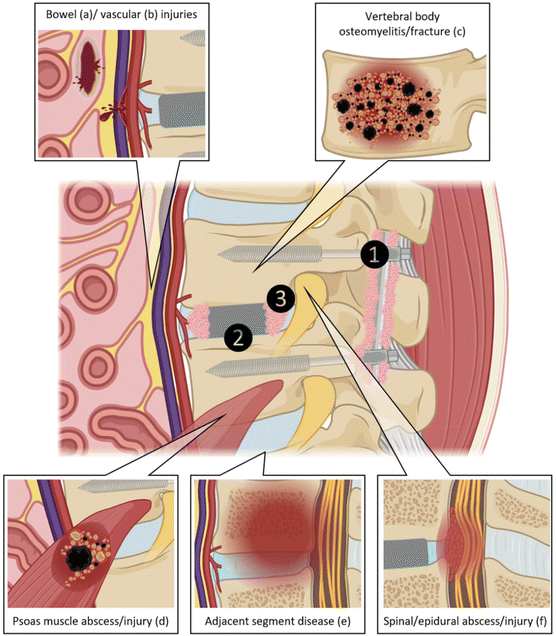 |
| Fig. 1 Anatomical exposure of adjacent, highly vulnerable structures in lumbar spinal fusion surgery and its main components of posterior stabilization (1), interbody cage (2) and bone graft (3). Particularly during anterior approaches to the lumbar spine, the bowel (a) and blood vessels (b) can be damaged. Potential complications during lumbar spinal fusion surgeries or during the post-operative follow-up include vertebral body osteomyelitis/fracture (c), psoas muscle abscess/injury (d), adjacent segment disease (e) and spinal/epidural abscess/injury (f). Adapted from supplement of ref. 8 with permission from Elsevier, copyright (2022) and partially created with BioRender.com. | |
2. Key historical events
The earliest descriptions of treatments for spinal deformity trace back to ancient Indian religious literature, specifically the Srimad Bhagwat Mahapuranam. This text recounts a Hindu mythological epic in which Lord Krishna corrects a “hunchback” by anchoring the patient's foot with his own and applying axial traction by pulling the patient's chin with two fingers.9 The principle of axial traction for spinal deformity correction endured for millennia. Advancements of several existing corrective procedures are further rooted in early spinal treatment descriptions applied by Hippocrates (460 BC to 377 BC), Galen (131 AD to 201 AD) and Ibn Sena (980 AD to 1037 AD).9–11 Nonetheless, the breadth of spinal surgical treatment options witnessed significant evolution only following the profound discoveries of general anaesthesia (1846), antisepsis (1867) and the advent of X-ray (1895).12–14 The rise of (spinal) tuberculosis, including Pott's disease in the 18th and 19th centuries,15,16 resulted in the need to develop alternative stabilising methods due to frequently observed severe destruction of vertebral bodies and intervertebral discs.17 The goal of instrumented spine surgery has thus notably shifted in the last century from a method of correcting deformities, to one of restoring stability and maintaining natural balance.17 Traditionally, the absolute indications for lumbar interbody surgery were lumbar spondylolisthesis, severe scoliosis, spinal tuberculosis, and fractures, some of which have gradually shifted more towards relative indications such as back pain, degenerative disc disease, and spinal stenosis.18
2.1 The genesis of interbody fusion techniques
In 1933, Capener pioneered the anterior fusion of the L5 (fifth lumbar vertebra) and S1 (first sacral vertebra) using a tibial graft peg for treating spondylolisthesis. Despite its biomechanical advantages, this approach faced resistance among clinicians due to its invasiveness.19 A decade later, Cloward performed the first posterior lumbar interbody fusion (PLIF) without posterior instrumentation; a procedure involving the removal of the intervertebral disc, including the cartilaginous endplates, through a partial bilateral laminectomy and essentially a complete facetectomy. Subsequently, Cloward performed autologous implantation, placing three or more large full-thickness bone grafts obtained from the iliac crest.20 Historically, bi- and tri-cortical structural bone grafts, such as autologous bone from the iliac crest or fibula, and allografts, were implanted in the intervertebral space to stabilise the spine and to achieve spinal fusion. However, these alternatives are associated with relevant donor site morbidity and insufficient spinal stability.21–25 Spinal fusion with bone graft alone is linked to a notable incidence of autograft or allograft collapse, eventually leading to pseudarthrosis.26 To address these complications, additional posterior stabilisation with pedicular screws interconnected with rods gained popularity in the 1980s to achieve spinal fusion (posterolateral instrumentation/fusion).
Current surgical techniques of spinal fusion rely heavily on autologous bone graft (ABG) or bone graft substitutes to achieve adequate bone healing and solid fusion.27 ABG remains crucial for stabilisation due to its inherent properties of osteogenic potential, osteoinductivity, and osteoconductivity.28–30 Modern practices involve morselising ABG to enhance its osteogenic potential and facilitate its integration within the intervertebral space and hollow regions of cages. Furthermore, ABG is placed around the screw–rod construct to facilitate the posterior fusion of adjacent bone surfaces.31 The iliac crest is frequently used as a source for harvesting cancellous ABG; however, its application is constrained by several factors. These include the restricted size and volume of obtainable bone grafts, donor site morbidity that can lead to persistent pain in up to 30% of patients post-harvesting,32–34 and the presence of a limited quantity of viable and biologically active cells within the graft.35 Additionally, the number of stem cells present in the graft notably decreases after the age of 55.36 The anatomical site from which AGB is harvested – be it the iliac crest, lamina, or during decortication – affects the graft's composition and subsequently influences its regenerative capacities.37–39
Nonetheless, rates of non-union after lumbar spine fusions range from 5% to 35%40 and are associated with the unsatisfactory resolution of clinical symptoms.41 Spine surgery revision rates of 10% to 20% pose a significant challenge for patients, surgeons, and the health care system in general.4–7 Consequently, a rigorous evaluation of the current treatment approach is warranted to support future preclinical and clinical research aimed at developing more sophisticated techniques leading towards more effective spinal fusion.
2.2 The introduction of interbody spacers
The application of interbody spacers, or ‘cages’, was first described by Bagby42 in the context of treating equine wobblers disease, involving arthrodesis through the distraction–compression method using a stainless steel cylindrical, fenestrated implant. Adapted for human use, the Bagby and Kuslich method of interbody stabilisation for chronic discogenic lower back pain incorporated the “Bagby Basket” with ABG rather than relying on ABG alone. This presented good fusion rates for lumber interbody fusion.43 Bagby and Kuslich (BAK) cages (SpineTech, Minneapolis, MN) achieved a 20% fusion success at six months with the addition of autograft, and reached a 100% fusion rate when it was combined with recombinant human bone morphogenetic protein-2 (rhBMP-2).44 Thus, using interbody cages provided further stability and fusion by restoring disk height and neuroforaminal volume.45,46
Throughout the 1990s, spinal interbody fusion with conventional metallic cages, primarily titanium–aluminium–vanadium (Ti6Al4V or Ti), gained widespread commercialisation. While titanium offers durability, strength, osteoconductivity, and resistance to corrosion, its high elastic modulus introduces complications in the form of stress-shielding, endplate trauma, adjacent segment disease, and cage subsidence.47–49 In the early 2000s, the use of polyetheretherketone (PEEK) cages gained in prominence as it has a favourable elastic modulus akin to bone, which typically aids in lowering subsidence rates.50 However, PEEK's bioinert nature diminishes its osteointegrative capacity, and challenges such as the need for greater endplate preparation and issues with over distraction compromise its effectiveness.51
The historical progression from traditional metallic cages to the adoption of PEEK marked a significant shift in addressing biomechanical challenges. While each material brings its specific advantages, the pursuit for an ideal balance between strength, osteoconduction, and biocompatibility spurred further innovations. The 2010s witnessed the introduction of hybrid materials and surface modifications, giving rise to the development of Ti-coated PEEK (Ti-PEEK) composite materials. This innovation amalgamated PEEK's mechanical advantages with the desired biocompatibility characteristics of Ti. The increasing popularity of additive manufacturing from the late 2010s allowed the creation of biomimetic structures resembling the porosity and biomechanical properties of cortical bone. This technological leap has paved the way for interlayer-mediated cages with osteoconductive coatings and topographical modifications, aiming to optimise the cages’ osteoinductive, osteoconductive, and osteogenic properties. While larger clinical trials are needed to validate the efficacy of these devices, in vitro, in vivo, and initial clinical trials have demonstrated their viability.52–58 However, primary concerns such as delamination and inflammatory responses due to wear remain significant challenges.59–61
Despite advancements in selective laser sintering (SLS) manufacturing of titanium implants, which improve the biomimetic properties and biomechanical performance of Ti spinal cages, PEEK remains the most cited material for spinal surgery.62 Fused deposition modelling (FDM) of PEEK is more cost-effective compared to SLS, which may contribute to its continued prevalence in spinal surgery.
2.3 Synergies in posterior lumbar stabilisation
In the late 20th century, the advent of the standardised use of posterior spinal instruments led to a transition to circumferential (360°) fusion in clinical practice, which further improved clinical outcomes and increased the robustness of results.63,64 Therefore, it can be deduced that the success of lumbar spinal fusion hinges on the synergistic interplay of various components – namely, spinal instrumentation, intervertebral body devices (cages), and ABG or bone graft substitutes (Fig. 2). To achieve an ‘ideal’ lumbar stabilisation model, characterised by a biomimetic approach mirroring the structure and properties of natural bone extracellular matrix (ECM), a comprehensive understanding of these elements is imperative. This analysis delves into the individual roles of these components, offering a concise overview coupled with critical discussions on advancing research.
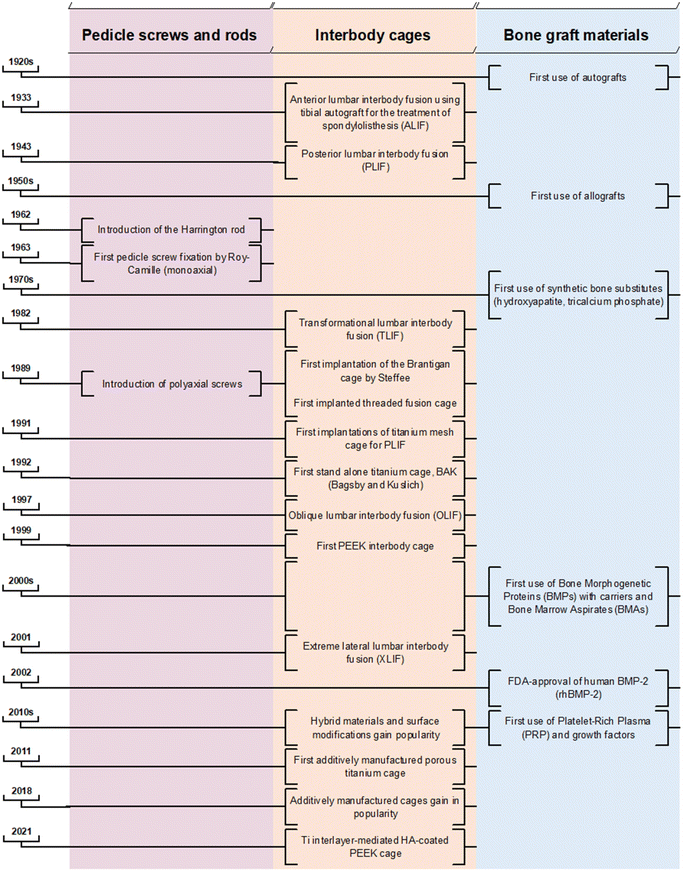 |
| Fig. 2 Chronology highlighting major milestones in clinical lumbar spine surgery, tracing the evolution of bone graft materials, interbody cages, and pedicle screws and rods, with a specific focus on advancements in interbody spinal fusion. | |
3. Anterior/posterior lumbar fusion: 360° spinal stabilisation
3.1 Pedicle screws
Pedicle screw fixation was first introduced in the context of thoracolumbar segmental fixation in 1963 by Roy-Camille.65 Initially designed for fractures, pedicle screws have since evolved to address various spinal conditions, including tumours, spondylolisthesis, fractures, and malunions. The initial pedicle screw design featured a fixed-headed, monoaxial approach, placed sagittally through the pedicles and articular processes, coupled with plates.66 The progression of pedicle screw design includes the integration of patient-specific contoured rods, marking a significant advancement in spinal deformity correction.67 Positioned in the most structurally robust region of the vertebrae, this instrumentation plays a pivotal role in achieving immediate immobilisation, enhancing the bone–screw interface, and thereby improving the biomechanical performance of the screws, allowing for optimal leverage to exert higher corrective forces on the deformed spine.
However, the conventional monoaxial screw design has limitations in manoeuvrability, presenting challenges in aligning the screw with the rod head saddle. To address this, Harms introduced the polyaxial pedicle screw in 1989, aiming to facilitate a more straightforward coupling of fixation points with rods. While polyaxial pedicle screw fixation has demonstrated an association with less adjacent segment degeneration, likely attributed to lower von Mises stress in screws and reduced intradiscal pressure in the adjacent segment,68 its enhanced manoeuvrability comes at the expense some construct strength, making polyaxial screws more susceptible to fatigue failure or breakage.
Despite the widespread adoption of polyaxial screws, the use of monoaxial screws or a combination of both types persists, aiming to optimise biomechanical performance in fixation constructs. For instance, in the treatment of thoracolumbar fractured vertebrae, monoaxial pedicle screws may be preferable due to their enhanced leveraging effect, providing increased stability during flexion and extension of the spine.68,69 This choice also contributes to improved uplift and restoration of the collapsed superior endplate. Similarly, hybrid constructs, incorporating both mono- and poly-axial screws or uniplanar screws, allow freedom of movement in assembly without sacrificing construct stiffness in the sagittal plane.70,71
3.1.1 Anchor and tip types.
The integrity of the bone–screw interface plays a pivotal role in ensuring the stability and pull-out strength of pedicle screws. Fig. 3 illustrates the diverse anchor and thread types that have been developed to enhance this metric. Pedicle screws are subjected to significant cyclic axial and lateral forces. The strength of these screws is directly proportional to the cube of the minor core diameter, with larger diameters offering greater resistance to screw bending or breakage. The pull-out resistance of the screw is influenced by factors such as the major (outside) diameter, thread depth, pitch, and shape. Notably, the thread pitch determines the volume of bone between the threads, which is directly proportional to pull-out resistance. The selection of inadequate anchor or thread types can lead to complications such as screw loosening or breakage. Many pedicle screws are cannulated to facilitate accurate implantation with a guide wire and features a standard threaded anchor. Given that lower bone density correlates with decreased strength in the bone–screw interface, leading to loosening and pull-out – particularly observed in elderly patients suffering from osteoporosis – various other anchor and screw types have been developed.72–74 These aim to increase the surface area attached to the cortical bone, thus enhancing interface stability.
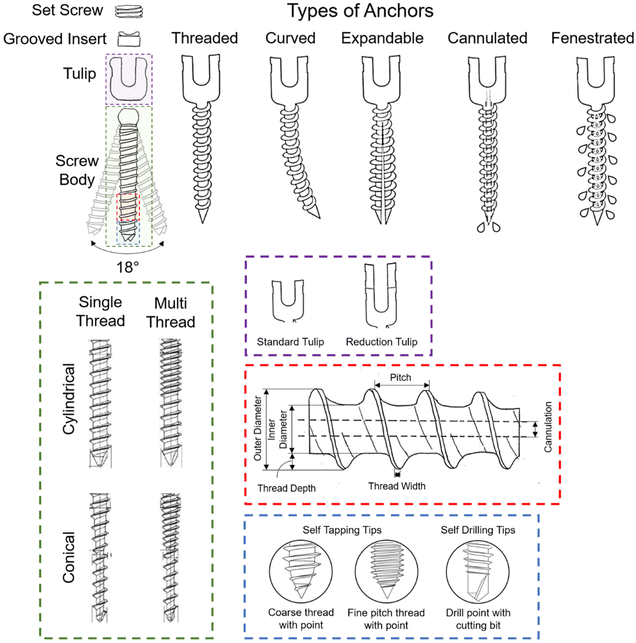 |
| Fig. 3 The various features found in commercial pedicle screws, including different anchor, thread, and tip types, are designed to influence the screw's biomechanical performance and integration with bone. Adapted from ref. 72 with permission from Springer Nature, copyright (2022). Adapted from ref. 73 with permission from MDPI AG, copyright (2022). | |
In the context of screw types, tapping and non-tapping screws play distinct roles. Tapping screws, through their design, create threads in the bone, providing a pre-formed path for the screw to follow during insertion. This process reduces the risk of generating excessive heat, preserving bone integrity. Non-tapping screws, conversely, lack pre-formed threads and engage directly with the bone as they are inserted. While non-tapping screws offer advantages in terms of reduced thermal damage, tapping screws may be preferred in certain scenarios for their simplicity and efficiency. Multithreaded screws, for instance, have been employed to generate locking forces. In this design, the screw interfaces between proximal-cortical, middle-cancellous, and distal-cancellous screw–bone segments are compressed against each other. This approach is typically complemented by a conical or dual-core screw type, enhancing load-bearing capacity, potentially minimising screw breakages,75,76 and ensuring optimal contact between the screw and bone surfaces. The combination of these design features contributes to the overall stability and longevity of the pedicle screw fixation in challenging bone conditions.
3.1.2 Biomaterials.
Since screws redirect force through the vertebral bodies they are commonly made of strong as well as bioinert material such as Ti6Al4V. Complications associated with pedicle screw placement which can affect bone healing include loosening, pull-out, and screw breakage. Pull-out resistance of screws is affected by the bone mineral density, the screw insertion technique and factors directly influencing the screw such as metal properties, and geometry. The holding power is further improved when self-tapping screws are used, however only when multiple time insertion is avoided. To improve screw performance coating and doping with various materials such as hydroxyapatite (HA), calcium phosphate (CaP), polymethylmethacrylate bone cement (PMMA-BC), ECM, tantalum (Ta), and Ti plasma spray have been investigated.77–81 In preclinical animal models coating of Ti6Al4V screws with HA was shown to improve resistance against pull-out force77 and bone-to-implant contact.78 When comparing HA, CaP, and PMMA-BC against control only PMMA-BC showed increased pull-out strength while it is noteworthy that rigid and solidified cement structure limits any post insertion modification.79 Further, thin Ta coating of Ti6Al4V pedicle screws exerted an inhibitory effect on osteoclasts and promoted trabecular bone growth in vivo.80 Coating of pedicle screws with the combination of ECM and HA improved pull-out strength compared to uncoated screws or coating with HA or ECM alone.81 However, the translation into clinical use is scarce and future clinical studies are necessary to define the role of specific screw coatings to improve pull-out force and spinal stability.
3.2 Spinal rods
Spinal rods have been routinely used for treating spinal deformities since the introduction of the “Harrington rod” for the treatment of scoliosis in 1962.82 In recent years, they have emerged as a standard and indispensable component of lumbar interbody fusions.83,84 These rods play an essential role in load distribution during instrumented (posterior) spinal arthrodesis. Strategically positioned along each spinal process side and affixed to the pedicle screws, these implants are manually contoured to a specific fit during surgery. They feature a diverse range of diameters that provide varying levels of stiffness, ensuring an optimal fit to the patient's individual spinal pathology. Due to its use in conjunction with other spinal implants such as screws and side-to-side connectors, spinal rods ideally establish a controlled environment that evenly distributes loads across the vertebral segment(s). This promotes a conducive setting for bone fusion. Therefore, the selection of biomaterials for spinal rods should prioritise stability and stiffness required by the individual patient, as these factors are crucial for immobilising interbody vertebrae, fostering bone fusion, and safeguarding bone graft integrity.
