DOI:
10.1039/D4BM00936C
(Review Article)
Biomater. Sci., 2024,
12, 5433-5449
Smart self-defensive coatings with bacteria-triggered antimicrobial response for medical devices†
Received
15th July 2024
, Accepted 7th September 2024
First published on 25th September 2024
Abstract
Bacterial colonization and biofilm formation on medical devices represent one of the most urgent and critical challenges in modern healthcare. These issues not only pose serious threats to patient health by increasing the risk of infections but also exert a considerable economic burden on national healthcare systems due to prolonged hospital stays and additional treatments. To address this challenge, there is a need for smart, customized biomaterials for medical device fabrication, particularly through the development of surface modification strategies that prevent bacterial adhesion and the growth of mature biofilms. This review explores three bioinspired approaches through which antibacterial and antiadhesive coatings can be engineered to exhibit smart, stimuli-responsive features. This responsiveness is greatly valuable as it provides the coatings with a controlled, on-demand antibacterial response that is activated only in the presence of bacteria, functioning as self-defensive coatings. Such coatings can be designed to release antibacterial agents or change their surface properties/conformation in response to specific stimuli, like changes in pH, temperature, or the presence of bacterial enzymes. This targeted approach minimizes the risk of developing antibiotic resistance and reduces the need for continuous, high-dose antibacterial treatments, thereby preserving the natural microbiome and further reducing healthcare costs. The final part of the review reports a critical analysis highlighting the potential improvements and future evolutions regarding antimicrobial self-defensive coatings and their validation.
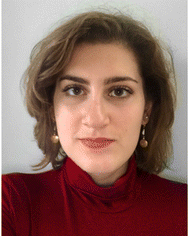 Maria Antonia Cassa | Maria Antonia Cassa received her Master's Degree in Biomedical Engineering in 2020 from Politecnico di Torino (Italy). She is currently a PhD candidate in Bioengineering and Medical-Surgical Sciences at the Department of Mechanical and Aerospace Engineering (Politecnico di Torino), focusing on surface functionalization strategies for biomaterials used in different biomedical applications. She is part of the BIOINSIDE LAB research group led by prof. Gianluca Ciardelli and prof. Valeria Chiono, whose main topics revolve around innovative biomaterials, nanomedicine, tissue engineering and in vitro tissue models. |
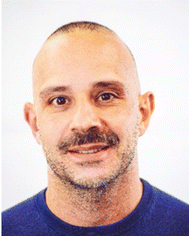 Piergiorgio Gentile | Piergiorgio Gentile is a Reader in Bionanotechnologies at Newcastle University, UK. He obtained his B.Sc., M.Sc., and Ph.D. in Biomedical Engineering from Politecnico di Torino, Italy. He moved to the UK in 2012 after being awarded with an IEF Marie Curie Fellowship. His research deals with the nano- and micro-scale design, manufacturing and surface functionalisation of biomimetic constructs for tissue engineering. He is author of >100 papers (H-index = 35) in the leading journals in Bioengineering, 5 book chapter and his research has led to 3 patents, >50 oral presentations at national & international conferences including >10 as invited speaker. |
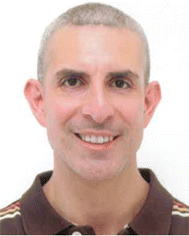 Joel Girón-Hernández | Joel Girón-Hernández is an Assistant Professor at the Faculty of Health and Life Sciences at Northumbria University, United Kingdom. His scientific activities are interdisciplinary, ranging from the valorisation of plant bio-waste to analytical quality determinations of food composition. Since obtaining his PhD in Food Engineering from the Universitat Politècnica de València, Spain, he has collaborated with various industrial partners, enabling him to translate his research from the lab to industry. |
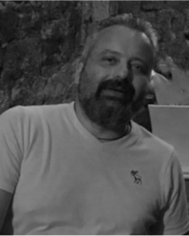 Gianluca Ciardelli | Gianluca Ciardelli graduated in Chemistry at the University of Pisa (Italy) and obtained a PhD in Natural Sciences at the ETH (Zurich, Switzerland). He is now Full Professor in Biomedical Engineering and coordinates the “Materials in Bionanotechnology and Biomedical Lab-Bioinside” group at the Department of Mechanical and Aerospace Engineering (Politecnico di Torino). He has over 20-years’ experience in research in Biomaterials for Tissue Engineering, Controlled Drug Delivery, and more recently Nanomedicine, Tissue and Organ Models. The scopus database reports over 240 documents, 8 book chapters; 12 patents are cited by espacenet. His h-index is 48 with over 8900 citations (SCOPUS). |