3.2.1 Biomechanical stability.
Metallic-based rod systems, mainly composed of stainless steel, have traditionally been crucial in spinal instrumentation due to their inherent stability and stiffness.85 This rigidity is essential for spinal immobilisation, facilitating interbody fusion and ABG integration stabilising the posterior screw–rod constructs. However, the use of such materials, while effective in achieving successful arthrodesis, presents challenges. The use of supraphysiological rigid materials may cause stress shielding and improper load sharing, which can lead to issues such as adjacent segment disease.86 This is due to the excessive stress imposed on neighbouring vertebrae. In cases where there is excessive load sharing, the mechanical load is distributed between the implant and surrounding vertebral bodies, resulting in a reduction in load through the bypassed vertebral body. As a living tissue, bone requires mechanical loading to maintain its density and strength. Therefore, the reduced mechanical strain on the bone of the bypassed vertebral body, while promoting fusion and immediate stability, can lead to bone mineral loss over time.86 This phenomenon is known as disuse osteoporosis or bone demineralisation.
To address these concerns, contemporary dynamic and flexible alternatives such as porous Ti and titanium alloys (pTi and Ti6Al4V), or polymers like PEEK, have been favoured for their superior biomechanical properties, closer resemblance to Young's modulus of bone, and enhanced biocompatibility.87,88 However, the semi-rigidity of polymer alternatives has led to its own suite of complications, including screw loosening, infection, back and leg pain, and endplate vertebral fracture. PEEK rods are a popular choice for semirigid rods due to their comparable stability to Ti rods89 and are associated with lower incidence of adjacent segment disease.90 Theoretical advantages include improved biological compliance, elasticity, and radiolucency. However, some studies have reported increased rates of pseudarthrosis and early reoperations due to an unstable screw interface.91 Future research could investigate materials with matching Young's Moduli, high bending strength, and high tensile strength. In particular, beta-type Ti-molybdenum and oxygen-modified beta-type Ti-chromium alloys possess desirable mechanical properties to mitigate stress-shielding and have good cytocompatibility.83,92,93 Future in vivo studies are required to test the efficacy of these materials for spinal rod applications.
Furthermore, the feasibility of biodegradability for rods in lumbar spinal fusions remains uncertain.83,94 Tsuang et al.94 demonstrated in vitro that biodegradable rods can withstand comparable dynamic compression cycles under axial load to standard Ti rods. However, they observed a 20% and 80% decrease in Young's modulus after six and 12 months, respectively, which could potentially impair spinal stability in vivo. Future preclinical large animal studies may shed light on the applicability of biodegradable rods for posterior lumbar fusion before clinical trials may be conceived.
3.2.2 Notch effect.
Despite advancements in material choices and surgical techniques, it is important to note that the process of rod contouring, typically performed with a French Bender, introduces notch points that compromise the rod's durability by imparting marks and weaknesses95 (Fig. 4). This is otherwise known as notch sensitivity.96 Therefore, in addition to the screw–rod junction these notch-points are susceptible to rod fatigue, fractures, and significant deformation.92 Recent advancements in preoperative planning software have led to the development of patient-specific spinal rods, which address the limitations of traditional contouring methods.97 Unlike conventionally contoured rods, these rods do not require on-site shaping during surgery. It has the potential to reduce surgical time, minimise the occurrence of rod microfractures, increase fatigue life, and lead to fewer mechanical complications. The integration of patient-specific spinal rods is a promising advancement in arthrodesis procedures, potentially reducing proximal junctional failure.98 This showcases a step towards enhancing the overall durability and performance of spinal instrumentation.
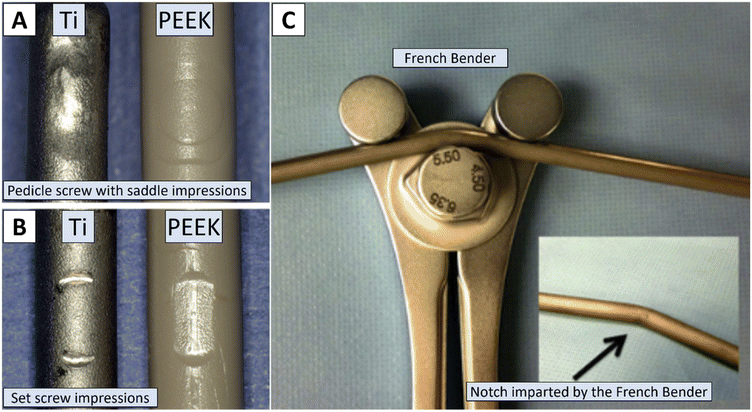 |
| Fig. 4 Surface defects and curvature deformation of the spinal rod can compromise its durability. Panels A and B show the surface impressions caused by pedicle screw fastening, while the black arrow in panel C indicates the notch imparted by the French bender, an instrument commonly used to contour the spinal rod to the patient's spine. These defects can significantly affect the rod's biomechanical performance and longevity. Reproduced from ref. 99 with permission from Elsevier, copyright (2019). Reproduced from ref. 86 with permission from Elsevier, copyright (2013). | |
3.2.3 Corrosion resistance and biocompatibility.
Additional research has explored materials such as nickel (Ni), nitinol (NiTi), and cobalt–chromium alloys (CoCr). These metals are biocompatible due to the stable oxide layer that protects against corrosion.100 Cobalt–chromium rods, which are more rigid than stainless steel rods, have demonstrated potential in treating adolescent idiopathic scoliosis when compared to Ti rods.101,102 Furthermore, while theoretical drawbacks such as increased artefacts on magnetic resonance imaging (MRI) compared to Ti have been noted, clinical studies have found no impairment of the spinal canal or neural elements from such CoCr artefacts.103 Nitinol does not evoke an inflammatory response from the lymph nodes or other organs104 and exerts shape recovery forces, making it a valuable material for scoliosis correction. Additionally, NiTi rods have been used for spinal instrumentation due to their wear resistance comparable to CoCr and 100 times higher compared with Ti.105 However, its lower Young's modulus compared to Ti or stainless steel rods, and higher costs make this material less attractive for spine surgery, particularly lumbar spinal fusion.106 Incorporating materials like CoCr and NiTi in spinal instrumentation offers promising alternatives to stainless steel or titanium, providing both biocompatibility and mechanical advantages. However, careful consideration of clinical implications, keeping in mind the cost-to-benefit ratio, is essential for their potential application in lumbar spinal fusion.107
3.3 Spinal cages for lumbar interbody fusion
The selection of an interbody cage in lumbar interbody fusion is intrinsically linked to the chosen surgical approach, aligning to the geometry and material properties of the bony endplate. Five primary approaches have been developed to address the complexities of spinal disorders – Posterior Lumbar Interbody Fusion (PLIF), Transforaminal Lumbar Interbody Fusion (TLIF), Anterior Lumbar Interbody Fusion (ALIF), extreme Lateral Lumbar Interbody Fusion (XLIF), and Oblique Lumbar Interbody Fusion (OLIF) as illustrated in Fig. 5. Notably, there is no conclusive evidence indicating the superiority of one approach over another,108 however, inherent advantages and disadvantages may lean towards specific indications for particular lumbar spine pathologies.84,109
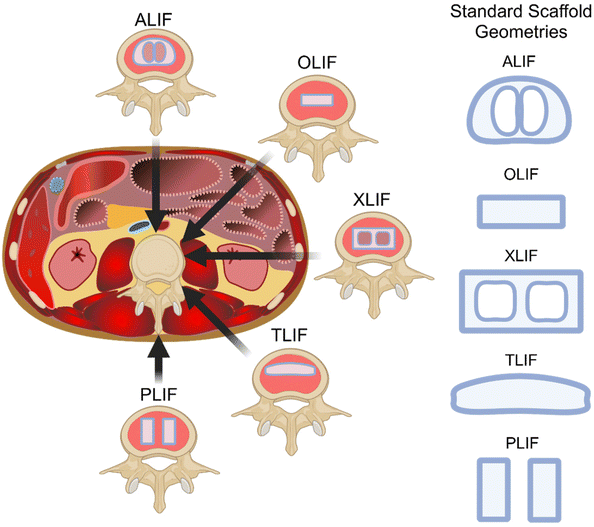 |
| Fig. 5 Surgical approaches for lumbar interbody fusion and their respective interbody cage designs. A transverse section through the abdomen highlights the anatomical structures encountered in each surgical approach, illustrating potential challenges and risks for different types of surgeries. Figure created with BioRender.com. | |
PLIF employs dual ovoid-shaped spacers packed with bone graft, offering comprehensive decompression and fusion capabilities through a posterior approach. With relatively low complications, it avoids vascular and neurological risks associated with anterior approaches, providing a single-incision option for bilateral decompression and interbody fusion.108 TLIF utilises a single kidney-shaped implant supplemented with bone graft for interbody fusion, avoiding anterior exposure and associated complications such as vascular and abdominal wall issues. Due to the unilateral hemilaminectomy (compared to bilateral hemilaminectomy with PLIF), TLIF is in theory superior to PLIF in terms of biomechanical stability, but a certain learning curve is necessary as significant muscle retraction and dissection can occur, which in turn can impact postoperative pain and rehabilitation.108 ALIF implants a single, wedge-shaped cage through an anterior approach, preserving posterior spinal elements and offering a viable option for revision surgery. However, it presents challenges related to intraabdominal and vascular complications, and lower fusion rates compared to PLIF and TLIF due to limitations in using local bone graft.108 XLIF uses a single ovoid implant through a transpsoas approach, providing good anterior column support with advantages such as avoiding major vascular manipulation. However, it has exposure limitations and risks damaging neural structures within the psoas muscle, leading to potential sensory and motor deficits.108 OLIF, like XLIF, places a single ovoid spacer without traversing the psoas muscle, making it suitable for various degenerative conditions and deformity corrections. While allowing aggressive deformity correction and achieving high fusion rates, OLIF introduces potential risks such as sympathetic dysfunction and vascular injury.110
The gold standard of a solid 360° interbody fusion (interbody spacer plus posterior pedicle screw-and-rod instrumentation)111 includes a radical debridement of the (infected) disc to facilitate intervertebral fusion using either interbody cages and/or ABG with or without additional decompression of the spinal canal.112–115 Due to its high corrosion resistance and biocompatibility Ti and its alloys are successfully employed as artificial implants, such as interbody cages, in orthopaedic surgery.116 Nevertheless, the ongoing risk of infection, challenges in radiographically assessing fusion, and adjacent segment diseases frequently linked to its greater stiffness compared to human bone have led to the exploration of cages made from alternative materials.117,118 The underlying causes of non-union and cage subsidence are multifactorial, including surgical techniques and bone quality,119–121 but the bone–hardware interface's ability to act as a biomechanical unit against axial loading stress is critical in preventing subsidence.122 Laboratory studies by123 demonstrate that stress shielding and subsidence can be mitigated by developing implants that mimic the properties of bone. Therefore, intervertebral body implants manufactured from PEEK are regularly used due to the polymer's radiolucency, proven biocompatibility, and an elastic modulus of 4.0 GPa, which closely approximates tht of cortical bone (4.89 GPa) compared to Ti (105 GPa).54,124,125 Furthermore, technological advances allowed for the development of Ti-PEEK composite materials combining the mechanical advantages of PEEK with the desired biocompatible characteristics of Ti.
Moreover, current research focuses on a variety of technologies for the production of antibiotic and non-antibiotic antimicrobial pro-osteogenic implants, ranging from inherently antimicrobial implants based on the effects of chemistry or topography to the application of antimicrobial metal ions and oxides, polymers or peptides.126 In line, preclinical and clinical outcomes in the last decennia stress the importance of implant designs in orthopaedics aiming to improve implant characteristics, such as Young's modulus, compression strength, biocompatibility, and surface topography.127–129 Such features are highlighted by the ‘ideal’ interbody cage characteristics in Fig. 6.
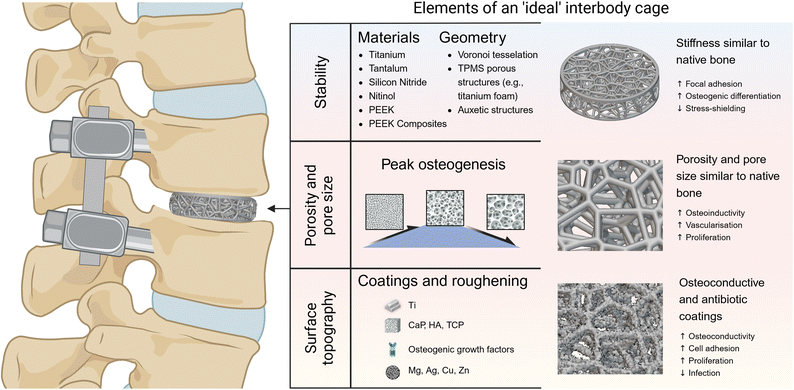 |
| Fig. 6 The essential elements of an ‘ideal’ lumbar interbody cage design include stability, porosity and pore size, and surface topography. The choice of materials (e.g., titanium, PEEK, composites) and geometry (e.g., Voronoi tessellation, TPMS, auxetic structures) helps to biomimetically replicate the structure and stiffness of native bone, enhancing stability and reducing stress shielding.127–129 Optimal porosity and pore size are crucial for osteoinductivity, vascularization, and cell proliferation.153–156 Changes to surface topography and coatings can help to improve osteoconductivity, cell adhesion, and reduce infection risk through antibiotic coatings.126 Figure created with BioRender.com. Scaffold created with nTop, Release 4.21.1, nTop Inc., https://ntop.com. | |
3.3.1 Titanium cages.
Titanium cages have high mechanical stability and can be manufactured in a variety of designs and surface structures. The merits of Ti interbody implants include their high mechanical strength under physiological loads, low density, high corrosion resistance, and good biocompatibility.60,130,131 Titanium and its alloys are widely utilised in orthopaedic surgery due to their resistance to corrosion and biocompatibility.116 While segmental spinal fusion effectively alleviates pain by addressing spinal instability, conventional metallic cages pose complications, including increased adjacent level (disc) disease due to its high stiffness, causing stress shielding, implant migration or subsidence, device-related osteopenia and imaging artefacts.47–49 Particularly, postoperative follow-up for bone healing determination is often impaired by radiopaque metallic cages, impeding the visualisation of bony fusion at the graft site.8,132–134 Superior biological response including the promotion of bone formation in vitro has been observed for intervertebral body implants manufactured out of Ti alloy compared to PEEK.59,130 Preclinical large animal studies comparing Ti and PEEK devices in lumbar fusion models show comparable patient range of motion and fusion rates. Although titanium cages exhibit higher subsidence rates than PEEK cages, clinical outcome variables remain insignificantly different.50,135 However, in the osteoporotic spine, bone stress concentration and absorption may lead to the instability or sinking of Ti (mesh) cages.136
3.3.1.1 Surface modifications.
Enhancing osteointegration in cages can be achieved through surface modifications, particularly by incorporating rough and porous Ti surfaces.137–139 Surface roughness, influenced by techniques like plasma spraying, electron beam (e-beam) melting, sandblasting/acid treatment, and 3D printing, positively induce cell adhesion and proliferation. Roughening a smooth surface has been shown to promote osteointegration by increasing osseous tissue apposition, favouring epithelial attachment to the implant, especially on Ti surfaces.140–142 Coating techniques involve adding a calcium-phosphate-based mineral film similar to bone mineral,143–146 and incorporating osteogenic growth factors to mineral coatings as delivery vehicles.147 Mineral coatings with “bone-like”-films significantly enhance the osteoconductivity and osteoinductivity of orthopaedic implant materials.148,149 For instance, Ti implants subjected to sandblasting with alumina grit (0.25–0.50 mm) followed by etching in HCl/H2SO4 acid bath have shown increased bone anchorage in a large animal study.150 Pelletier et al.134 observed in their large animal lumbar fusion model that treated Ti endplates had greater bone apposition compared to polished internal Ti surfaces or PEEK. Moreover, rough Ti alloy compared to smooth Ti alloy or PEEK was shown to stimulate cells in creating an osteogenic–angiogenic microenvironment in vitro. This includes the expression of integrins crucial for collagen recognition and enhanced osteoblast maturation.59,151 Microroughened Ti surfaces demonstrate increased osteoblast differentiation and protein production, associated with bone formation and decreased bone resorption.152 Further, the bioactivity of porous Ti cages can be enhanced through chemical and thermal treatments.157 Modifications of Ti surfaces by addition of nanoparticles such as Ti oxide (TiO2) and zirconia (ZrO2) are also possible. By adjusting the nanostructure of the surface of Ti implants in terms of titanium dioxide (TiO(2)) nanotubes, Bjursten et al.158 observed improved bone bonding strength, greater bone–implant contact area, new bone formation, and calcium and phosphorus levels on the nanotube surfaces compared with TiO(2) grit blasted surfaces. Thereby, these surface technologies potentially increase the rate of fusion by fostering osteointegration at interfaces of both endplate–implant and bone graft–implant. However, surface modification of blasting or acid etching of Ti implants and porous coatings may cause crack initiation potentially influencing its fatigue and bending strength.159,160
In recent decades, orthopaedic implants, particularly Ti and Ti alloys, are successively shifting from the early premise of mechanical strength to biocompatibility, fast osteointegration to multifunctional properties including the function of antibacterial actions as well as modulation of inflammation. Instrumentation failure related to microbial infections of Ti implants are a heavy burden on patient health and healthcare costs.161 Titanium surfaces may be rendered antibacterial to decrease bacterial infections by surface modification and coatings (chemical or physical).162 Gentamicin-coated tibial nails have already been successfully used in patients with high infection risk163 and in vitro cytocompatibility and antimicrobial activity has been shown for hybrid surfaces of porous Ti structure, silver particles in the Ti dioxide layer, and gentamicin-loading.164
3.3.1.2 Porous titanium cages.
Recent developments in fabrication techniques for selective laser melting, and e-beam laser melting allow for the wide use of Ti.165 Porous implants, compared to solid metal implants, are more lightweight, offer larger contact surfaces, and may possess mechanical properties that more closely resemble human bone. Furthermore, advanced additive manufacturing techniques enable the printing of porous metallic scaffolds that more accurately mimic bone's complex 3D inhomogeneous structure, featuring intricate details from the macro- to the nano-scale.166,167 When selecting pore shape, size, and overall porosity, it is crucial to carefully consider mechanical properties and intended functionality, as these factors significantly influence the mechanical behaviour of porous metallic biomaterials.154 Additionally, they affect biological performance, including cell adhesion and proliferation, nutrient transport, and the osteointegration of the implant.153,155 Porous Ti cages with a porosity of 60% to 70%, pore sizes ranging from 250 to 750 μm, and pore interconnectivity greater than 99% can be fabricated with high mechanical strength.156
In silico analyses suggest that porous cages with a porosity between 65% and 80% may offer beneficial biomechanical effects compared to solid Ti or PEEK cages in lumbar interbody fusion.168,169 Preclinical large animal studies have demonstrated good biocompatibility and osteointegration of 3D-printed pTi cages.131,170–172 For instance, these implants have shown superior push-out strength compared to PEEK or allograft materials in a preclinical animal study.131 Notably, in an ovine model, 3D-printed porous Ti interbody cages were associated with enhanced bone ingrowth, significantly reduced range of motion, and improved construct stiffness compared to PEEK and plasma sprayed pTi-coated PEEK for lumbar fusion.170 Furthermore, the absence of an interface between different materials with different moduli solid 3D-printed metal cages is theorised to reduce the risk of delamination.170 Initial clinical evidence indicates that porous Ti cages yield superior radiographic and clinical outcomes compared to PEEK cages, underscoring their successful translation from preclinical to clinical settings.156,173,174 Fujibayashi et al.156 implanted porous bioactive Ti cages with autograft in a TLIF technique in five patients, achieving successful bony fusion within six months and a significant improvement in clinical parameters in all cases. Subsidence rates of 6.7% per surgery and 3.4% per implant for 3D-printed Ti cage (Modulus; NuVasive, San Diego, CA) implanted via minimally invasive transpsoas XLIF were reported by Krafft et al.173 which are lower compared to previously reported subsidence rates of PEEK cages implanted via minimally invasive XLIF (10.0 to 16.1%).120,175,176 By comparing Ti-coated PEEK cages (ProSpace XP; Aesculap AG, Tuttlingen, Germany; pore size, 50–200 μm; mean porosity, 37.3%; elastic modulus, 4.6 GPa) in 34 patients with 3D porous Ti alloy cages (Tritanium PL; Stryker, Kalamazoo, MI; pore size, 100–700 mm; mean porosity, 60%; elastic modulus, 6.2 GPa) in 29 patients, Makino et al.174 observed similar fusion rates and patient-reported outcomes. However, the Ti-coated PEEK cage group exhibited a higher incidence and severity of postoperative vertebral endplate cyst formation. Thus, long-term prospective randomised trials comparing these with conventional cage materials and designs are necessary to confirm the radiographic and clinical superiority of the promising short-term results associated with porous Ti cages.167