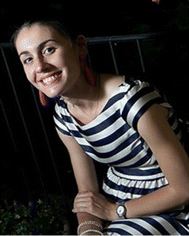 Irene Carmagnola | Irene Carmagnola earned her Ph.D. in Biomedical Engineering in 2013 and she is currently assistant professor at Politecnico di Torino. Her research activity is primarily focused on the design, fabrication, and characterization of polymeric-based scaffolds and on nanotechnologies applied to the biomedical fields, particularly Tissue Engineering. Specifically, she has utilized conventional and additive manufacturing techniques to engineer scaffolds. She also has studied the preparation of hydrogels to be used as “ink” for 3D printing techniques to obtain cellularized constructs. In parallel, she has developed nanostructured coatings using innovative strategies for various applications, such as coronary stents, wound healing and antibacterial surfaces. |
Introduction
Short and long-term invasive medical devices are often related to a major complication, which is surface colonization from biofilm-forming bacteria. Among the wide variety of affected medical devices, cardiac valves and pacemakers,1–4 silicone prosthesis,5–7 dental8 and orthopaedic implants,9 catheters for several anatomical regions,10,11 pulmonary ventilators endotracheal tubes,12 ophthalmological contact lenses,13 and also suture threads14 can be considered the most critical. Once bacteria adhere to the device surface, they initiate the formation of a biofilm, organizing themselves into colonies. This biofilm enhances their survival through enhanced adhesion, access to nutrients, and increased resistance to external stresses.
Particularly, bacterial attachment can be considered the first step of biofilm formation, triggering the production of extracellular matrix (ECM) composed of substances like polysaccharides, proteins, and DNA that are secreted by the bacteria themselves or by the host organism.15 Furthermore, the ECM can embed and shield microcolonies while maintaining their cohesion.16 The process of biofilm formation is continuously supported by communication among and within different bacterial species, a phenomenon known as quorum sensing.17 The onset frequently takes place in healthcare settings, where it is impossible to completely eradicate every potential source of contamination from the surroundings, such as unsterile instruments, administered intravenous fluids, and weakly aseptic medical procedures. Also, pathogens are naturally present on human skin and some anatomical regions can be concealed, hence difficult to reach and clean.18 Such infections are termed nosocomial and pose serious threats to the health and well-being of patients, especially considering that they might be already debilitated or immunosuppressed and could potentially perish in the worst cases.19 In addition to the infection itself, the overgrowth of bacterial biofilm on medical devices leads to severe malfunctions and aesthetical deformities, causing unbearable discomforts for patients, thus necessitating their replacement or removal.
All these serious issues translate into an economic burden upon national healthcare systems because of frequent clinical visits, prolonged hospital stays and therapies, production of new devices and scheduling of multiple revision surgeries.20 Given the high incidence of nosocomial infections, it is crucial to find solutions to mitigate this problem. Current systemic therapies, which rely on antibiotics, often lack suitable efficacy due to the increasing number of multi- and pan-resistant bacterial strains, rendering these drugs inadequate for eradicating bacteria in the biofilm mode of growth.21,22
Therefore, several research efforts have been made throughout the years to develop innovative engineered antimicrobial biomaterials.23–26 These efforts have focused particularly on strategies that allow prevention and treatment of biofilms, aiming to exert their action at the initial interface between bacteria and medical devices. Surface functionalization can be a useful tool to develop biomaterials with a tailored antibacterial action, ranging from antifouling and quorum quenching to bactericidal and biofilm disruptive surfaces.15,27 More interestingly, it is possible to design such antibacterial coatings to regulate their action in a controlled and targeted manner.