3.3.2 PEEK cages.
Due to their inert nature in biological environments, ease of processing, and ability to provide mechanical support, many non-degradable synthetic polymers have been extensively investigated for biomedical applications.177 While synthetic polymers generally resist hydrolytic, oxidative, and other degradation mechanisms, PEEK stands out as a semi-crystalline thermoplastic with exceptional mechanical and chemical resistance properties. These characteristics confer resistance to post-irradiation degradation, allowing PEEK to be sterilised by gamma and e-beam irradiation while maintaining its mechanical strength over extended periods in dynamic environments.177
PEEK's elastic modulus is nearly identical to human cortical bone's modulus, particularly when reinforced using carbon, which might lead to advantages in load sharing and stress distribution and, thereby, reduce cage subsidence.50 Additionally, compared to Ti, PEEK has shown significantly lower stress compression strength (2.5 times weaker).178 Concerns of synovitis and the lymphatic spread of non-absorbable polymer debris has been raised in early studies.179–181 However, multiple subsequent studies on local and systemic toxicity showed that PEEK does not illicit adverse tissue reactions.182–185 After its first development in 1978 it was approved a year later by the FDA for intervertebral spacers124 and its good mechanical properties and chemical resistance resulted in its wide use.53 Particularly, due to its radiolucency, less artefacts on computed tomography (CT), and MRI scans occur, which allow for more appropriate visualisation of possible migration (i.e., radiolucency) and the bony fusion status.125,178 However, lower support for osteogenic tissue and lower level of bone integration has been reported for PEEK compared to Ti.50 For instance, single-level interbody fusion rates of only 71% have been observed with PEEK cages augmented with autograft in ovine models.186 This reduced fusion rate is partly attributed to the formation of a fibrous layer at the bone–implant interface, which is inhibited due to PEEK's chemically inert and hydrophobic surface.187 For instance often peri-implant fibrous tissue is formed on the bone–implant interface, potentially due to a hydrophobic PEEK surface that is associated with production of inflammatory chemokines188 and inhibitory effects on osteoblastic differentiation of progenitor cells.60 Eventually this reduced capacity for osteointegration and achieving a solid bone-cage fusion increases the risk of long-term sequelae such as micromotion, which in turn is associated with implant failure and pseudarthrosis.189,190
Notably, clinical studies reported no difference in clinical outcomes between PEEK, Ti, and carbon fibre cages.50,191,192 Thus, recommendations for the most effective interbody fusion material are limited due to the similarity in clinical and radiographic outcomes among these materials. Combined Ti/PEEK spinal fusion cages have shown comparable safety, efficacy, fusion rates, and clinical outcomes to standard PEEK cages.193 Therefore, PEEK cages are primarily used when their radiolucent properties are essential, such as in cases requiring radiotherapy or following spinal infections.
3.3.2.1 PEEK composites and surface modifications.
To enhance the biological interaction of otherwise inert materials and promote tissue integration, various additives and surface modification techniques are employed. For instance, adding metals like Ti to PEEK has been shown to improve its performance in spinal fusion applications.194 Methods to create Ti-PEEK composites include compression moulding to construct Ti endplates around a PEEK core, or surface coatings via techniques such as e-beam deposition, plasma spray coating, or direct metal laser sintering.52 Surface coating of PEEK with a pure Ti layer using e-beam deposition has demonstrated improved cell survival, higher alkaline phosphatase activity, and a greater bone-in-contact ratio compared to uncoated PEEK implants.53 However, plasma spraying and vapor deposition methods for coating PEEK cages can lead to surface cracking due to weak interfaces, potentially impacting implant performance.59–61 Plasma-sprayed Ti creates a rough and porous surface that may enhance osteointegration while providing X-ray translucency and reducing stress shielding. This process of rough surface coating potentially inhibits implant migration while its porous texture provides optimal support for osteointegration.130,195–197 Ti-coated PEEK (PlasmaporeXP®; Aesculap AG, Tuttlingen, Germany) showed increased early bone formation activity and improved bone–implant interface compared to PEEK alone (PEEK-Optima™; Invibio, Lancashire, UK) in vitro as well as in vivo when implanted orthotopically.54 However, plasma spraying results in a relatively thick Ti layer (ranging from 13.4 to 70 μm) which may raise concerns about delamination198,199 and denaturation of PEEK due to the high temperatures used in the coating process. Delamination can result in wear debris, which may cause local inflammatory reactions, although specific particle concentration thresholds for adverse postoperative effects are not yet defined.200 Various animal and clinical studies reported local inflammatory reactions of Ti-related wear debris which may cause biological reactions in the human body201–203 but possible cut-off values for concentrations of these particles causing consequences in terms of postoperative complications are yet to be defined.200
Authors of smaller clinical trials conclude based on their results that Ti-coated PEEK-cages are safe and efficacious.55–57 Rickert et al.57 observed identical clinical outcomes and a high rate of fusion (thin slice CT at three months and functional radiography at 12 months) in both groups of PEEK comparing to Ti-coated PEEK cages in a pilot trail of 40 patients. A prospective randomised trial including 60 patients with six and 12 months follow-up points for fusion rates (plain X-rays and CT scans) and clinical outcomes of PLIF surgery with Ti-coated PEEK cages (coated Wave®, Advanced Medical Technologies AG, Medtronic, Minneapolis, Minnesota, USA) versus uncoated PEEK cages (Wave®, Advanced Medical Technologies AG) performed for 24 months (visual analogue scale, VAS; Oswestry Disability Index score, ODI; EQ-5D) showed similar clinical and radiological results.204 A vacuum plasma spray technique for coating of the upper and lower surfaces of the cages was used, melting pure Ti particles in a hot plasma beam and vaporising the droplets onto the PEEK cage which resulted in thickness of the Ti coating of 25–160 μm and a micro-roughness of at least 50 μm.204 Another multicentre, randomised study with 149 patients (PLIF and TLIF) followed up for 12 months showed no difference in spinal fusion rate.58 However, Ti-coated PEEK cages compared to PEEK cages were associated with better bone fusion at six months after surgery and the authors claim thereby an earlier return to work for the patients.58
Alternative surface coatings include CaP, HA, metallic oxides, or polymers.205–207 Methods for applying these coatings include drop-casting, dipping, spraying, and polyelectrolytic deposition.205 Extensive research on its challenges and approaches208 and release kinetics as well as methods of encapsulation and protection of incorporated factors209 have been published. Additionally, release of incorporated factors may occur in response to certain local stimuli after implantation of the device such as enzymes (e.g., matrix metalloproteinases) or pH changes, as well as external stimuli such as ultrasound as described in detail by Qu et al.210 While these types of coating are mainly achieved by using plasma spraying, electrochemical deposition, the sol–gel technique, and high-velocity suspension flame-spraying, their detailed application processes are described elsewhere.211
Hydroxyapatite coating on PEEK can be applied through various methods such as plasma spray, cold spray, and aerosol deposition. In vitro studies indicate that such application is associated with improved cell viability, adhesion, proliferation, and alkaline phosphatase activity.212–214 Although no published human clinical trials specifically address HA-coated PEEK cages, a recent multicentre, single-arm, prospective study assessed the safety and osteoconductivity of a silver-HA-coated (Ag-HA coating thickness: 2 mm, KYOCERA, Kyoto, Japan) Ti intervertebral cage (ResitageTM, Kyocera, Kyoto) used in PLIF or TLIF. The study, comprising 55 participants with six- and 12-month follow-up points for fusion rates (evaluated through lateral dynamic X-rays and multidetector-row CT scans), demonstrated comparable clinical and radiological outcomes. Nevertheless, further investigations, including a subsequent ongoing study (UMIN 000039964), are warranted to evaluate the antimicrobial and osteoconductive effectiveness of Ag-HA coatings against a control group, employing larger sample sizes to comprehensively assess the safety of Ag. Despite the absence of specific human trials, small animal studies with HA coating alone suggest increased peri-implant bone formation and biomechanically relevant bone implant-contact.212,215,216 Moreover, large animal studies have reported enhanced osteointegration and pull-out strength for plasma-sprayed HA coating on PEEK and carbon fibre-reinforced PEEK for implants placed in the pelvis.217 Although HA coatings naturally degrade in the body, contributing to their bioactivity, excessive degradation over a long-term implantation period could lead to adverse effects.218 This raises unresolved concerns regarding the efficacy and safety of HA coatings. Further research is essential to better understand and address the nuances of HA degradation, ensuring its optimal functionality throughout extended periods of implantation.
Recently, simple and cost-effective methods were developed such as a novel bioactive sol–gel TiO2 coating involving sandblasting and acid treatment offering increased PEEK bone-bonding ability without affecting mechanical behaviour.219 Thereby, the mechanical behaviour, i.e. the elastic modulus, is not altered because the surface coating does not exceed the glass transition temperature of PEEK.125,220 The bonding strength of the TiO2 gel layer to the PEEK was successfully evaluated with a modified ISO 2409 tape test.220 These results validated Pätsi et al.221 who suggested that sol–gel-derived layers were sufficient for their use as an implant coating material because of the bonding strength (>24 MPa). Sandblasting of the TiO2 particles and the sol–gel layer results in a strong chemical bonding layer between the two as well as a beneficial nano-scale roughness.222 Similar nano-scale roughness on neat PEEK was produced by Khoury et al.223 utilising a neutral atom beam technique to improve bone apposition in a rat calvarial model. Sol–gel-derived TiO2-coated bioactive PEEK implants demonstrated better fusion rates and bone-bonding ability compared to the uncoated PEEK implant in a canine anterior cervical fusion model.222 Further, the maximum temperature of 80 °C did not adversely affect the mechanical properties of PEEK.222In vitro and preclinical in vivo studies of cell activity and osteointegration have demonstrated improved cell attachment, greater bone volume and increased bone deposition and remodelling, fabricated by powder mixing and compression moulding methods,224 when comparing n-TiO2 nanoparticle/PEEK composite, against PEEK polymer control.225
Therefore, future implant structures and design may want to improve material chemistry and architecture as well as further optimise surfaces allowing for more efficient bone integration. For instance, PEEK composites, e.g. incorporating additives such as HA, or surface modifications may further improve the attractiveness of the biocompatible, inert, and inherently strong PEEK.
3.3.3 Alternative non-resorbable materials.
3.3.3.1 Tantalum (trabecular metal).
Comparable to Ti, Ta is a metal which has been used in orthopaedic surgery since the 1940s226 and is particularly noted for its porous structure, which closely resembles the cancellous bone. With a porosity ranging between 75% and 85%, porous Ta supports cell proliferation and osteogenesis of human osteoblasts.227 Its low elastic modulus closely mimics that of subchondral bone, while its high coefficient of friction provides excellent primary stability.228 For in depth overview of porous Ta in spinal surgery please consider the review of Hanc et al.228 Despite its favourable mechanical properties, Ta's biological inertness limits its ability to bond with bone. However, the open-pore structure may still promote vascularisation and bone remodelling. Studies have shown mixed results regarding its clinical efficacy.229
Bone marrow (BM)-derived mesenchymal stem cells (BM-MSCs) cultured on porous Ta implant and implanted in the intervertebral space of rabbits did not show superior lumbar interbody fusion rates compared to ABG alone after 12 months.230 Also, large preclinical animal studies indicate limited bone in growth of 8% to 15% into porous Ta implants at 12 weeks after implantation.231,232 A small clinical trial report of 40 patients that received anterior, posterior, or transforaminal lumbar 360° interbody fusion including a Ta cage without additional ABG showed a fair fusion rate of 68%.233 However, higher rates of subsidence234 and excessive artefacts on postoperative MRI and computed tomographic imaging are major concerns limiting the application of Ta implants.235
3.3.3.2 Silicon nitride (Si3N4).
Silicon nitride (Si3N4 implants, ceramic material) has recently attracted attention for its use in interbody fusion. Si3N4 is a hydrophilic, negatively charged ceramic with superior compressive strength compared to PEEK and Ti.236 Its surface charges enhance the adhesion of cells and proteins, which may facilitate better osteointegration.237 Moreover, Si3N4 implants tend to produce fewer artifacts on advanced imaging compared to other materials238 and exhibit increased resistance to bacterial biofilm formation.239 A two-year industry-sponsored randomized controlled trial comparing PEEK and Si3N4 in the TLIF technique found insufficient evidence to establish Si3N4's non-inferiority to PEEK. Nevertheless, both materials showed favourable clinical improvements.240 Similarly, a single-blinded randomised controlled trial comparing PEEK to Si3N4 in anterior cervical discectomy and fusion (ACDF) reported comparable clinical outcomes and fusing rates at 24 months for both materials.241 Additionally, a multicentre retrospective study found Si3N4 to have comparable pain reduction and reoperation rates to other materials used in various lumbar spinal fusion procedure.242 However, further prospective long-term studies are necessary to evaluate Si3N4's effectiveness in facilitating lumbar spinal fusion compared to conventional materials.237,239 Future research should also confirm the antimicrobial properties attributed to Si3N4. Current evidence suggests that Si3N4 does not significantly differ from conventional PEEK cages in terms of bony fusion outcomes.
3.3.3.3 Nitinol.
Nitinol, discovered in 1962 by William J. Buehler and later industrially utilised,243 has garnered interest for its unique properties. In the 1980s, its rheological similarity to biological tissues was noted following the development of specialised NiTi-based alloys.244 Details of material properties and characteristics are reviewed elsewhere.245 In brief, NiTi belongs to the family of Ti based intermetallic materials containing almost equal amount of Ni and Ti. Thermoelastic martensitic transformation properties allow for shape memory and superelastic characteristics.246,247 Its transformation temperature (36.85 °C) which is close to body temperature as well as low elastic modulus similar to bone and compressive strength greater than human bone make its use for biomedical implant applications appealing.248,249 Noteworthy, Ni is a toxic element with (contact) allergy reported for up to 20% of the female European population250 due to the corrosion of NiTi in physiological environments Ni and Ti are released.251 To improve corrosion resistance self-propagating high-temperature synthesis (SHS) is used to obtain porous NiTi alloys (PTN). Surface layers of PTN serve as a protective barrier in physiological environments, improving corrosion resistance.252 For instance, porous NiTi particles from the Actipore PLFx (Biorthex Inc., Montreal, QC, Canada), produced using SHS, were implanted in the spinal canal on the dura mater of rabbits.104 Follow-up studies showed no particles or abnormalities in the organs, mild inflammation confined to the epidural space, and similar results to a control group of Ti implants, suggesting that NiTi fabricated with SHS may be a safe option for intervertebral fusion devices.104 Ungrafted cylindric NiTi cages (Actipore™; Biorthex Inc., Montreal, QC, Canada) demonstrated favourable outcomes compared to conventional TiAlV cages (BAK™ cages; Sulzer Spine-Tech, Inc., Minneapolis, MN) packed with autologous bone, showing superior bone integration and apposition capacities in an ovine PLIF model.253 A retrospective cohort study of 41 patients receiving the porous NiTi cage (Actipore™; Biorthex Inc., Montreal, QC, Canada) reported a 98% complete fusion rate on radiography at one year.254 Further investigations on corrosion fatigue behaviours of porous NiTi alloys and prospective studies comparing to conventional interbody devices are necessary to accomplish a complete and systematic understanding of PTN.
4. Bone grafts to facilitate spinal fusion
4.1 Autograft
Autologous bone graft, harvested either from the iliac crest or local bone excised during spinal decompression, remains as the established gold standard for promoting bone regeneration in lumbar spinal fusion surgery.38,255,256 Despite the substantiated efficacy of iliac crest ABG demonstrated in numerous studies, concerns regarding associated morbidities, such as donor site pain, wound complications, prolonged operation time, and long-term functional impairment, have been reported.257–261 Importantly, volume loss of up to ±35% during the first year after implantation in the posterolateral spine is described due to the resorption and remodelling of the autograft.262–264 However, a preclinical large animal study employing a goat instrumental posterolateral fusion model observed superior fusion potential for autograft compared to donor allograft and synthetic bone substitutes.265 The recognised advantages of ABG, including its lower cost and absence of disease transmission risk, further underscore its significance.30 Recent studies have shifted focus towards local ABG retrieved from the vertebral segment, as extensively reviewed by others.266 Specifically, bone dust collected during spinal decompression has shown the capability to release growth factors and cytokines with anabolic effects on human osteoblasts.37 Moreover, studies have demonstrated a superior osteogenic potential of vertebral body BM-MSC compared to iliac crest BM-MSC.267 Several commercially available bone graft collectors,268 such as the Bone Vac (Stryker®) (Supplement 1) or Marrow Cellution™ (Medtronic®), are currently marketed with the aim of reusing or collecting local bone (dust) or BM to induce spinal fusion. In a goat intertransverse processes fusion model, intraoperatively isolated BM-MSC with poly-L-lysine-enriched demineralised bone matrix (DBM) showed comparable bone fusion to ABG.
However, the ideal biomaterial for cell delivery remains unidentified.269 For instance, delivery within a poly(lactic-co-glycolic acid) (PLGA)/biphasic calcium phosphate (BCP)/collagen graft, as opposed to a PLGA/HA/collagen composite,270 or BM-MSC within mineralised silk scaffold, as compared to a non-mineralised scaffold, induced greater bone formation.271 Importantly, human clinical studies focusing on fusion/pseudarthrosis rates and clinical outcomes while comparing different ABG sources for spinal interbody and posterolateral fusion are scarce.272 Therefore, the improvement of bone graft harvesting approaches, and the development of bone graft substitutes emerge as fundamental components of future research endeavours to enhance the success of spinal fusion surgeries. Table 1 provides a summary of the advantages and disadvantages, along with osteoconductive, osteogenic and osteoinductive properties of ABG and bone graft substitutes.
Table 1 Advantages and disadvantages along with osteoconductive, osteogenic and osteoinductive properties of autologous bone graft and bone graft substitutes. Partially created with BioRender.com. Table adapted from ref. 288 with permission from Elsevier, copyright (2021). Table adapted from ref. 289 with permission from Taylor & Francis, copyright (2012)
|
Autologous bone graft |
Allogenic bone graft |
Synthetic bone material |
Growth factors and bio-active molecules |
Form |
Cancellous (trabecular) and cortical |
Cancellous (trabecular), cortical and demineralised bone matrix (DBM) |
Pellets, powders, mixable injectable forms, injectable pastes, mouldable semi-solid cement, granules, and various implantable solid forms: blocks, cubes, wedges, cylinders |
Molecular carriers to deliver and hold to their intended targets in bone. Structural (e.g., synthetic polymers or calcium ceramics) or non-structural, e.g., BMP-saturated collagen sponges |
Advantages |
Gold standard as it is osteoconductive, osteoinductive and osteogenic290,291 |
No second surgical site (lower risk of infection and no additional pain)292 |
Many options for bone substitute materials, custom scaffolds (3D-printed and injectable)293,294 |
Both osteoinductive and osteoconductive properties289 |
Disadvantages |
Additional surgical site increases the risk of infection and limits the amount of available graft material289 |
Risk of disease transmission or adverse immune response274,295 |
Deficiency of growth factors to promote bone growth296 |
No long-term studies, inflammatory complications with off-label applications, very expensive28,297 |
Osteoconductive |
✓ |
✓ |
✓ |
✘ |
Osteoinductive |
✓ |
✓ |
✘ |
✓ |
Osteogenic |
✓ |
✘ |
✘ |
✘ |
Specification |
— |
Allogenic Spongiosa |
Demineralised bone matrix |
Tricalcium-phosphate |
Biphasic calcium-phosphate |
Calcium-phosphate |
Calcium-sulphate |
rhBMPs |
Costs in US-Dollar ($) |
Estimates report average (surgical) costs of 338–1000 $ depending on the size of the areas to be transplanted288 |
Cancellous, freeze-dried bone:298 376 $ per 30 cm3 |
Grafton/Allomatrix:298 726–1225 $ per 10 mL |
Vitoss, Orthovita: 875 $ per 10 mL |
NovaBone (Osteo-genics): 410 $ per cm3 |
CopiOs (Zimmer Spine): 1520 $ per 10 mL |
Osteoset (Wright Medical Technology): 655 $ per 10 mL |
OP-1 (Stryker) USD 5000; Infuse (Medtronic-Sofamor Danek) USD 3500–4900; 3500–5000 $ |
MinerOss cancellous bone (BioHorizons): 464 $ per cm3 |
DBX (Synthes)/Dynagraft: 200–4500 $ |
Chron OS (synthes): 475 $ per 5 cm3 |
Osteocel (NuVasive): 472 $ per cm3 |
4.2 Bone graft substitutes
The escalating incidence of spinal surgeries, coupled with the growing need for larger bone graft volumes, has driven the extension of autograft applications to promote effective bony healing.273 This surge in demand has spurred the development of diverse bone graft substitutes, including allografts and synthetic alternatives. Albeit a multitude of choices, allografts in comparison to autografts, exhibit diminished osteoinductive capacity, lack osteoprogenitor cells, pose risks of immune reactions, and carry a small but potential risk of disease transmission.274–276 Consequently, the reliance on synthetic bone graft substitutes has become prominent, with the United Kingdom alone offering 59 different products in the market.277 These substitutes are utilised in over a third of bone graft surgeries,278 underscoring their crucial role in mitigating complications associated with ABG and facilitating bony fusion, especially in complex, multi-segmental spine surgeries. Notably, synthetic bone graft substitutes comprise demineralised bone matrix (allograft) and various ceramic and bioglass formulations, alongside commercially available growth factor products. These alternatives contribute to the armamentarium of available options for enhancing spinal fusion.
4.2.1 Allografts.
Demineralised bone matrix is a cortical allograft bone that undergoes acid-decalcification and sterilisation, emerging as a supplementary or alternative option to ABG since its introduction in 1991. The mineralised component of bone is selectively removed through a mild acid-extraction process pioneered by Urist in 1965,279 resulting in a composite product of collagen, non-collagenous proteins and growth factors.280,281 Although its mechanical strength is significantly reduced during demineralisation and sterilisation, the trabecular structure is retained, along with some growth factors, preserving its osteoconductive and partially osteoinductive capacity.282 Due to its biological and structural properties, DBM is considered a hybrid material. In various commercially available products, DBM is used in combination with autograft, allograft, bone marrow aspirate, collagen–ceramic composites, polymer–ceramic composites, or growth factors.283 Fusion rates of 94%, comparable to those of ABG alone, have been observed in lumbar fusion using DBM as graft expander.284 A comprehensive review of the efficacy of DMB in spinal fusion has been published by others.281,285
In a more challenging elderly population undergoing posterolateral interbody fusion, the combination of DBM with BM aspirate yielded successful fusion in 84% of cases.286 However, using allograft alone as bone substitute in posterolateral fusion is not sufficient, with average fusion rates as low as 52% reported.273 The main underlying reason for slower and less complete allograft incorporation into native bone is particularly related to lack of vascularisation,274,287 emphasising the substantial need for further investment in synthetic bone grafts.
4.2.2 Synthetic bone grafts.
Traditionally, synthetic bone grafts investigated for spine surgery are CaP-based synthetic ceramics and bioactive glass.277,299 The mineralised inorganic phase of bone encompassing osteoconductive, biocompatible, and bone-bonding properties, bears similarity to the microstructure of ceramic composites.300 These inert substances pose no risk of disease transmission and have a long storage life.301 Consequently, the biocompatibility, osteoconductivity, and strong mechanical properties of ceramic-based substitutes are crucial features stemming from their chemical resemblance to the inorganic phase of bone, elucidating their relevant role in bone graft replacement.28,287 However, their limitations regarding osteogenic and osteoinductive capabilities, slow degradation, and mechanically brittle and stiff behaviour are less advantageous.282,302 Typically, mono-, bi- and tri-calcium phosphate and HA are employed alone or combined with collagen, polymers, or other carrier materials to create cement.303,304
BoneSave (Stryker), consisting of 80% Tri-Calcium Phosphate (TCP) and 20% HA provides an effective, biocompatible matrix. Clinical outcomes from the early 2000s showed a 57% fusion rate in a retrospective cohort of 45 patients undergoing PLIF, comparable to traditional allograft and autograft techniques.305,306 Recent third-generation bioactive ceramics have further explored the concept of standalone bone graft substitutes. Biphasic calcium phosphate (BCP) bone graft (MagnetOs; Kuros Biosciences), composed of 65–75% TCP (Ca3(PO4)2) and 25–35% HA (Ca10(PO4)6·(OH)2), exhibited similar performance in posterolateral spinal fusion in rabbits when used as autograft extender compared to autograft alone.307 AttraX (NuVasive) has also demonstrated success in animal models and clinical trials. In a recent clinical trial, 100 patients undergoing PLIF received AttraX putty and autografts on contralateral sides. At the one-year follow up, the bioactive ceramic demonstrated a 55% fusion rate compared to a 52% fusion rate for the autograft.308,309 The fusion rate at the two-year follow up significantly increased to 70% and 68% respectively, with no difference between the grafts.310
Additionally,311 observed equivalent performance in achieving spinal fusion in a large animal study of calcium phosphate with submicron topography compared to autograft. While fusion rates of up to 87% are reported for calcium phosphate compared to iliac crest bone graft (ICBG) rates of up to 90% after 33 months, it is concurrently associated with inflammation and wound healing complications in up to 51% of patients.312,313 Fusion rates of up to 100% after posterolateral fusion are observed for beta-TCP (β-TCP) in combination with autograft or allograft at three years follow-up.314 Hyperelastic Bone® is a 3D-printable HA-based material that, in combination with rhBMP-2, showed promising fusion rates, although when used alone, a sufficient fusion rate to justify clinical testing was not achieved.315 However, it is necessary to consider that bone and ceramic substitutes have similar density on plain radiographs and CT scans, potentially mimicking true bone fusion and thereby impairing the interpretation of fusion rates.
Bioactive glass, including 45S5 bioactive glass (i.e., Bioglass) developed by Hench and colleagues in the 1970s316 as a bone graft substitute material,299 stimulates activity of osteogenic cells in vitro by releasing ionic dissolution products (osteostimulation).317–319 In simulated body fluid, bioactive glass elicits deposition of a crystalline calcium phosphate surface layer,320,321 which is associated with osteoconduction and strong bone-bonding in vivo.148,322 Advanced laboratory techniques, including additive manufacturing, allowed for processing into 3D scaffolds, making it a promising synthetic bone graft substitute candidate. The increased porosity improved the osteoinduction and resorption while relevantly decreasing its mechanical strength, as shown in preclinical trials.323 However, in a preclinical large animal study of posterolateral fusion, no promotion of spinal fusion has been observed for 45S5 Bioglass in BG and TCP/BG grafts.324 Ultimately, inferior fusion results relative to autograft controls are observed because bioactive glass resorbs too rapidly before bone formation can occur.325 Thus, osteostimulative bioglass in its current texture and biological composition may have limited relevance as bone graft material in spinal fusion. Moreover, preclinical animal studies indicate an inflammatory foreign body reaction around bioglass.326–328 Nonetheless, the authors are confident that ongoing developments in additive manufacturing will support and improve the application possibilities of bioglass while stressing its inherently suitable osteogenic properties as a bone substitute.
4.2.3 Growth factors and bio-active molecules.
The differentiation, maturation, and proliferation of MSCs into osteogenic cells are substantially influenced by growth factors (Fig. 7). Potential growth factor candidates include bone morphogenetic proteins (BMPs), transforming growth factor-β, and platelet-derived growth factor. In this review, we focus on BMPs, initially described by Marshal Urist in 1965,279 later identified as soluble members of the transforming growth factor-β superfamily.261,329 The stimulation of bone healing by BMPs,330 particularly rhBMP-2 (INFUSE, Medtronic) and rhBMP-7 (OP-1 putty, Stryker Biotech) in the early 2000s, has been demonstrated as safe and efficacious in non-union of long bones.331 Strong interactions with bone-like mineral substrates have also been shown for multiple growth factors, including BMP-2, insulin-like growth factor-1 (IGF-1) and TGF-β.332–337 In non-unions, rhBMP-7 has been shown to have an efficacy similar to bone grafting, and fewer complications.338 However, large, prospective, randomised, controlled multicentre clinical trials investigating OP-1 for its use in lumbar spinal fusion showed superior results for autograft versus OP-1 on plain films at 2-year follow-up and CT-scan results at 3 years,339,340 leading to FDA rejection of Pre-Market Approval (PMA) of OP-1 in April 2009.341 This biological enhancer of bone formation is currently not commercially available.342 Additionally, rhBMP-7 is associated with high costs and unknown carcinogenic potential.343 Thus, rhBMP-2 is the most clinically relevant growth factor. Extensive reviews of additionally relevant growth factors and signalling molecules for migration and differentiation of bone formatting cells are published by others.344,345
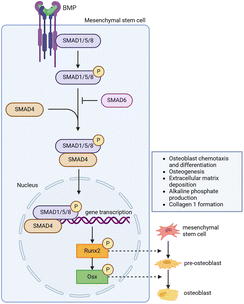 |
| Fig. 7 Working mechanism of bone morphogenetic proteins in bone regeneration. Figure created with BioRender.com. | |
RhBMP-2 achieved FDA approval for single-level ALIF with a specific Ti cage in 2002. Any other application is considered off-label or ‘physician-directed application’. Its use in spinal fusion surgery increased from 0.7% in 2002 to 20% in 2006 and Medtronic reports nearly $400 million dollars in sales in 2012.346 However, due to serious complications including inflammation and pain associated with supraphysiologic dosing and off-label use, the clinical use of rhBMP-2 has drastically decreased.297,347 Nonetheless, rhBMP-2 is an FDA-approved bone graft substitute for spine fusion, used in combination with a type I collagen sponge as a carrier and commercially available as an additional substrate with high fusion rates without autograft bone or bone graft extenders.346,348,349 The landmark study of rhBMP-2 published by Burkus et al.350 demonstrated that patients treated with rhBMP-2 with a Lumbar Tapered Fusion Device (LT-CAGE, Medtronic Sofamor Danek, Memphis, TN, USA) had superior fusion rates and functional outcome compared to patients treated with ICBG. Particularly, they observed in a follow-up study including the combination of datasets from two additional clinical trials superior 24-months fusion rates for rhBMP-2 (94.4%) compared to autograft (89.4%).351 These beneficial radiographic and clinical outcomes have been challenged in multiple reports and trials reporting significant endplate resorption, osteolysis, and graft subsidence with rhBMP-2 used in ALIF.352–354 Higher reoperation rates in patients treated with rhBMP-2 has also been attributed to graft subsidence complications.355 Further, increased rates of retrograde ejaculation of 6–7% are reported in ALIF with rhBMP-2356,357 compared to baseline rates of less than 1% in patients with no rhBMP-2 application.358–360
The use of rhBMP-2 in PLIF has been evaluated in two prospective randomised multicentre trials showed. In the first trial, a dose of 20 mg per side resulted in a higher fusion rate compared to iliac crest autograft alone (100% vs. 40%). In the second study, a higher dose of rhBMP-2 (AMPLIFY rhBMP-2 Matrix; Medtronic Sofamor Danek) was used, showing once again higher fusion rates relative to autograft (96% versus 89%) at the 2-year follow-up (p = 0.014). While clinical outcomes were similar between the two groups, the autograft group experienced significantly higher reoperation rates (16% vs. 8%, p = 0.015), and at two-year follow-up, 60% reported donor site iliac crest pain.361 However, a retrospective review involving 130 patients undergoing PLIF with rhBMP-2 reported a reoperation rate of 4.6% due to sterile seromas.362 Concerns regarding these complications led to the FDA rejecting Medtronic's application for the use of a higher-dose rhBMP-2 (AMPLIFY) in PLIF in March 2011. In summary, despite the relatively low absolute morbidity risk associated with the rhBMP-2 use in PLIF, which primarily involves higher rates of ectopic bone formation (EBF), a meta-analysis suggests that rhBMP-2 increases the likelihood of successful fusion without a clinically relevant reduction in pain for up to 24 months.363
The application of RhBMP-2 in PLIF as a fusion-inducing agent applied within intervertebral cages has demonstrated superior fusion rates compared to autograft. However, this approach is associated with relevant EBF, resulting in statistically significant extradiscal bone formation. Importantly, this increased EBF has not been correlated with clinical symptoms.364 Reports on the incidence of asymptomatic EBF are inconclusive, with rates ranging from very low to high.365–368 The off-label use of rhBMP-2 in TLIF, combined with allograft or autograft,369,370 has shown good fusion rates with improvements in clinical systems. However, it is noteworthy that there are no available prospective randomised studies on this application. Complications associated with rhBMP-2 use in TLIF procedures have been reported in various case series and reports.45,371,372 Notably, postoperative radiculitis has been described in up to 20% of cases,371 along with delayed neural compression due to symptomatic EBF,45 and vertebral osteolysis in the range of 5.8% to 7.4%.371,372 In summary, the use of rhBMP-2 may be considered in PLIF when autograft is unavailable or harvesting is rejected by the patient.341 However, caution is advised in PLIF and TLIF procedures due to concerns about EBF and potential neurological compromise.363
The delivery of pluripotent MSCs or growth factors represents a novel therapeutic approach aimed at stimulating bone deposition and amplifying fusion directly, potentially as a component of biodegradable scaffold material.373–375 In a preclinical large animal thoracic fusion study, superior histological and biomechanical fusion outcomes were observed when rhBMP-2 was loaded onto collagen-coated medical-grade polycaprolactone TCP (mPCL-TCP) scaffolds compared to ABG.376 Recognising the dose dependent response of rhBMP-2 on bone formation, it remains a potent agent in fostering bone formation while avoiding osteolytic outcomes.377 Additionally, rhBMP-2 may serve as template substrate to improve the binding of HA minerals, fostering bone healing and regeneration.332,337 More recently, an in vitro study suggests that achieving sufficient release rates of rhBMP-2 over a 2–4 weeks period is crucial for maximising angiogenesis and osteogenesis, essential components for successful bone regeneration.378
Incorporating growth factors into tailored bioresorbable implants with tuneable release is feasible379 and likely associated with accelerating tissue regeneration by matching the spatiotemporal demand.380 However, the design and manufacturing process of suitable delivery vehicles pose challenges, and dosing remains an issue.373 Currently, a commercially available delivery system for BMPs involves collagen sponges soaked in BMP at concentrations significantly higher than physiologically found in the human body. The effects of large bolus release are still under debate, and sponge-based BMP delivery systems, reliant on absorption into the sponge, make controlled delivery difficult to achieve. Initial reports suggest osteolysis of surrounding bone, promoting implant subsidence, swelling of surrounding soft tissue, and EBF. Future research explore alternative physiological and biocompatible carriers for BMPs, such as OSTEOGROW, derived from blood coagulum, aiming to address current challenges and improve outcomes in bone regeneration.381
5. Conclusions and outlook
The growing prevalence of interbody spinal fusion, particularly among patients aged 75 and above, presents a looming challenge for global healthcare systems.3 Despite notable advancements in surgical techniques, biomaterials and spinal implants, the incidence of pseudarthrosis in over 10% of patients382 presents significant hurdles, manifesting in poorer patient-reported outcomes, heightened demand for revision procedures, and elevated healthcare expenditures.383–385
The landscape of interbody devices has undergone transformations, with modifications in Ti and PEEK devices incorporating diverse surface coatings and modifications. However, their efficacy lacks comprehensive validation from preclinical and clinical studies. A notable development has been the advent of 3D-printed Polyetherketoneketone (PEKK), exemplified by the TETRAfuse 3D spinal interbody implants. Preliminary findings from preclinical trials indicate enhanced implant osteointegration and trabecular bone in-growth, promising a potential paradigm shift in spinal fusion technology.386 A PEKK nano-roughened surface with antibacterial properties, has shown potential in fusion within an ovine bone femoral defect model, addressing concerns related to surface delamination and inadequate bone apposition linked to traditional materials like PEEK and Ti-coated PEEK.387 The future trajectory of this innovative cage candidate hinges on insightful clinical studies.
Traditional reliance on the gold standard of ABGs has been tempered by associated morbidity and limited donor tissue availability, fostering the quest for alternatives. Encouragingly, multiple rabbit studies exploring combinations of porous HA/polylactic acid (PLA) composites,388 silicate-substituted HA grafts,389 and HA/TCP grafts390 have exhibited radiographic outcomes comparable to or surpassing those achieved with ABG. These studies, validated for true inter-transverse process arthrodesis, hold promise in translational relevance to humans.391 Synthetic ceramic scaffolds, acting as extenders or replacements for bone grafts, have shown efficacy in providing a highly porous 3D-structure conducive to improved cell migration and osteointegration. Novel modifications, such as coating DBM with poly-L-lysine, or DBM supplemented with TCP, as shown in studies with protein kinase C-binding protein (NEL)-like protein-1 (NELL-1),392,393 highlight the ongoing exploration of these materials as potential graft extenders, with future investigations poised to unveil their clinical efficacy.394–396
The intersection of material science and engineering has given rise to novel biologic materials, including nanocomposites, 3D-printed materials, and various biologic composites.397 These innovations address inherent limitations in current bone graft substitutes.398 Notably, DBM and cancellous scaffolds can serve as carriers for allogeneic MSCs and bone-marrow derived osteoprogenitor cells, exemplified by cellular bone matrices (e.g., Osteocel™, Nuvasive, Ca, USA). Preliminary outcomes, including fusion rates of up to 91.3%–92.3% in lumbar interbody fusion procedures with Osteocel™ (Plus)™, demonstrate promise with low complication rates of less than 2%.399,400 The availability of various cellular bone matrices in the market necessitates future prospective studies to compare them to BMPs and ABGs for more conclusive recommendations.401–403 Furthermore, integrating bioactive peptides with porous implants and materials has advanced the development of fusion extenders.404 Notably, P-15™, a synthetic polypeptide that facilitates osteogenic cell attachment by mimicking a domain of type I collagen405,406 has recently transitioned from dental applications to spinal use. I-FACTOR™ (Cerapedics, Inc., Westminster, CO), a proprietary composite of P-15 and anorganic bovine bone mineral (ABM),407 has shown promising outcomes in enhancing fusion and clinical results in patients undergoing ALIF for degenerative spine conditions.404 In PLIF procedures, I-FACTOR demonstrated efficacy similar to or better than autografts at both 6 and 12 months, with improvements in pain and function surpassing success criteria at all evaluated intervals.405 While conclusive findings for lumbar spine applications pose challenges, recent level III and IV studies suggest that ABM/P-15 may offer benefits for lumbar fusion.407
The culmination of successful 360° stabilisation and fusion hinges on the harmonious integration of spinal instrumentation, intervertebral body devices, and ABGs or bone graft substitutes. The advent of additive manufacturing has democratised medical device research and development, equipping laboratories with the tools to explore complex interbody cage designs that tailor geometry, porosity, and interconnectivity to achieve better biomimetic mechanical performance, osteogenic differentiation, and osteointegration in in vivo models. Surface topographic modifications, particularly, HA coatings, antibacterial elements, and mechanical roughening show great promise and contribute to the development of an inhabitable environment, fostering optimal conditions for cellular processes critical to successful fusion. As research endeavours unfold, the synergistic relationship between technology and biological materials holds the key to unlock transformative advancements in the field of lumbar spinal fusion. However, the trajectory towards safer and more efficacious procedures is contingent on collaborative efforts and innovative research, placing substantial emphasis on rigorous clinical validations. While in vitro and animal in vivo trials provide valuable insights, the translation of these findings into clinical practice necessitates robust clinical evidence. A concerted commitment to comprehensive clinical validations will be pivotal in ensuring that the promising developments witnessed in the laboratory setting translate into tangible benefits for patients undergoing spinal fusion procedures.