In this review, we provide an overview of strategies for fighting the infection onset through the recent design and manufacturing of engineered antibacterial surface coatings. Specifically, we present three promising surface functionalization techniques, offering description and outlining their general antibacterial applications before going into the details of their potential in achieving micro- and nano-structured self-defensive antibacterial coatings. In the final section, the potential improvements for the design and clinical validation of these smart and on-demand antimicrobial approaches are hypothesized upon, and their powerful innovative drive in the fight against antimicrobial-resistant bacterial infections is stressed (Fig. S1†).
Considerations and requirements for the design of antibacterial surface coatings
Process of bacterial adhesion and strategies for their disruption
Bacterial adhesion is a three-staged process, including (1) bacterial transport towards a surface, (2) reversible adhesion, and (3) the transition from reversible to irreversible adhesion, that is governed by both physical and chemical interactions.28 Specifically, the bacteria transport towards the solid substrate surface relies on the bacterial species as well as various environmental factors, where non-motile bacteria are influenced by gravitational and hydrodynamic forces, and Brownian motion, while motile bacteria are capable to navigate on an imperfect circular path close to the surface to facilitate their adhesion.29 After their initial contact with the surface, bacteria undergo unstable and reversible adhesion. Particularly, Lifshitz-van der Waals interactions and electrostatic forces are responsible of the bacteria adhesion on the substrate surface under static culture conditions, characterized by absence of turbulent or laminar flow. Reversible adhesion typically occurs when bacteria reach the separation distance corresponding to the secondary minimum energy. Overcoming the energy barrier allows bacteria to approach the substrate closely and form irreversible adhesion. Additionally, acid–base interactions have been studied to affect the bacterial adhesion phenomena.30
The final stage, transitioning from reversible to irreversible adhesion, occurs through molecular-level interactions between bacterial surface structures and substratum surface components. These components include extracellular polymeric substances (EPS), lipopolysaccharides, fimbriae, pili, and slime.31 As example, protein corneodesmosin (CDSN) has been identified as ligand for S. aureus and Towell et al. demonstrated that blocking the N terminus of CDSN is able to reduce the bacteria interaction with the corneocyte surface.32 Throughout these stages of bacterial adhesion, surface properties play a pivotal role. Understanding the intricate relationship between bacterial adhesion and surface properties forms the foundation for designing surfaces with anti-adhesion properties against bacteria.
Among strategies aimed at bacterial eradication, targeting the cytoplasmic membrane is crucial due to their limited ability to self-renew after damage. Consequently, antibacterial agents can bind to the cell membrane, followed by hydrophobic insertion into lipid tails, resulting in its lysis.33 Various models explain this mechanism to eradicate adhered bacteria. As example, the “Carpet” model suggests antimicrobial agents disrupt the bacterial membrane's phospholipid structure at multiple sites, creating irreparable holes, while the “Toroidal-pore” model proposes antibacterial molecules insert spirally into the membrane, forming circular holes. Lastly, the “Barrel” model suggests antibacterial molecules assemble into spiral structures within the membrane, creating pores34 (Fig. 1).
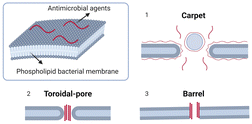 |
| Fig. 1 Three popular models to explain bacterial membrane permeabilization, (1) high accumulation of antimicrobial agent parallel to the membrane leads to its disintegration and formation of micelles, (2) lipid monolayers are forced to bend from top to bottom forming a toroidal pore, (3) antimicrobial agents completely insert themselves into the membrane forming a channel. | |
Another mechanism to eradicate bacteria involves targeting the peptidoglycans, that provide bacterial cell wall support. Indeed. targeting lipid II, a precursor for peptidoglycan synthesis, is a common strategy.35 For example, a compound synthesized from D-glucal, resembling fragments found in the recycling process of bacterial cell walls, effectively inhibits the growth of S. aureus walls. This discovery suggests the potential of developing novel antibiotics that function by halting cell wall growth.36 To note, Gram-negative bacteria possess an additional outer layer that serves as a barrier to large hydrophobic molecules. As a result, many antimicrobial agents typically exhibit lower potency against Gram-negative bacteria compared to Gram-positive ones.