Author contributions
Giles M. Cheers: conceptualization, methodology, investigation, writing – original draft, visualization. Lucas P. Weimer: methodology, investigation, writing – review & editing, visualization. Carl Neuerburg: methodology, investigation, writing – review & editing. Jörg Arnholdt: methodology, investigation, writing – review & editing. Fabian Gilbert: methodology, investigation, writing – review & editing. Christoph Thorwächter: methodology, investigation, writing – review & editing. Boris M. Holzapfel: methodology, investigation, resources, writing – review & editing, supervision. Susanne Mayer-Wagner: methodology, investigation, resources, writing – review & editing, supervision. Markus Laubach: conceptualization, methodology, writing – original draft, visualization, supervision, project administration.
Data availability
No primary research results, software or code have been included and no new data were generated or analysed as part of this review.
Conflicts of interest
The authors declare that they have no known competing financial interests or personal relationships that could have appeared to influence the work reported in this paper.
Acknowledgements
Graphical abstract created with https://www.BioRender.com, scaffold created with nTop, Release 4.21.1, nTop Inc., https://www.ntop.com. We would like to thank Sara Mohr for her support in designing Fig. 1 and 7, which were created using https://www.BioRender.com. The authors gratefully acknowledge the support of the Alexander von Humboldt Foundation funding a Feodor Lynen Return Fellowship awarded to Markus Laubach.
References
- G. B. Andersson, Lancet, 1999, 354, 581–585 CrossRef CAS.
- B. I. Martin, S. K. Mirza, N. Spina, W. R. Spiker, B. Lawrence and D. S. Brodke, Spine, 2019, 55, 369–376 CrossRef.
- V. J. Heck, K. Klug, T. Prasse, S. Oikonomidis, A. Klug, B. Himpe, P. Egenolf, M. Lenz, P. Eysel and M. J. Scheyerer, Clin. Orthop. Relat. Res., 2023, 481, 1610–1619 CrossRef.
- T. Mabud, J. Norden, A. Veeravagu, C. Swinney, T. Cole, B. A. McCutcheon and J. Ratliff, Clin. Spine Surg., 2017, 30, E1376–e1381 CrossRef PubMed.
- R. A. Deyo, B. I. Martin, W. Kreuter, J. G. Jarvik, H. Angier and S. K. Mirza, J. Bone Joint Surg. Am., 2011, 93, 1979–1986 CrossRef PubMed.
- B. I. Martin, S. K. Mirza, B. A. Comstock, D. T. Gray, W. Kreuter and R. A. Deyo, Spine, 2007, 32, 382–387 CrossRef.
- A. D. Malter, B. McNeney, J. D. Loeser and R. A. Deyo, Spine, 1998, 23, 814–820 CrossRef CAS.
- M. Laubach, P. Kobbe and D. W. Hutmacher, Biomaterials, 2022, 288, 121699 CrossRef CAS PubMed.
- K. Kumar, Spine, 1996, 21, 653–655 CrossRef CAS.
- J. T. Hughes, Paraplegia, 1988, 26, 71–82 CAS.
- S. Naderi, N. Andalkar and E. C. Benzel, Neurosurgery, 2007, 60, 382–391 CrossRef PubMed.
- J. Lister, Br. Med. J., 1867, 2, 246–248 CrossRef CAS PubMed.
- D. H. Robinson and A. H. Toledo, J. Invest. Surg., 2012, 25, 141–149 CrossRef PubMed.
- E. A. Underwood, Proc. R. Soc. Med., 1945, 38, 697–706 CAS.
- J. Dobson, Ann. R. Coll. Surg. Engl., 1972, 50, 54–65 CAS.
- T. M. Daniel, Respir. Med., 2006, 100, 1862–1870 CrossRef.
- S. L. de Kunder, K. Rijkers, I. J. M. H. Caelers, R. A. de Bie, P. J. Koehler and H. van Santbrink, Spine, 2018, 43, 1161–1168 CrossRef.
- R. A. Deyo, A. Nachemson and S. K. Mirza, N. Engl. J. Med., 2004, 350, 722–726 CrossRef CAS PubMed.
- R. N. Stauffer and M. B. Coventry, J. Bone Jt. Surg., 1972, 54, 756–768 CrossRef CAS.
- R. B. Cloward, J. Neurosurg., 1953, 10, 154 CAS.
- J. S. Silber, D. G. Anderson, S. D. Daffner, B. T. Brislin, J. M. Leland, A. S. Hilibrand, A. R. Vaccaro and T. J. Albert, Spine, 2003, 28, 134–139 CrossRef PubMed.
- R. L. Macdonald, M. G. Fehlings, C. H. Tator, A. Lozano, J. R. Fleming, F. Gentili, M. Bernstein, M. C. Wallace and R. R. Tasker, J. Neurosurg., 1997, 86, 990 CAS.
- A. Rieger, C. Holz, T. Marx, L. Sanchin and M. Menzel, Neurosurgery, 2003, 52, 449–453 CrossRef ; discussion 453–444.
- K. U. Lewandrowski, A. C. Hecht, T. F. DeLaney, P. A. Chapman, F. J. Hornicek and F. X. Pedlow, Spine, 2004, 29, 1150–1158 CrossRef ; discussion 1159.
- K. H. Bridwell, L. G. Lenke, K. W. McEnery, C. Baldus and K. Blanke, Spine, 1995, 20, 1410–1418 CrossRef CAS.
- D. E. Couture and C. L. Branch Jr., Neurosurg. Focus, 2004, 16, E8 Search PubMed.
- C. R. Fischer, R. Cassilly, W. Cantor, E. Edusei, Q. Hammouri and T. Errico, Eur. Spine J., 2013, 22, 1423–1435 CrossRef.
- V. Campana, G. Milano, E. Pagano, M. Barba, C. Cicione, G. Salonna, W. Lattanzi and G. Logroscino, J. Mater. Sci.: Mater. Med., 2014, 25, 2445–2461 CrossRef CAS PubMed.
- H. C. Pape, A. Evans and P. Kobbe, J. Orthop. Trauma, 2010, 24, S36–S40 CrossRef PubMed.
- G. Grabowski and C. A. Cornett, J. Am. Acad. Orthop. Surg., 2013, 21, 51–60 CrossRef.
- K. G. Heiple, V. M. Goldberg, A. E. Powell, G. D. Bos and J. M. Zika, Orthop. Clin. N. Am., 1987, 18, 179–185 CrossRef CAS.
- J. A. Goulet, L. E. Senunas, G. L. Desilva and M. L. Greenfield, Clin. Orthop. Relat. Res., 1997, 76–81 CrossRef CAS.
- D. H. Kim, R. Rhim, L. Li, J. Martha, B. H. Swaim, R. J. Banco, L. G. Jenis and S. G. Tromanhauser, Spine J., 2009, 9, 886–892 CrossRef.
- R. C. Sasso, J. I. Williams, N. Dimasi and P. R. Meyer, J. Bone Joint Surg. Am., 1998, 80, 631–635 CrossRef CAS.
- S. Khan, F. P. Cammisa, H. Sandhu, A. Diwan, F. Girardi and J. Lane, J. Am. Acad. Orthop. Surg., 2005, 13, 77–86 CrossRef PubMed.
- P. Ganguly, J. J. El-Jawhari, P. V. Giannoudis, A. N. Burska, F. Ponchel and E. A. Jones, Cell Transplant., 2017, 26, 1520–1529 Search PubMed.
- R. Gao, M. Street, M. L. Tay, K. E. Callon, D. Naot, A. Lock, J. T. Munro, J. Cornish, J. Ferguson and D. Musson, Spine, 2018, 43, E193–E199 CrossRef PubMed.
- F. Migliorini, F. Cuozzo, E. Torsiello, F. Spiezia, F. Oliva and N. Maffulli, J. Clin. Med., 2021, 10, 4347 CrossRef PubMed.
- F. Oliva, F. Migliorini, F. Cuozzo, E. Torsiello, F. Hildebrand and N. Maffulli, J. Orthop. Trauma., 2021, 22, 50 CrossRef PubMed.
- D. S. Chun, K. C. Baker and W. K. Hsu, Neurosurg. Focus, 2015, 39, E10 Search PubMed.
- M. B. Kornblum, J. S. Fischgrund, H. N. Herkowitz, D. A. Abraham, D. L. Berkower and J. S. Ditkoff, Spine, 2004, 29, 726–733 CrossRef ; discussion 733–724.
- G. W. Bagby, Orthopedics, 1988, 11, 931–934 CrossRef CAS PubMed.
- S. D. Kuslich, C. L. Ulstrom, S. L. Griffith, J. W. Ahern and J. D. Dowdle, Spine, 1998, 23, 1267–1278 CrossRef CAS ; discussion 1279.
- H. S. Sandhu, J. M. Toth, A. D. Diwan, H. B. Seim 3rd, L. E. Kanim, J. M. Kabo and A. S. Turner, Spine, 2002, 27, 567–575 CrossRef PubMed.
- N. F. Chen, Z. A. Smith, E. Stiner, S. Armin, H. Sheikh and L. T. Khoo, J. Neurosurg. Spine, 2010, 12, 40–46 Search PubMed.
- P. C. McAfee, J. Bone Joint Surg. Am., 1999, 81, 859–880 CrossRef CAS PubMed.
- M. Kanayama, B. W. Cunningham, C. J. Haggerty, K. Abumi, K. Kaneda and P. C. McAfee, J. Neurosurg., 2000, 93, 259–265 CAS.
- M. van Dijk, T. H. Smit, S. Sugihara, E. H. Burger and P. I. Wuisman, Spine, 2002, 27, 682–688 CrossRef.
- C. C. Niu, J. C. Liao, W. J. Chen and L. H. Chen, J. Spinal Disord. Tech., 2010, 23, 310–316 CrossRef.
- S. Seaman, P. Kerezoudis, M. Bydon, J. C. Torner and P. W. Hitchon, J. Clin. Neurosci., 2017, 44, 23–29 CrossRef CAS.
- D. V. Patel, J. S. Yoo, S. S. Karmarkar, E. H. Lamoutte and K. Singh, J. Spine Surg., 2019, S19–S24 CrossRef PubMed.
- W. K. Hsu, C. L. Goldstein, M. F. Shamji, S. K. Cho, P. M. Arnold, M. G. Fehlings and T. E. Mroz, Neurosurgery, 2017, 80, S100–S107 CrossRef.
- C. M. Han, E. J. Lee, H. E. Kim, Y. H. Koh, K. N. Kim, Y. Ha and S. U. Kuh, Biomaterials, 2010, 31, 3465–3470 CrossRef CAS.
- B. C. Cheng, S. Koduri, C. A. Wing, N. Woolery, D. J. Cook and R. C. Spiro, Med. Devices, 2018, 11, 391–402 CrossRef.
- H. Sakaura, A. Ohnishi, A. Yamagishi and T. Ohwada, Asian Spine J., 2019, 13, 248–253 CrossRef PubMed.
- H. Manabe, T. Sakai, M. Morimoto, F. Tezuka, K. Yamashita, Y. Takata and K. Sairyo, J. Med. Invest., 2019, 66, 119–122 CrossRef.
- M. Rickert, C. Fleege, T. Tarhan, S. Schreiner, M. R. Makowski, M. Rauschmann and M. Arabmotlagh, Bone Jt. J., 2017, 99-B, 1366–1372 CrossRef CAS.
- T. Hasegawa, H. Ushirozako, E. Shigeto, T. Ohba, H. Oba, K. Mukaiyama, S. Shimizu, Y. Yamato, K. Ide, Y. Shibata, T. Ojima, J. Takahashi, H. Haro and Y. Matsuyama, Spine, 2020, 45, E892–E902 CrossRef.
- R. Olivares-Navarrete, R. A. Gittens, J. M. Schneider, S. L. Hyzy, D. A. Haithcock, P. F. Ullrich, Z. Schwartz and B. D. Boyan, Spine J., 2012, 12, 265–272 CrossRef PubMed.
- R. Olivares-Navarrete, S. L. Hyzy, P. J. Slosar, J. M. Schneider, Z. Schwartz and B. D. Boyan, Spine, 2015, 40, 399–404 CrossRef PubMed.
- F. Kong, Z. Nie, Z. Liu, S. Hou and J. Ji, J. Photochem. Photobiol., B, 2018, 187, 120–125 CrossRef CAS PubMed.
- R. Ermawan, H. Corrigan and N. Wiyono, J. Orthop., 2024, 50, 22–28 CrossRef PubMed.
- X. Han, Y. Zhu, C. Cui and Y. Wu, Spine, 2009, 34, E618–E625 CrossRef PubMed.
- R. Soegaard, C. E. Bünger, T. Christiansen, K. Høy, S. P. Eiskjaer and F. B. Christensen, Spine, 2007, 32, 2405–2414 CrossRef PubMed.
- R. Roy-Camille, M. Roy-Camille and C. Demeulenaere, Presse Med., 1970, 78, 1447–1448 CAS.
- M. B. Kabins and J. N. Weinstein, Iowa Orthop. J., 1991, 127–136 Search PubMed.
- J. M. Cotler, J. V. Vernace and J. A. Michalski, Orthop. Clin. N. Am., 1986, 17, 87–103 CrossRef CAS PubMed.
- H. Wang, Y. Zhao, Z. Mo, J. Han, Y. Chen, H. Yu, Q. Wang, J. Liu, C. Li, Y. Zhou and L. Xiang, Clinics, 2017, 72, 609–617 CrossRef.
- W. Yao, T. Zhou, K. Huang, M. Dai, F. Mo, J. Xu, Z. Cao, Q. Lai, B. Xie, R. Guo and B. Zhang, Ann. Transl. Med., 2021, 9, 669 CrossRef.
- W. Qin, K. Chen, H. Chen, P. Yang, H. Yang and H. Mao, World Neurosurg., 2020, 138, e10–e16 CrossRef PubMed.
- J. Li, L.-C. Zhang, J. Li, H. Zhang, J.-X. Zhao and W. Zhang, BioMed Res. Int., 2020, 2020, 5497030 Search PubMed.
- E. P. de Kater, A. Sakes, E. Edström, A. Elmi-Terander, G. Kraan and P. Breedveld, Eur./ Spine J., 2022, 31, 1553–1565 CrossRef.
- M.-K. Hsieh, Y.-D. Li, Y.-J. Hsu, T.-T. Tsai, P.-L. Lai, D.-M. Lee and C.-L. Tai, Appl. Sci., 2022, 12, 6172 CrossRef CAS.
- C.-L. Tai, T.-T. Tsai, P.-L. Lai, Y.-L. Chen, M.-Y. Liu and L.-H. Chen, PLoS One, 2015, 10, e0146294 CrossRef.
- M. Law, A. F. Tencer and P. A. Anderson, Spine, 1993, 18, 2438–2443 CrossRef CAS.
- R. Skinner, J. Maybee, E. Transfeldt, R. Venter and W. Chalmers, Spine, 1990, 15, 195–201 CrossRef CAS.
- T. Hasegawa, A. Inufusa, Y. Imai, Y. Mikawa, T. H. Lim and H. S. An, Spine J., 2005, 5, 239–243 CrossRef PubMed.
- B. Sandén, C. Olerud, C. Johansson and S. Larsson, Spine, 2001, 26, 2673–2678 CrossRef PubMed.
- S. Yi, D. C. Rim, S. W. Park, J. A. Murovic, J. Lim and J. Park, World Neurosurg., 2015, 83, 976–981 CrossRef PubMed.
- L.-Y. Shi, A. Wang, F.-Z. Zang, J.-X. Wang, X.-W. Pan and H.-J. Chen, Colloids Surf., B, 2017, 160, 22–32 CrossRef CAS PubMed.
- G. M. Liu, N. Kong, X. Y. Zhang, H. T. Bai, Y. Yao, H. Z. Han and Y. G. Luo, Eur. J. Orthop. Surg. Traumatol., 2014, 24(Suppl 1), S173–S182 CrossRef.
- P. R. Harrington, J. Bone Jt. Surg., 1962, 44, 591–634 CrossRef.
- A. Warburton, S. J. Girdler, C. M. Mikhail, A. Ahn and S. K. Cho, Neurospine, 2020, 17, 101–110 CrossRef PubMed.
- B. Meng, J. Bunch, D. Burton and J. Wang, Eur. Spine J., 2021, 30, 22–33 CrossRef PubMed.
- J. Litak, M. Szymoniuk, W. Czyżewski, Z. Hoffman, J. Litak, L. Sakwa and P. Kamieniak, Materials, 2022, 15, 3650 CrossRef CAS PubMed.
- H. Yoshihara, Spine J., 2013, 13, 1350–1358 CrossRef PubMed.
- W. Li, H. Zhao, C. Li, T. Liu, J. Guan, Y. Yang and X. Yu, J. Orthop. Surg. Res, 2023, 18, 348 CrossRef PubMed.
- L. Qi, M. Li, S. Zhang, J. Xue and H. Si, Acta Neurochir., 2013, 155, 1187–1193 CrossRef PubMed.
- R. K. Ponnappan, H. Serhan, B. Zarda, R. Patel, T. Albert and A. R. Vaccaro, Spine J., 2009, 9, 263–267 CrossRef.
- C. Li, L. Liu, J. Y. Shi, K. Z. Yan, W. Z. Shen and Z. R. Yang, Neurosurg. Rev., 2018, 41, 375–389 CrossRef.
- D. Grob, A. Benini, A. Junge and A. F. Mannion, Spine, 2005, 30, 324–331 CrossRef.
- X. Zhao, M. Niinomi, M. Nakai and J. Hieda, Acta Biomater., 2012, 8, 1990–1997 CrossRef CAS.
- H. Liu, M. Niinomi, M. Nakai, K. Cho, K. Narita, M. Şen, H. Shiku and T. Matsue, Acta Biomater., 2015, 12, 352–361 CrossRef CAS.
-
F.-Y. Tsuang, Y.-Y. Hsieh, Y.-J. Kuo, C.-H. Chen, F.-H. Lin, C.-S. Chen and C.-J. Chiang, PLoS One, 2017, 12, e0188034 Search PubMed.
- C. Lindsey, V. Deviren, Z. Xu, R. F. Yeh and C. M. Puttlitz, Spine, 2006, 31, 1680–1687 CrossRef PubMed.
- J. C. Dick and C. A. Bourgeault, Spine, 2001, 26, 1668–1672 CrossRef CAS PubMed.
- K. Yamada, H. Sudo, N. Iwasaki and A. Chiba, Spine, 2020, 45, E312–E318 CrossRef.
- C. R. Faulks, D. T. Biddau, N. R. Munday, D. P. McKenzie and G. M. Malham, J. Spine Surg., 2023, 9, 409–421 CrossRef.
-
S. M. Kurtz and T. Lanman, in PEEK Biomaterials Handbook, ed. S. M. Kurtz, William Andrew Publishing, 2nd edn, 2019, pp. 281–289. DOI:10.1016/B978-0-12-812524-3.00016-8.
- I. Gotman, J. Endourol., 1997, 11, 383–389 CrossRef CAS.
- H. Serhan, D. Mhatre, P. Newton, P. Giorgio and P. Sturm, Clin. Spine Surg., 2013, 26, E70–E74 Search PubMed.
- A. Angelliaume, E. Ferrero, K. Mazda, M. Le Hanneur, F. Accabled, J. S. de Gauzy and B. Ilharreborde, Eur. Spine J., 2017, 26, 1732–1738 CrossRef PubMed.
- F. U. Ahmad, C. Sidani, R. Fourzali and M. Y. Wang, J. Neurosurg. Spine., 2013, 19, 629 Search PubMed.
- S. Rhalmi, S. Charette, M. Assad, C. Coillard and C. H. Rivard, Eur. Spine J., 2007, 16, 1063–1072 CrossRef.
- Y. S. Kim, H. Y. Zhang, B. J. Moon, K. W. Park, K. Y. Ji, W. C. Lee, K. S. Oh, G. U. Ryu and D. H. Kim, Neurosurg. Focus, 2007, 22, E10 Search PubMed.
- A. Biesiekierski, J. Wang, M. Abdel-Hady Gepreel and C. Wen, Acta Biomater., 2012, 8, 1661–1669 CrossRef CAS PubMed.
- A. Kirisits and W. K. Redekop, Appl. Health Econ. Health policy, 2013, 11, 15–26 CrossRef.
- A. J. Talia, M. L. Wong, H. C. Lau and A. H. Kaye, J. Clin. Neurosci., 2015, 22, 243–251 CrossRef.
- M.-J. Reisener, M. Pumberger, J. Shue, F. P. Girardi and A. P. Hughes, J. Spine Surg., 2020, 6, 752–761 CrossRef.
- R. J. Mobbs, K. Phan, G. Malham, K. Seex and P. J. Rao, J. Spine Surg., 2015, 1, 2–18 Search PubMed.
- M. A. Flierl, K. M. Beauchamp, G. E. Bolles, E. E. Moore and P. F. Stahel, Patient Saf. Surg., 2009, 3, 4 CrossRef PubMed.
- P. Korovessis, G. Petsinis, G. Koureas, P. Iliopoulos and S. Zacharatos, Spine, 2006, 31, 1014–1019 CrossRef PubMed.
- Y. Robinson, S. K. Tschoeke, T. Finke, R. Kayser, W. Ertel and C. E. Heyde, Acta Orthop., 2008, 79, 660–664 CrossRef.
- Y. Robinson, S. K. Tschoeke, R. Kayser, H. Boehm and C. E. Heyde, Int. Orthop., 2009, 33, 745–749 CrossRef PubMed.
- M. Ruf, D. Stoltze, H. R. Merk, M. Ames and J. Harms, Spine, 2007, 32, E275–E280 CrossRef.
- M. Long and H. Rack, Biomaterials, 1998, 19, 1621–1639 CrossRef CAS.
- T. Lanman and T. Hopkins, Neurosurg. Focus, 2004, 16(3), E6 Search PubMed.
- C. R. Lippman, M. Hajjar, B. Abshire, G. Martin, R. W. Engelman and D. W. Cahill, Neurosurg. Focus, 2004, 16, E4 Search PubMed.
- L. Marchi, N. Abdala, L. Oliveira, R. Amaral, E. Coutinho and L. Pimenta, J. Neurosurg. Spine, 2013, 19, 110–118 Search PubMed.
- R. F. Frisch, I. Y. Luna, D. M. Brooks, G. Joshua and J. R. O'Brien, J. Spine Surg., 2018, 4, 62–71 CrossRef.
- A. J. Kwon, W. D. Hunter, M. Moldavsky, K. Salloum and B. Bucklen, J. Neurosurg. Spine, 2016, 24, 727–733 Search PubMed.
- Y. Hou and Z. Luo, Spine, 2009, 34, E427–E433 CrossRef.
- L. S. Chatham, V. V. Patel, C. M. Yakacki and R. Dana Carpenter, J. Biomech. Eng., 2017, 139, 0510051–0510058 CrossRef.
- S. M. Kurtz and J. N. Devine, Biomaterials, 2007, 28, 4845–4869 CrossRef CAS PubMed.
- J. M. Toth, M. Wang, B. T. Estes, J. L. Scifert, H. B. Seim and A. S. Turner, Biomaterials, 2006, 27, 324–334 CrossRef CAS PubMed.
- J. M. Sadowska, K. J. Genoud, D. J. Kelly and F. J. O'Brien, Mater. Today, 2021, 46, 136–154 CrossRef CAS.
- S. Weiner, W. Traub and H. D. Wagner, J. Struct. Biol., 1999, 126, 241–255 CrossRef CAS PubMed.
- S. Gómez, M. D. Vlad, J. López and E. Fernández, Acta Biomater., 2016, 42, 341–350 CrossRef.
- M. Fantini and M. Curto, Int. J. Interact. Des. Manuf., 2018, 12, 585–596 CrossRef.
- P. J. Rao, M. H. Pelletier, W. R. Walsh and R. J. Mobbs, Orthop. Surg., 2014, 6, 81–89 CrossRef PubMed.
- R. D. Guyer, J. J. Abitbol, D. D. Ohnmeiss and C. Yao, Spine, 2016, 41, E1146–E1150 CrossRef PubMed.
- G. R. Cizek and L. M. Boyd, Spine, 2000, 25, 2633–2636 CrossRef CAS PubMed.
- D. D. Robertson, G. B. Sharma, L. G. Gilbertson and J. D. Kang, Spine, 2009, 34, 2792–2796 CrossRef.
- M. H. Pelletier, N. Cordaro, V. M. Punjabi, M. Waites, A. Lau and W. R. Walsh, Clin. Spine Surg., 2016, 29, E208–E214 CrossRef PubMed.
- O. Nemoto, T. Asazuma, Y. Yato, H. Imabayashi, H. Yasuoka and A. Fujikawa, Eur. Spine J., 2014, 23, 2150–2155 CrossRef.
- H. Nakase, Y.-S. Park, H. Kimura, T. Sakaki and T. Morimoto, Clin. Spine Surg., 2006, 19, 353–357 Search PubMed.
- J. A. Disegi, Injury, 2000, 31, D14–D17 CrossRef.
- H. Kienapfel, C. Sprey, A. Wilke and P. Griss, J. Arthroplasty, 1999, 14, 355–368 CrossRef CAS.
- R. A. Gittens, R. Olivares-Navarrete, Z. Schwartz and B. D. Boyan, Acta Biomater., 2014, 10, 3363–3371 CrossRef PubMed.
- J. Hao, Y. Li, B. Li, X. Wang, H. Li, S. Liu, C. Liang and H. Wang, Appl. Biochem. Biotechnol., 2017, 183, 280–292 CrossRef CAS PubMed.
- D. N. Heo, W. K. Ko, H. R. Lee, S. J. Lee, D. Lee, S. H. Um, J. H. Lee, Y. H. Woo, L. G. Zhang, D. W. Lee and I. K. Kwon, J. Colloid Interface Sci., 2016, 469, 129–137 CrossRef CAS.
- P. J. Hou, K. L. Ou, C. C. Wang, C. F. Huang, M. Ruslin, E. Sugiatno, T. S. Yang and H. H. Chou, J. Mech. Behav. Biomed. Mater., 2018, 79, 173–180 CrossRef CAS PubMed.
- B. C. Bunker, P. C. Rieke, B. J. Tarasevich, A. A. Campbell, G. E. Fryxell, G. L. Graff, L. Song, J. Liu, J. W. Virden and G. L. McVay, Science, 1994, 264, 48–55 CrossRef CAS PubMed.
- S. Mann, D. D. Archibald, J. M. Didymus, T. Douglas, B. R. Heywood, F. C. Meldrum and N. J. Reeves, Science, 1993, 261, 1286–1292 CrossRef CAS.
- W. L. Murphy and D. J. Mooney, J. Am. Chem. Soc., 2002, 124, 1910–1917 CrossRef CAS PubMed.
- H. Ohgushi and A. I. Caplan, J. Biomed. Mater. Res., 1999, 48, 913–927 CrossRef CAS.
- H. Seeherman, J. Wozney and R. Li, Spine, 2002, 27, S16–S23 CrossRef PubMed.
- L. L. Hench, J. Am. Ceram. Soc., 1991, 74, 1487–1510 CrossRef CAS.
- W. L. Murphy, C. A. Simmons, D. Kaigler and D. J. Mooney, J. Dent. Res., 2004, 83, 204–210 CrossRef CAS.
- S. Szmukler-Moncler, D. Perrin, V. Ahossi, G. Magnin and J. P. Bernard, J. Biomed. Mater. Res., Part B, 2004, 68, 149–159 CrossRef CAS PubMed.
- R. Olivares-Navarrete, S. L. Hyzy, R. A. s. Gittens, J. M. Schneider, D. A. Haithcock, P. F. Ullrich, P. J. Slosar, Z. Schwartz and B. D. Boyan, Spine J., 2013, 13, 1563–1570 CrossRef.
- R. A. Gittens, R. Olivares-Navarrete, S. L. Hyzy, K. H. Sandhage, Z. Schwartz and B. D. Boyan, Connect Tissue Res., 2014, 55(Suppl 1), 164–168 CrossRef CAS.
- A. A. Zadpoor, Biomater. Sci., 2015, 3, 231–245 RSC.
- A. A. Zadpoor, Acta Biomater., 2019, 85, 41–59 CrossRef CAS PubMed.
- S. J. P. Callens, R. J. C. Uyttendaele, L. E. Fratila-Apachitei and A. A. Zadpoor, Biomaterials, 2020, 232, 119739 CrossRef CAS.
- S. Fujibayashi, M. Takemoto, M. Neo, T. Matsushita, T. Kokubo, K. Doi, T. Ito, A. Shimizu and T. Nakamura, Eur. Spine J., 2011, 20, 1486–1495 CrossRef.
- M. Takemoto, S. Fujibayashi, M. Neo, K. So, N. Akiyama, T. Matsushita, T. Kokubo and T. Nakamura, J. Neurosurg. Spine, 2007, 7, 435–443 Search PubMed.
- L. M. Bjursten, L. Rasmusson, S. Oh, G. C. Smith, K. S. Brammer and S. Jin, J. Biomed. Mater. Res., Part A, 2010, 92, 1218–1224 CrossRef PubMed.
- L. Pazos, P. Corengia and H. Svoboda, J. Mech. Behav. Biomed. Mater., 2010, 3, 416–424 CrossRef CAS PubMed.
- S. Yue, R. M. Pilliar and G. C. Weatherly, J. Biomed. Mater. Res., 1984, 18, 1043–1058 CrossRef CAS.
- J. Mouhyi, D. M. Dohan Ehrenfest and T. Albrektsson, Clin. Implant Dent. Relat. Res., 2012, 14, 170–183 CrossRef.
- H. Chouirfa, H. Bouloussa, V. Migonney and C. Falentin-Daudré, Acta Biomater., 2019, 83, 37–54 CrossRef CAS PubMed.
- G. Schmidmaier, M. Kerstan, P. Schwabe, N. Südkamp and M. Raschke, Injury, 2017, 48, 2235–2241 CrossRef CAS PubMed.
- K. Borcherding, D. Marx, L. Gätjen, N. Bormann, B. Wildemann, U. Specht, D. Salz, K. Thiel and I. Grunwald, Materials, 2019, 12, 3838 CrossRef CAS PubMed.
- X. P. Tan, Y. J. Tan, C. S. L. Chow, S. B. Tor and W. Y. Yeong, Mater. Sci. Eng., C, 2017, 76, 1328–1343 CrossRef CAS PubMed.
- X. Wang, S. Xu, S. Zhou, W. Xu, M. Leary, P. Choong, M. Qian, M. Brandt and Y. M. Xie, Biomaterials, 2016, 83, 127–141 CrossRef CAS.
- C. Donaldson, T. Santro, M. Awad and A. Morokoff, J. Spine Surg., 2024, 10, 22–29 CrossRef PubMed.
- Z. Zhang, H. Li, G. R. Fogel, D. Xiang, Z. Liao and W. Liu, Comput. Biol. Med., 2018, 95, 167–174 CrossRef.
- Z. Zhang, H. Li, G. R. Fogel, Z. Liao, Y. Li and W. Liu, World Neurosurg., 2018, 111, e581–e591 CrossRef PubMed.
- K. C. McGilvray, J. Easley, H. B. Seim, D. Regan, S. H. Berven, W. K. Hsu, T. E. Mroz and C. M. Puttlitz, Spine J., 2018, 18, 1250–1260 CrossRef PubMed.
- P. Li, W. Jiang, J. Yan, K. Hu, Z. Han, B. Wang, Y. Zhao, G. Cui, Z. Wang, K. Mao, Y. Wang and F. Cui, J. Biomed. Mater. Res., Part A, 2019, 107, 1386–1392 CrossRef CAS.
- S. H. Wu, Y. Li, Y. Q. Zhang, X. K. Li, C. F. Yuan, Y. L. Hao, Z. Y. Zhang and Z. Guo, Artif. Organs, 2013, 37, E191–E201 CAS.
- P. R. Krafft, B. Osburn, A. C. Vivas, G. Rao and P. Alikhani, Spine Surg. Relat. Res., 2020, 4, 171–177 CrossRef PubMed.
- T. Makino, S. Takenaka, Y. Sakai, H. Yoshikawa and T. Kaito, Global Spine J., 2020, 2192568220972334, DOI:10.1177/2192568220972334.
- G. M. Malham, R. M. Parker, B. Goss, C. M. Blecher and Z. E. Ballok, Spine, 2014, 39, E1303–E1310 CrossRef.
- T. V. Le, A. A. Baaj, E. Dakwar, C. J. Burkett, G. Murray, D. A. Smith and J. S. Uribe, Spine, 2012, 37, 1268–1273 CrossRef PubMed.
-
P. A. Gunatillake and R. Adhikari, Biosynthetic Polymers for Medical Applications, 2016, pp. 33–62 Search PubMed.
- S. Vadapalli, K. Sairyo, V. K. Goel, M. Robon, A. Biyani, A. Khandha and N. A. Ebraheim, Spine, 2006, 31, E992–E998 CrossRef.
- J. R. Parsons, S. Bhayani, H. Alexander and A. B. Weiss, Clin. Orthop. Relat. Res., 1985, 196, 69–76 CrossRef CAS.
- F. C. Ewald, C. B. Sledge, J. M. Corson, R. M. Rose and E. L. Radin, Clin. Orthop. Relat. Res., 1976, 115, 213–219 Search PubMed.
- H. E. Groth and J. M. Shilling, J. Orthop. Res., 1983, 1, 129–135 CrossRef CAS.
-
J. M. Toth, in PEEK Biomaterials Handbook, ed. S. M. Kurtz, William Andrew Publishing, Oxford, 2012, pp. 81–92. DOI:10.1016/B978-1-4377-4463-7.10007-7.
- L. M. Wenz, K. Merritt, S. A. Brown, A. Moet and A. D. Steffee, J. Biomed. Mater. Res., 1990, 24, 207–215 CrossRef CAS PubMed.
- D. F. Williams, A. McNamara and R. M. Turner, J. Mater. Sci. Lett., 1987, 6, 188–190 CrossRef CAS.
- O. Petillo, G. Peluso, L. Ambrosio, L. Nicolais, W. J. Kao and J. M. Anderson, J. Biomed. Mater. Res., 1994, 28, 635–646 CrossRef CAS PubMed.
- J. M. Toth, M. Wang, B. T. Estes, J. L. Scifert, H. B. Seim 3rd and A. S. Turner, Biomaterials, 2006, 27, 324–334 CrossRef CAS.
- B. J. Yoon, F. Xavier, B. R. Walker, S. Grinberg, F. P. Cammisa and C. Abjornson, Spine J., 2016, 16, 1238–1243 CrossRef PubMed.
- M. C. Kuo, C. M. Tsai, J. C. Huang and M. Chen, Mater. Chem. Phys., 2005, 90, 185–195 CrossRef CAS.
- J. W. Duncan and R. A. Bailey, Eur. Spine J., 2013, 22, 439–445 CrossRef.
- Y. Kim, Spine, 2001, 26, 1437–1442 CrossRef CAS.
- R. F. Kersten, S. M. van Gaalen, A. de Gast and F. C. Öner, Spine J., 2015, 15, 1446–1460 CrossRef PubMed.
- S. Tanida, S. Fujibayashi, B. Otsuki, K. Masamoto, Y. Takahashi, T. Nakayama and S. Matsuda, Spine, 2016, 41, E1216–e1222 CrossRef PubMed.
- Y. Assem, R. J. Mobbs, M. H. Pelletier, K. Phan and W. R. Walsh, Eur. Spine J., 2017, 26, 593–605 CrossRef.
- S. Jain, A. E. M. Eltorai, R. Ruttiman and A. H. Daniels, Orthop. Surg., 2016, 8, 278–284 CrossRef.
- W. R. Walsh, N. Bertollo, C. Christou, D. Schaffner and R. J. Mobbs, Spine J., 2015, 15, 1041–1049 CrossRef PubMed.
- C. M. F. Meers, G. B. M. Verleye, D. Smeets, H. Y. R. Van Hauwermeiren, D. Loeckx, K. Willems, V. G. M. G. G. B. Siau and P. J. M. E. Lauweryns, Int. J. Spine Surg., 2015, 9, 35 CrossRef PubMed.
- N. Aebli, J. Krebs, H. Stich, P. Schawalder, M. Walton, D. Schwenke, H. Gruner, B. Gasser and J. C. Theis, J. Biomed. Mater. Res., Part A, 2003, 66, 356–363 CrossRef PubMed.
- A. Wieling, Eur. Cells Mater., 2009, 17, 10 Search PubMed.
- D. M. Devine, J. Hahn, R. G. Richards, H. Gruner, R. Wieling and S. G. Pearce, J. Biomed. Mater. Res., Part B, 2013, 101, 591–598 CrossRef PubMed.
- A. Kienle, N. Graf and H. J. Wilke, Spine J., 2016, 16, 235–242 CrossRef.
- B. W. Cunningham, C. M. Orbegoso, A. E. Dmitriev, N. J. Hallab, J. C. Sefter, P. Asdourian and P. C. McAfee, Spine J., 2003, 3, 19–32 CrossRef PubMed.
- B. W. Cunningham, C. M. Orbegoso, A. E. Dmitriev, N. J. Hallab, J. C. Sefter and P. C. McAfee, Spine, 2002, 27, 1971–1981 CrossRef PubMed.
- H.-D. Kim, K.-S. Kim, S.-C. Ki and Y.-S. Choi, Asian Spine J., 2007, 1, 1–7 CrossRef.
- K. J. Schnake, N. Fleiter, C. Hoffmann, A. Pingel, M. Scholz, A. Langheinrich and F. Kandziora, Eur. Spine J., 2021, 30, 114–121 CrossRef PubMed.
- K. Borcherding, G. Schmidmaier, G. O. Hofmann and B. Wildemann, Injury, 2020, 52, S106–S111 CrossRef.
- C. Yao, D. Storey and T. J. Webster, Int.
J. Nanomed., 2007, 2, 487–492 CAS.
- S. W. Ha, M. Kirch, F. Birchler, K. L. Eckert, J. Mayer, E. Wintermantel, C. Sittig, I. Pfund-Klingenfuss, M. Textor, N. D. Spencer, M. Guecheva and H. Vonmont, J. Mater. Sci. Mater. Med., 1997, 8, 683–690 CrossRef CAS PubMed.
- A. Barik and N. Chakravorty, Trends Biosci. Res., 2020, 1–17 CAS.
- M. Goldberg, R. Langer and X. Jia, J. Biomater. Sci., Polym. Ed., 2007, 18, 241–268 CrossRef CAS PubMed.