Finally, innovative synthesized biocompounds have been proved in their ability (1) to enter cells and target intracellular bacteria processes, e.g., by inducing strong ribosomal inhibition in E. coli37 and (2) to modulate the in vivo immune response by reducing IL-10 levels but increasing TNF-α and INF-γ levels, considered representative immune cytokines and associated with high antibacterial activity.38
Defence mechanisms to adopt in the design of antimicrobial coatings
Since bacterial adhesion, proliferation and further colonization occur at the interface between microorganisms and medical devices, antimicrobial surface functionalization of biomaterials represents a valid approach to face the issue. There are several ways to fight the onset of an infection, acting at different stages of surface colonization and with different mechanisms, three of them are shown in Fig. 2 while Table 1 reports the applications of such defence mechanisms in literature.
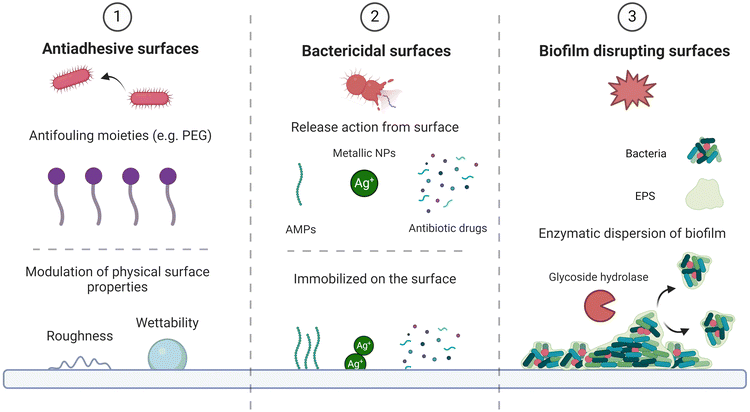 |
| Fig. 2 Scheme summarizing the three conventional defence mechanisms adopted in the design of (1) antiadhesive surfaces, exploiting physical and chemical modifications, (2) bactericidal surfaces and (3) biofilm disrupting antimicrobial coatings, that exploit immobilization or release of active molecules. The strategies and/or molecules mentioned are a few examples of the several options available in literature. | |
Table 1 Summary of published works exploiting the three defence mechanisms for the development of antibacterial solutions
Active molecules |
Substrates |
Bacterial/fungus strain |
Application & Outcome |
Ref. |
Antiadhesive surfaces |
Polymethylmethacrylate-2-methacryloyloxyethyl phosphorylcholine (PMMA-MPC) |
Liquid silicone rubber (LSR) |
S. aureus
|
PMMA-MPC modified LSR had a very high resistance against S. aureus adherence and biofilm formation. Interesting for cardiovascular implants applications. |
63
|
S. epidermidis
|
R89 rhamnolipid |
Medical-grade silicone |
S. aureus
|
R89 has proven to be a good biosurfactant for implantable devices, strongly inhibiting biofilm formation in terms of biomass and cell metabolic activity. |
64
|
S. epidermidis
|
Polysulfobetaine methacrylate (PSBMA) |
Polydimethylsiloxane (PDMS) |
E. coli
|
Beyond in vitro tests, in vivo assays on S. aureus were performed on hydrogel-coated catheters and found significant inhibition of bacterial adhesion and proliferation. |
65
|
S. aureus
|
Poly(sulfobetaine methacrylate) (pSBMA) |
PDMS |
Protein adsorption and fibroblast adhesion assays |
Durable hydrogels with strong resistance to proteins and fibroblast adhesion have been optimized, representing an interesting coating for implantable materials to contain the foreign body response. |
66
|
Poly(carboxybetaine methacrylate) (pCBMA) |
Bactericidal surfaces |
Cefuroxime sodium salt |
Polyether ether ketone (PEEK) coated with nano hydroxyapatite |
S. aureus
|
Sonocoated nanoHAP layers were loaded with drugs, the release kinetics granted good antimicrobial activity up to 24 h. Structure and cristallinity of nanoHAP were not affected, suitable for bone implants. |
67
|
Chlorhexidine |
Removable partial dentures |
S. mutans
|
A single application of the slow-releasing dosage coating on partial dentures effectively restrained S. Mutans levels and reduced plaque score for a minimum of 1 week. |
68
|
Octenidine-dihydrochloride (OCT) |
Polymeric tracheotomy tubes |
S. aureus
|