- M. Qu, X. Jiang, X. Zhou, C. Wang, Q. Wu, L. Ren, J. Zhu, S. Zhu, P. Tebon, W. Sun and A. Khademhosseini, Adv. Healthcare Mater., 2020, 9, 1901714 CrossRef CAS.
- A. Dehghanghadikolaei and B. Fotovvati, Materials, 2019, 12, 1795 CrossRef CAS.
- J. H. Lee, H. L. Jang, K. M. Lee, H. R. Baek, K. Jin, K. S. Hong, J. H. Noh and H. K. Lee, Acta Biomater., 2013, 9, 6177–6187 CrossRef CAS.
- J. W. Durham III, M. J. Allen and A. Rabiei, J. Biomed. Mater. Res., Part B, 2017, 105, 560–567 CrossRef.
- S. Yu, K. P. Hariram, R. Kumar, P. Cheang and K. K. Aik, Biomaterials, 2005, 26, 2343–2352 CrossRef CAS PubMed.
- S. Barkarmo, M. Andersson, F. Currie, P. Kjellin, R. Jimbo, C. B. Johansson and V. Stenport, J. Biomater. Appl., 2014, 29, 737–747 CrossRef CAS PubMed.
- P. Johansson, R. Jimbo, Y. Naito, P. Kjellin, F. Currie and A. Wennerberg, Int. J. Nanomed., 2016, 11, 1435–1442 CrossRef CAS PubMed.
- S. Stübinger, A. Drechsler, A. Bürki, K. Klein, P. Kronen and B. von Rechenberg, J. Biomed. Mater. Res., Part B, 2016, 104, 1182–1191 CrossRef PubMed.
- M. Røkkum, A. Reigstad, C. B. Johansson and T. Albrektsson, J. Bone Jt. Surg. Br., 2003, 85, 440–447 CrossRef PubMed.
- T. Shimizu, S. Fujibayashi, S. Yamaguchi, K. Yamamoto, B. Otsuki, M. Takemoto, M. Tsukanaka, T. Kizuki, T. Matsushita, T. Kokubo and S. Matsuda, Acta Biomater., 2016, 35, 305–317 CrossRef CAS PubMed.
- T. Kizuki, T. Matsushita and T. Kokubo, J. Mater. Sci.: Mater. Med., 2015, 26, 41 CrossRef.
- M. E. Pätsi, J. A. Hautaniemi, H. M. Rahiala, T. O. Peltola and I. M. O. Kangasniemi, J. Sol-Gel Sci. Technol., 1998, 11, 55–66 CrossRef.
- T. Shimizu, S. Fujibayashi, S. Yamaguchi, B. Otsuki, Y. Okuzu, T. Matsushita, T. Kokubo and S. Matsuda, PLoS One, 2017, 12, e0184495 CrossRef.
- J. Khoury, S. R. Kirkpatrick, M. Maxwell, R. E. Cherian, A. Kirkpatrick and R. C. Svrluga, Nucl. Instrum. Methods Phys. Res., Sect. B, 2013, 307, 630–634 CrossRef CAS.
- K. L. Wong, C. T. Wong, W. C. Liu, H. B. Pan, M. K. Fong, W. M. Lam, W. L. Cheung, W. M. Tang, K. Y. Chiu, K. D. Luk and W. W. Lu, Biomaterials, 2009, 30, 3810–3817 CrossRef CAS PubMed.
- X. Wu, X. Liu, J. Wei, J. Ma, F. Deng and S. Wei, Int. J. Nanomed., 2012, 7, 1215–1225 CAS.
- R. Wauthle, J. van der Stok, S. Amin Yavari, J. Van Humbeeck, J.-P. Kruth, A. A. Zadpoor, H. Weinans, M. Mulier and J. Schrooten, Acta Biomater., 2015, 14, 217–225 CrossRef CAS PubMed.
- K. B. Sagomonyants, M. Hakim-Zargar, A. Jhaveri, M. S. Aronow and G. Gronowicz, J. Orthop. Res., 2011, 29, 609–616 CrossRef PubMed.
- M. Hanc, S. K. Fokter, M. Vogrin, A. Molicnik and G. Recnik, Eur. J. Orthop. Surg. Traumatol., 2016, 26, 1–7 CrossRef.
- J. D. Bobyn, G. J. Stackpool, S. A. Hacking, M. Tanzer and J. J. Krygier, J. Bone Jt. Surg. Br., 1999, 81, 907–914 CrossRef CAS.
- M. Lu, S. Xu, Z.-X. Lei, D. Lu, W. Cao, M. Huttula, C.-H. Hou, S.-H. Du, W. Chen, S.-W. Dai, H.-M. Li and D.-D. Jin, China Med. J., 2019, 132, 51–62 CrossRef CAS.
- S. K. Sinclair, G. J. Konz, J. M. Dawson, R. T. Epperson and R. D. Bloebaum, Spine, 2012, 37, E571–E580 CrossRef PubMed.
- X. Zou, H. Li, M. Bünger, N. Egund, M. Lind and C. Bünger, Spine J., 2004, 4, 99–105 CrossRef PubMed.
- J. Matejka, J. Zeman and J. Belatka, Acta Chir. Orthop. Traumatol. Cech., 2009, 76, 388–393 CrossRef CAS PubMed.
- M. B. Lequin, D. Verbaan and G. J. Bouma, J. Neurosurg. Spine, 2014, 20, 617–622 Search PubMed.
- C. A. Elliott, R. Fox, R. Ashforth, S. Gourishankar and A. Nataraj, J. Neurosurg. Spine, 2016, 24, 496–501 Search PubMed.
- R. F. Kersten, S. M. van Gaalen, M. P. Arts, K. C. Roes, A. de Gast, T. P. Corbin and F. C. Öner, BMC Musculoskelet. Disord., 2014, 15, 57 CrossRef.
- T. J. Webster, A. A. Patel, M. N. Rahaman and B. Sonny Bal, Acta Biomater., 2012, 8, 4447–4454 CrossRef CAS PubMed.
- M. P. Arts, J. F. C. Wolfs and T. P. Corbin, BMC Musculoskelet. Disord., 2013, 14, 244 CrossRef PubMed.
- D. J. Gorth, S. Puckett, B. Ercan, T. J. Webster, M. Rahaman and B. S. Bal, Int. J. Nanomed., 2012, 7, 4829–4840 CAS.
- R. F. M. R. Kersten, F. C. Öner, M. P. Arts, M. Mitroiu, K. C. B. Roes, A. de Gast and S. M. van Gaalen, Glob. Spine J., 2022, 12, 1687–1695 CrossRef CAS.
- M. P. Arts, J. F. C. Wolfs and T. P. Corbin, Eur. Spine J., 2017, 26, 2372–2379 CrossRef PubMed.
- G. C. Calvert, G. VanBuren Huffmon 3rd, W. M. Rambo Jr., M. W. Smith, B. J. McEntire and B. S. Bal, J. Spine Surg., 2020, 6, 33–48 CrossRef.
- W. J. Buehler, J. V. Gilfrich and R. Wiley, J. Appl. Phys., 1963, 34, 1475–1477 CrossRef CAS.
- Y. Yasenchuk, E. Marchenko, V. Gunther, A. Radkevich, O. Kokorev, S. Gunther, G. Baigonakova, V. Hodorenko, T. Chekalkin, J.-h. Kang, S. Weiss and A. Obrosov, Materials, 2019, 12, 2405 CrossRef CAS PubMed.
- A. Wadood, Adv. Mater. Sci. Eng., 2016, 2016, 4173138 Search PubMed.
-
K. Otsuka and C. M. Wayman, Shape memory materials, Cambridge university press, 1999 Search PubMed.
- A. Saigal and M. Fonte, Mater. Sci. Eng., A, 2011, 528, 5536–5550 CrossRef CAS.
- M. Niinomi, Sci. Technol. Adv. Mater., 2003, 4, 445–454 CrossRef CAS.
- V. Brailovski, S. Prokoshkin, M. Gauthier, K. Inaekyan, S. Dubinskiy, M. Petrzhik and M. Filonov, Mater. Sci. Eng., C, 2011, 31, 643–657 CrossRef CAS.
- A. Hensten-Pettersen, Eur. J. Oral Sci., 1998, 106, 707–712 CrossRef CAS.
-
S. Shabalovskaya and J. Van Humbeeck, in Shape Memory Alloys for Biomedical Applications, ed. T. Yoneyama and S. Miyazaki, Woodhead Publishing, 2009, pp. 194–233. DOI:10.1533/9781845695248.1.194.
- J. Schrooten, M. Assad, J. V. Humbeeck and M. A. Leroux, Smart Mater. Struct., 2007, 16, S145–S154 CrossRef CAS.
- M. Assad, P. Jarzem, M. A. Leroux, C. Coillard, A. V. Chernyshov, S. Charette and C. H. Rivard, J. Biomed. Mater. Res., Part B, 2003, 64, 107–120 CrossRef PubMed.
- F. H. Abduljabbar, A. M. Makhdom, M. Rajeh, A. R. Tales, J. Mathew, J. Ouellet, M. Weber and P. Jarzem, Glob. Spine J., 2017, 7, 780–786 CrossRef PubMed.
- V. Letchuman, L. Ampie, W. Choy, J. D. DiDomenico, H. R. Syed and A. L. Buchholz, Neurosurg. Focus, 2021, 50, E8 Search PubMed.
- Z. Buser, D. S. Brodke, J. A. Youssef, H.-J. Meisel, S. L. Myhre, R. Hashimoto, J.-B. Park, S. T. Yoon and J. C. Wang, J. Neurosurg. Spine, 2016, 25, 509–516 Search PubMed.
- E. D. Arrington, W. J. Smith, H. G. Chambers, A. L. Bucknell and N. A. Davino, Clin. Orthop. Relat. Res., 1996, 300–309, DOI:10.1097/00003086-199608000-00037.
- R. Dimitriou, G. I. Mataliotakis, A. G. Angoules, N. K. Kanakaris and P. V. Giannoudis, Injury, 2011, 42(Suppl 2), S3–15 CrossRef PubMed.
- J. S. Silber, D. G. Anderson, S. D. Daffner, B. T. Brislin, J. M. Leland, A. S. Hilibrand, A. R. Vaccaro and T. J. Albert, Spine, 2003, 28, 134–139 CrossRef PubMed.
- P. A. Robertson and A. C. Wray, Spine, 2001, 26, 1473–1476 CrossRef CAS.
- A. Kannan, S. N. Dodwad and W. K. Hsu, J. Spinal Disord. Tech, 2015, 28, 163–170 CrossRef.
- M. K. Aghi, B. P. Walcott, B. V. Nahed, G. L. Cvetanovich, K. T. Kahle, N. Redjal and J. V. Coumans, J. Clin. Neurosci., 2011, 18, 1193–1196 CrossRef PubMed.
- K. Y. Ha, J. S. Lee and K. W. Kim, Spine, 2009, 34, 1663–1668 CrossRef PubMed.
- K. W. Kim, K. Y. Ha, M. S. Moon, Y. S. Kim, S. Y. Kwon and Y. K. Woo, Spine, 1999, 24, 428–433 CrossRef CAS.
- D. Delawi, M. C. Kruyt, Y. Huipin, K. L. Vincken, J. D. de Bruijn, F. C. Oner and W. J. Dhert, Tissue Eng., Part C, 2013, 19, 821–828 CrossRef CAS PubMed.
- M. Street, R. Gao, W. Martis, J. Munro, D. Musson, J. Cornish and J. Ferguson, Spine Deform., 2017, 5, 231–237 CrossRef.
- E. M. Fragkakis, J. J. El-Jawhari, R. A. Dunsmuir, P. A. Millner, A. S. Rao, K. T. Henshaw, I. Pountos, E. Jones and P. V. Giannoudis, PLoS One, 2018, 13, e0197969 CrossRef.
- K.-W. Wong, H.-W. Wang, C.-S. Chien, C.-H. Li, C.-B. Li and C.-L. Lin, Expert Rev. Med. Devices, 2024, 1–8, DOI:10.1080/17434440.2024.2367688.
- R. M. Duarte, P. Varanda, R. L. Reis, A. R. C. Duarte and J. Correia-Pinto, Tissue Eng., Part B, 2017, 23, 540–551 CrossRef.
- C.-C. Niu, S.-S. Lin, W.-J. Chen, S.-J. Liu, L.-H. Chen, C.-Y. Yang, C.-J. Wang, L.-J. Yuan, P.-H. Chen and H.-Y. Cheng, J. Orthop. Surg. Res., 2015, 10, 111 CrossRef.
- Y. Gu, L. Chen, H.-Y. Niu, X.-F. Shen and H.-L. Yang, J. Biomater. Appl., 2016, 30, 1251–1260 CrossRef CAS.
- A. Tuchman, D. S. Brodke, J. A. Youssef, H.-J. Meisel, J. R. Dettori, J.-B. Park, S. T. Yoon and J. C. Wang, Glob. Spine J., 2016, 6, 592–606 CrossRef.
- W. K. Hsu, M. S. Nickoli, J. C. Wang, J. R. Lieberman, H. S. An, S. T. Yoon, J. A. Youssef, D. S. Brodke and C. M. McCullough, Glob. Spine J., 2012, 2, 239–248 CrossRef.
- C. Delloye, O. Cornu, V. Druez and O. Barbier, J. Bone Joint. Surg. Br., 2007, 89-B, 574–580 CrossRef PubMed.
- V. Grover, A. Kapoor, R. Malhotra and S. Sachdeva, Indian J. Dent. Res., 2011, 22, 496 CrossRef PubMed.
- A. S. Greenwald, S. D. Boden, V. M. Goldberg, Y. Khan, C. T. Laurencin and R. N. Rosier, J. Bone Jt. Surg. Am., 2001, 83-A(Suppl 2 Pt 2), 98–103 CrossRef.
- T. Kurien, R. G. Pearson and B. E. Scammell, Bone Joint J., 2013, 95-B, 583–597 CrossRef CAS.
- W. G. De Long Jr., T. A. Einhorn, K. Koval, M. McKee, W. Smith, R. Sanders and T. Watson, J. Bone Jt. Surg. Am., 2007, 89, 649–658 CrossRef.
- M. R. Urist, Science, 1965, 150, 893–899 CrossRef CAS.
- B. Peterson, P. G. Whang, R. Iglesias, J. C. Wang and J. R. Lieberman, J. Bone Jt. Surg., 2004, 86, 2243–2250 CrossRef.
- K. Tilkeridis, P. Touzopoulos, A. Ververidis, S. Christodoulou, K. Kazakos and G. I. Drosos, World J. Orthop., 2014, 5, 30–37 CrossRef PubMed.
- P. V. Giannoudis, H. Dinopoulos and E. Tsiridis, Injury, 2005, 36(Suppl 3), S20–S27 CrossRef.
- M. C. Makhni, J. M. Caldwell, C. Saifi, C. R. Fischer, R. A. Lehman, L. G. Lenke and F. Y. Lee, Regen. Med., 2016, 11, 211–222 CrossRef CAS.
- F. Baumann, W. Krutsch, C. Pfeifer, C. Neumann, M. Nerlich and M. Loibl, Acta Chir. Orthop. Traumatol. Cech., 2015, 82, 119–125 CrossRef CAS PubMed.
- B. Aghdasi, S. R. Montgomery, M. D. Daubs and J. C. Wang, Surgeon, 2013, 11, 39–48 CrossRef CAS.
- R. M. Ajiboye, J. T. Hamamoto, M. A. Eckardt and J. C. Wang, Eur. Spine J., 2015, 24, 2567–2572 CrossRef PubMed.
- A. Gupta, N. Kukkar, K. Sharif, B. J. Main, C. E. Albers and S. F. El-Amin III, World J. Orthop., 2015, 6, 449 CrossRef.
- C. E. Gillman and A. C. Jayasuriya, Mater. Sci. Eng., C, 2021, 130, 112466 CrossRef CAS PubMed.
- T. T. Roberts and A. J. Rosenbaum, Organogenesis, 2012, 8, 114–124 CrossRef PubMed.
- W. Wang and K. W. K. Yeung, Bioact. Mater., 2017, 2, 224–247 Search PubMed.
- H. C. Pape, A. Evans and P. Kobbe, J. Orthop. Trauma, 2010, 24(Suppl 1), S36–S40 CrossRef PubMed.
- H.-S. Sohn and J.-K. Oh, Biomater. Res., 2019, 23, 9 CrossRef.
- J. M. Bouler, P. Pilet, O. Gauthier and E. Verron, Acta Biomater., 2017, 53, 1–12 CrossRef CAS.
- H. H. K. Xu, M. D. Weir, E. F. Burguera and A. M. Fraser, Biomaterials, 2006, 27, 4279–4287 CrossRef CAS.
- E. U. Conrad, D. R. Gretch, K. R. Obermeyer, M. S. Moogk, M. Sayers, J. J. Wilson and D. M. Strong, J. Bone Jt. Surg. Am., 1995, 77, 214–224 CrossRef CAS.
- W. R. Moore, S. E. Graves and G. I. Bain, ANZ J. Surg., 2001, 71, 354–361 CrossRef CAS.
- A. W. James, G. LaChaud, J. Shen, G. Asatrian, V. Nguyen, X. Zhang, K. Ting and C. Soo, Tissue Eng., Part B, 2016, 22, 284–297 CrossRef CAS PubMed.
- M. P. Bostrom and D. A. Seigerman, HSS J., 2005, 1, 9–18 CrossRef PubMed.
- J. R. Jones, D. S. Brauer, L. Hupa and D. C. Greenspan, Int. J. Appl. Glass Sci., 2016, 7, 423–434 CrossRef CAS.
- R. Z. LeGeros, Clin. Orthop. Relat. Res., 2002, 81–98, DOI:10.1097/00003086-200202000-00009.
- G. Zimmermann and A. Moghaddam, Injury, 2011, 42(Suppl 2), S16–S21 CrossRef PubMed.
- R. M. Pilliar, M. J. Filiaggi, J. D. Wells, M. D. Grynpas and R. A. Kandel, Biomaterials, 2001, 22, 963–972 CrossRef CAS.
- E. P. Frankenburg, S. A. Goldstein, T. W. Bauer, S. A. Harris and R. D. Poser, J. Bone Jt. Surg. Am., 1998, 80, 1112–1124 CrossRef CAS PubMed.
- M. Ikenaga, P. Hardouin, J. Lemaître, H. Andrianjatovo and B. Flautre, J. Biomed. Mater. Res., 1998, 40, 139–144 CrossRef CAS PubMed.
- M. A. Plantz, E. B. Gerlach and W. K. Hsu, Int. J. Spine Surg., 2021, 15, 104 CrossRef.
- R. A. Kapur, R. Amirfeyz, V. Wylde, A. W. Blom, I. W. Nelson and J. Hutchinson, Arch. Orthop. Trauma Surg., 2010, 130, 641–647 CrossRef PubMed.
- L. A. van Dijk, D. Barbieri, F. Barrère-de Groot, H. Yuan, R. Oliver, C. Christou, W. R. Walsh and J. D. de Bruijn, J. Biomed. Mater. Res., Part B, 2019, 107, 2080–2090 CrossRef CAS.
- A. M. Lehr, F. C. Oner, D. Delawi, R. K. Stellato, E. A. Hoebink, D. H. R. Kempen, J. L. C. van Susante, R. M. Castelein and M. C. Kruyt, Spine, 2020, 45, 944–951 CrossRef PubMed.
- J. E. Inglis, A. M. Goodwin, S. N. Divi and W. K. Hsu, Int. J. Spine Surg., 2023, 17, S18 CrossRef PubMed.
- A. M. Lehr, F. C. Oner, D. Delawi, R. K. Stellato, E. A. Hoebink, D. H. R. Kempen, J. L. C. van Susante, R. M. Castelein and M. C. Kruyt, Spine, 2020, 45, 1403–1410 CrossRef PubMed.
- L. A. van Dijk, R. Duan, X. Luo, D. Barbieri, M. Pelletier, C. Christou, A. J. W. P. Rosenberg, H. Yuan, F. Barrèrre-de Groot, W. R. Walsh and J. D. de Bruijn, JOR Spine, 2018, 1, e1039 CrossRef.
- B. H. Ziran, W. R. Smith and S. J. Morgan, J. Trauma, 2007, 63, 1324–1328 CAS.
- W. J. Chen, T. T. Tsai, L. H. Chen, C. C. Niu, P. L. Lai, T. S. Fu and K. McCarthy, Spine, 2005, 30, 2293–2297 CrossRef PubMed.
- L. Y. Dai and L. S. Jiang, Spine, 2008, 33, 1299–1304 CrossRef.
- A. E. Jakus, A. L. Rutz, S. W. Jordan, A. Kannan, S. M. Mitchell, C. Yun, K. D. Koube, S. C. Yoo, H. E. Whiteley, C.-P. Richter, R. D. Galiano, W. K. Hsu, S. R. Stock, E. L. Hsu and R. N. Shah, Sci. Transl. Med., 2016, 8, 358ra127–358ra127 Search PubMed.
- L. L. Hench, R. J. Splinter, W. C. Allen and T. K. Greenlee, J. Biomed. Mater. Res., 1971, 5, 117–141 CrossRef.
- I. D. Xynos, M. V. Hukkanen, J. J. Batten, L. D. Buttery, L. L. Hench and J. M. Polak, Calcif. Tissue Int., 2000, 67, 321–329 CrossRef CAS.
- I. D. Xynos, A. J. Edgar, L. D. Buttery, L. L. Hench and J. M. Polak, J. Biomed. Mater. Res., 2001, 55, 151–157 CrossRef CAS.
- Z. Qiu, H. Yang, J. Wu, L. Wei and J. Li, J. Int. Med. Res., 2009, 37, 737–745 CrossRef CAS PubMed.
- Ö. H. Andersson and I. Kangasniemi, J. Biomed. Mater. Res., 1991, 25, 1019–1030 CrossRef PubMed.
- M. R. Filgueiras, G. La Torre and L. L. Hench, J. Biomed. Mater. Res., 1993, 27, 445–453 CrossRef CAS PubMed.
- L. L. Hench, R. J. Splinter, W. C. Allen and T. K. Greenlee, J. Biomed. Mater. Res., Part A, 1971, 5, 117–141 CrossRef.
- C. Xu, P. Su, X. Chen, Y. Meng, W. Yu, A. P. Xiang and Y. Wang, Biomaterials, 2011, 32, 1051–1058 CrossRef CAS PubMed.
- L. A. van Dijk, F. Barrère-de Groot, A. Rosenberg, M. Pelletier, C. Christou, J. D. de Bruijn and W. R. Walsh, Clin. Spine Surg., 2020, 33, E276–e287 CrossRef PubMed.
- J. H. Lee, H. S. Ryu, J. H. Seo, D. Y. Lee, B. S. Chang and C. K. Lee, Clin. Orthop. Surg., 2014, 6, 87–95 CrossRef PubMed.
- J. M. Schmitt, D. C. Buck, S.-P. Joh, S. E. Lynch and J. O. Hollinger, J. Periodontol., 1997, 68, 1043–1053 CrossRef CAS.
- A. Moreira-Gonzalez, C. Lobocki, K. Barakat, L. Andrus, M. Bradford, M. Gilsdorf and I. T. Jackson, J. Craniofac. Surg., 2005, 16, 63–70 CrossRef PubMed.
- H. Kobayashi, A. S. Turner, H. B. Seim III, T. Kawamoto and T. W. Bauer, J. Biomed. Mater. Res., Part A, 2010, 92, 596–603 CrossRef PubMed.
- M. R. Urist and B. S. Strates, J. Dent. Res., 1971, 50, 1392–1406 CrossRef CAS PubMed.
- K. R. Garrison, I. Shemilt, S. Donell, J. J. Ryder, M. Mugford, I. Harvey, F. Song and V. Alt, Cochrane Database Syst. Rev., 2010, 6, CD006950 Search PubMed.
- G. E. Friedlaender, C. R. Perry, J. D. Cole, S. D. Cook, G. Cierny, G. F. Muschler, G. A. Zych, J. H. Calhoun, A. J. LaForte and S. Yin, J. Bone Jt. Surg. Am., 2001, 83-A(Suppl 1), S151–S158 Search PubMed.
- S. A. Gittens, K. Bagnall, J. R. Matyas, R. Löbenberg and H. Uludag, J. Controlled Release, 2004, 98, 255–268 CrossRef CAS.
- J. P. Gorski, Crit. Rev. Oral Biol. Med., 1998, 9, 201–223 CrossRef CAS PubMed.
- J. P. Gorski, D. Griffin, G. Dudley, C. Stanford, R. Thomas, C. Huang, E. Lai, B. Karr and M. Solursh, J. Biol. Chem., 1990, 265, 14956–14963 CrossRef CAS.
- Y. Liu, E. B. Hunziker, P. Layrolle, J. D. De Bruijn and K. De Groot, Tissue Eng., 2004, 10, 101–108 CrossRef CAS.
- T. Matsumoto, M. Okazaki, M. Inoue, S. Yamaguchi, T. Kusunose, T. Toyonaga, Y. Hamada and J. Takahashi, Biomaterials, 2004, 25, 3807–3812 CrossRef CAS PubMed.
- A. Sachse, A. Wagner, M. Keller, O. Wagner, W. D. Wetzel, F. Layher, R. A. Venbrocks, P. Hortschansky, M. Pietraszczyk, B. Wiederanders, H. J. Hempel, J. Bossert, J. Horn, K. Schmuck and J. Mollenhauer, Bone, 2005, 37, 699–710 CrossRef CAS PubMed.
- S. Cecchi, S. J. Bennet and M. Arora, J. Orthop. Translat., 2016, 4, 28–34 CrossRef.
- A. R. Vaccaro, P. G. Whang, T. Patel, F. M. Phillips, D. G. Anderson, T. J. Albert, A. S. Hilibrand, R. S. Brower, M. F. Kurd, A. Appannagari, M. Patel and J. S. Fischgrund, Spine J., 2008, 8, 457–465 CrossRef.
- J. Munns, D. K. Park and K. Singh, Orthop. Res. Rev., 2009, 1, 11–21 CAS.
- J. W. Hustedt and D. J. Blizzard, Yale J. Biol. Med., 2014, 87, 549–561 Search PubMed.
- J. Everding, J. Stolberg-Stolberg, M. J. Raschke and R. Stange, Unfallchirurg, 2019, 122, 534–543 CrossRef CAS.
- E. R. Balmayor and M. van Griensven, Front. Bioeng. Biotechnol., 2015, 3, 9 Search PubMed.
- E. Tsiridis, N. Upadhyay and P. Giannoudis, Injury, 2007, 38(Suppl 1), S11–S25 CrossRef.
- K. D. Hankenson, K. Gagne and M. Shaughnessy, Adv. Drug Delivery Rev., 2015, 94, 3–12 CrossRef CAS PubMed.
- E. J. Carragee, E. L. Hurwitz and B. K. Weiner, J. Spine, 2011, 11, 471–491 CrossRef.
- L. Lao, J. R. Cohen, Z. Buser, D. S. Brodke, S. T. Yoon, J. A. Youssef, J.-B. Park, H.-J. Meisel and J. C. Wang, Glob. Spine J., 2018, 8, 137–141 CrossRef.
- R. Fu, S. Selph, M. McDonagh, K. Peterson, A. Tiwari, R. Chou and M. Helfand, Ann. Intern. Med., 2013, 158, 890–902 CrossRef.
- N. E. Epstein, Surg. Neurol. Int., 2014, 5, S552–S560 CrossRef PubMed.
- J. K. Burkus, M. F. Gornet, C. A. Dickman and T. A. Zdeblick, J. Spinal Disord. Tech., 2002, 15, 337–349 CrossRef.
- J. K. Burkus, S. E. Heim, M. F. Gornet and T. A. Zdeblick, J. Spinal Disord. Tech., 2003, 16, 113–122 CrossRef.
- S. M. Hansen and R. C. Sasso, J. Spinal Disord. Tech., 2006, 19, 130–134 CrossRef.
- R. Vaidya, A. Sethi, S. Bartol, M. Jacobson, C. Coe and J. G. Craig, J. Spinal Disord. Tech., 2008, 21, 557–562 CrossRef.
- R. Vaidya, R. Weir, A. Sethi, S. Meisterling, W. Hakeos and C. D. Wybo, J. Bone Jt. Surg., Br. Vol., 2007, 89-B, 342–345 CrossRef PubMed.
- E. J. Carragee, E. L. Hurwitz and B. K. Weiner, Spine J., 2011, 11, 471–491 CrossRef PubMed.
- E. J. Carragee, K. A. Mitsunaga, E. L. Hurwitz and G. J. Scuderi, Spine J., 2011, 11, 511–516 CrossRef.
- C. D. Jarrett, J. G. Heller and L. Tsai, J. Spinal Disord. Tech., 2009, 22, 559–564 CrossRef.
- B. U. Kang, W. C. Choi, S. H. Lee, S. H. Jeon, J. D. Park, D. H. Maeng and Y. G. Choi, J. Neurosurg. Spine, 2009, 10, 60–65 Search PubMed.
- R. C. Sasso, N. M. Best, P. V. Mummaneni, T. M. Reilly and S. M. Hussain, Spine, 2005, 30, 670–674 CrossRef PubMed.
- R. C. Sasso, S. H. Kitchel and E. G. Dawson, Spine, 2004, 29, 113–122 CrossRef ; discussion 121–112.
- L. Y. Carreon, S. D. Glassman, M. Djurasovic, M. J. Campbell, R. M. Puno, J. R. Johnson and J. R. Dimar 2nd, Spine, 2009, 34, 238–243 CrossRef.
- M. P. Garrett, U. K. Kakarla, R. W. Porter and V. K. Sonntag, Neurosurgery, 2010, 66, 1044–1049 CrossRef PubMed ; discussion 1049.
- M. C. Simmonds, J. V. Brown, M. K. Heirs, J. P. Higgins, R. J. Mannion, M. A. Rodgers and L. A. Stewart, Ann. Intern. Med., 2013, 158, 877–889 CrossRef.
- R. W. Haid Jr., C. L. Branch Jr., J. T. Alexander and J. K. Burkus, Spine J., 2004, 4, 527–538 CrossRef PubMed ; discussion 538–529.
- V. Joseph and Y. R. Rampersaud, Spine, 2007, 32, 2885–2890 CrossRef.
- H. J. Meisel, M. Schnöring, C. Hohaus, Y. Minkus, A. Beier, T. Ganey and U. Mansmann, Eur. Spine J., 2008, 17, 1735–1744 CrossRef.
- D. A. Wong, A. Kumar, S. Jatana, G. Ghiselli and K. Wong, Spine J., 2008, 8, 1011–1018 CrossRef.
- P. T. Geibel, D. L. Boyd and V. Slabisak, J. Spinal Disord. Tech., 2009, 22, 315–320 CrossRef.
- A. T. Villavicencio, S. Burneikiene, E. L. Nelson, K. R. Bulsara, M. Favors and J. Thramann, J. Neurosurg. Spine, 2005, 3, 436–443 Search PubMed.
- J. A. Rihn, J. Makda, J. Hong, R. Patel, A. S. Hilibrand, D. G. Anderson, A. R. Vaccaro and T. J. Albert, Eur. Spine J., 2009, 18, 1629–1636 CrossRef.
- J. A. Rihn, R. Patel, J. Makda, J. Hong, D. G. Anderson, A. R. Vaccaro, A. S. Hilibrand and T. J. Albert, Spine J., 2009, 9, 623–629 CrossRef PubMed.
- S. Balseiro and E. W. Nottmeier, Spine J., 2010, 10, e6–e10 CrossRef PubMed.
- G. A. Helm, H. Dayoub and J. A. Jane, Neurosurg. Focus, 2001, 10, E4 CAS.
- F. Kandziora, G. Schmidmaier, G. Schollmeier, H. Bail, R. Pflugmacher, T. Görke, M. Wagner, M. Raschke, T. Mittlmeier and N. P. Haas, Spine, 2002, 27, 1710–1723 CrossRef.
- B. M. Holzapfel, J. C. Reichert, J. T. Schantz, U. Gbureck, L. Rackwitz, U. Nöth, F. Jakob, M. Rudert, J. Groll and D. W. Hutmacher, Adv. Drug Delivery Rev., 2013, 65, 581–603 CrossRef CAS PubMed.
- S. A. Abbah, C. X. L. Lam, D. W. Hutmacher, J. C. H. Goh and H.-K. Wong, Biomaterials, 2009, 30, 5086–5093 CrossRef CAS.
- J. N. Zara, R. K. Siu, X. Zhang, J. Shen, R. Ngo, M. Lee, W. Li, M. Chiang, J. Chung, J. Kwak, B. M. Wu, K. Ting and C. Soo, Tissue Eng., Part A, 2011, 17, 1389–1399 CrossRef CAS.
- B.-B. Seo, J.-T. Koh and S.-C. Song, Biomaterials, 2017, 122, 91–104 CrossRef CAS PubMed.
- Z. Wang, Z. Wang, W. W. Lu, W. Zhen, D. Yang and S. Peng, NPG Asia Mater., 2017, 9, e435–e435 CrossRef CAS.
- I. R. Calori, G. Braga, P. d. C. C. de Jesus, H. Bi and A. C. Tedesco, Eur. Polym. J., 2020, 129, 109621 CrossRef CAS.
- S. Vukicevic, H. Oppermann, D. Verbanac, M. Jankolija, I. Popek, J. Curak, J. Brkljacic, M. Pauk, I. Erjavec, I. Francetic, I. Dumic-Cule, M. Jelic, D. Durdevic, T. Vlahovic, R. Novak, V. Kufner, T. Bordukalo Niksic, M. Kozlovic, Z. Banic Tomisic, J. Bubic-Spoljar, I. Bastalic, S. Vikic-Topic, M. Peric, M. Pecina and L. Grgurevic, Int. Orthop., 2014, 38, 635–647 CrossRef.
- J. R. Dimar 2nd, S. D. Glassman, J. K. Burkus, P. W. Pryor, J. W. Hardacker and L. Y. Carreon, Spine J., 2009, 9, 880–885 CrossRef PubMed.
- S. L. Ondra and S. Marzouk, Neurosurg. Focus, 2003, 15, 1–5 Search PubMed.
- N. M. Raizman, J. R. O'Brien, K. L. Poehling-Monaghan and D. Y. Warren, J. Am. Acad. Orthop. Surg., 2009, 17, 494–503 CrossRef.
- M. G. Kaiser, M. W. Groff, W. C. Watters, Z. Ghogawala, P. V. Mummaneni, A. T. Dailey, T. F. Choudhri, J. C. Eck, A. Sharan, J. C. Wang, S. S. Dhall and D. K. Resnick, J. Neurosurg. Spine, 2014, 21, 106 Search PubMed.
- T. H. Smit, T. A. Engels, P. I. Wuisman and L. E. Govaert, Spine, 2008, 33, 14–18 CrossRef.
- B. C. Cheng, S. Jaffee, S. Averick, I. Swink, S. Horvath and R. Zhukauskas, Spine J., 2020, 20, 457–464 CrossRef PubMed.
- K. Tanaka, M. Takemoto, S. Fujibayashi, M. Neo, Y. Shikinami and T. Nakamura, Spine, 2011, 36, 441–447 CrossRef.
- D. C. Fredericks, E. B. Petersen, N. Sahai, K. G. N. Corley, N. DeVries, N. M. Grosland and J. D. Smucker, Iowa Orthop. J., 2013, 33, 25–32 Search PubMed.
- J. D. Smucker, E. B. Petersen, J. V. Nepola and D. C. Fredericks, Iowa Orthop. J., 2012, 32, 61–68 Search PubMed.
- A. M. Riordan, R. Rangarajan, J. W. Balts, W. K. Hsu and P. A. Anderson, J. Orthop. Res., 2013, 31, 1261–1269 CrossRef PubMed.
- S. Lee, X. Zhang, J. Shen, A. W. James, C. G. Chung, R. Hardy, C. Li, C. Girgius, Y. Zhang, D. Stoker, H. Wang, B. M. Wu, B. Peault, K. Ting and C. Soo, Stem Cells, 2015, 33, 3158–3163 CrossRef CAS.
- W. Li, M. Lee, J. Whang, R. K. Siu, X. Zhang, C. Liu, B. M. Wu, J. C. Wang, K. Ting and C. Soo, Tissue Eng., Part A, 2010, 16, 2861–2870 CrossRef CAS PubMed.
- Q. Ye, K. Chen, W. Huang, Y. He, M. Nong, C. Li and T. Liang, Exp. Ther. Med., 2015, 9, 25–32 CrossRef.
- Y. Wen, S. Xun, M. Haoye, S. Baichuan, C. Peng, L. Xuejian, Z. Kaihong, Y. Xuan, P. Jiang and L. Shibi, Biomater. Sci., 2017, 5, 1690–1698 RSC.
- H. Yoshikawa, N. Tamai, T. Murase and A. Myoui, J. R. Soc., Interface, 2009, 6(Suppl 3), S341–S348 CAS.
- F. Salamanna, D. Contartese, G. Tedesco, A. Ruffilli, M. Manzetti, G. Viroli, M. Traversari, C. Faldini and G. Giavaresi, JOR Spine, 2024, 7, e1347 CrossRef CAS PubMed.
- M. A. Plantz and W. K. Hsu, Curr. Rev. Musculoskelet. Med., 2020, 13, 318–325 CrossRef.
- E. J. Kerr 3rd, A. Jawahar, T. Wooten, S. Kay, D. A. Cavanaugh and P. D. Nunley, J. Surg. Orthop. Adv., 2011, 20, 193–197 Search PubMed.
- J. M. Ammerman, J. Libricz and M. D. Ammerman, Clin. Neurol. Neurosurg., 2013, 115, 991–994 CrossRef PubMed.
- B. Johnstone, N. Zhang, E. I. Waldorff, E. Semler, A. Dasgupta, M. Betsch, P. Punsalan, H. Cho, J. T. Ryaby and J. Yoo, Int. J. Spine Surg., 2020, 14, 213–221 CrossRef.
- C. Lin, N. Zhang, E. I. Waldorff, P. Punsalan, D. Wang, E. Semler, J. T. Ryaby, J. Yoo and B. Johnstone, JOR Spine, 2020, 3, e1084 CrossRef PubMed.
- P. C. Hsieh, Z. Buser, A. C. Skelly, E. D. Brodt, D. Brodke, H. J. Meisel, J. B. Park, S. T. Yoon and J. C. Wang, Global Spine J., 2019, 9, 22s–38s CrossRef PubMed.
- B. W. Cunningham, B. L. Atkinson, N. Hu, J. Kikkawa, L. Jenis, J. Bryant, P. O. Zamora and P. C. McAfee, J. Neurosurg. Spine, 2009, 10, 300–307 Search PubMed.
- P. Lauweryns and Y. Raskin, Int. J. Spine Surg., 2015, 9, 2 CrossRef PubMed.
- J. J. Qian and R. S. Bhatnagar, J. Biomed. Mater. Res., 1996, 31, 545–554 CrossRef CAS.
- A. Kadam, P. W. Millhouse, C. K. Kepler, K. E. Radcliff, M. G. Fehlings, M. E. Janssen, R. C. Sasso, J. J. Benedict and A. R. Vaccaro, Int. J. Spine Surg., 2016, 10, 33 CrossRef.
|
This journal is © The Royal Society of Chemistry 2024 |
Click here to see how this site uses Cookies. View our privacy policy here.