OCT coated tubes had good initial antimicrobial action and reduced superficial biofilm formation, but the effect rapidly decreased after reprocessing of the tubes because of poor adhesion properties. |
69
|
P. aeruginosa
|
Synthetic AMP (comprising KRWWKWWRR) |
PDMS |
E. coli
|
AMPs-impregnated PCL coatings achieved optimal release kinetics and did not support cells and biofilm growth up to 7 days. In vitro and in vivo studies on coated urinary catheters showed good antibacterial performance. |
70
|
Silicone Foley catheters |
S. aureus
|
P. aeruginosa
|
(9-amino-acid) cationic peptide 1037 |
Polypropylene plates |
P. aeruginosa
|
Flagellum-dependent swimming motility was reduced, bacterial swarming was inhibited, twitching motility was stimulated. The result was a reduction in biofilm formation, its combination with a second powerful antimicrobial agent could be a good therapeutic strategy. |
71
|
B. cenocepacia
|
L. monocytogenes 568
|
Silver Nanoparticles (AgNPs) |
Medical-grade silicone elastomers |
C. auris 0390
|
Potent in vitro fungicidal effect of AgNPs tested against C. auris biofilms on medical and environmental surfaces. The inhibitory action was efficient on both biofilm formation and preformed biofilm and durable in time even after several washings with PBS. |
72
|
Elastic bendage dressings |
Gold NPs biofabricated with C. Annicus aqueous extract (AuNPs-CA) |
Glass coverslips |
P. aeruginosa PAO1
|
Great antibiofilm and quorum sensing inhibition potential was observed, by dose-dependent reduction of QS-mediated virulence factors, biofilm formation and exopolysaccharide production. |
73
|
S. marcescens MTCC 97
|
Magnetic Fe3O4@PEG-Ag nanocomposites |
Polystyrene plates |
C. albicans
|
Promising magnetic field-guided smart drug delivery system and antibiotic agent with insignificant toxicity, its efficacy was comparable to commercial drugs and could be further combined with antibiotics that can form a shell on its structure. |
74
|
Petri dishes |
S. aureus
|
E. coli
|
L. major
|
AgNPs |
Silicone elastomers (PDMS) |
S. aureus
|
Great antimicrobial activity against S. aureus and good also against E. coli and P. aeruginosa, the effect depended on the particle size and NPs retained good cytocompatibility below 10 mM of silver. The synthesis allowed simultaneous reduction of silver and PDMS surface grafting. |
53
|
E. coli
|
P. aeruginosa
|
Synthetic halogenated furanone compound (furanone 56) |
Flow-cell based P. Aeruginosa biofilms |
P. aeruginosa
|
Interference with AHL-mediated quorum sensing by repressing expression on target genes of the las quorum sensing circuit and reducing production of virulence factors. It affected the architecture of biofilm and promoted the bacterial detachment process. Promising for novel non-antibiotic anti-pathogenic agents. |
75
|
Biofilm disrupting surfaces |
Streptococcal-specific bacteriophage-encoded endolysin (PlyC) |
Polystyrene plates |
Group A streptococcal (GAS) strain D471 |
PlyC, a cell wall hydrolase, directly lysed GAS cells as it diffused within the biofilm matrix acting on more than one bond. |
76
|
DNase-mimetic artificial enzyme |
S. Aureus biofilms |
S. aureus
|
In the first hour of incubation bacterial attachment was found to be less than 10%, followed by low biofilm formation after 120 h thanks to the artificial enzyme better stability. Against formed biofilms of ages 12 to 120 h, artificial DNase showed high disruption efficiency, better penetration and reusability compared to natural DNase. |
54
|
Engineered quorum-quenching lactonase (GKL) |
A. Baumannii biofilms |
A. baumannii
|
Biofilm formation was significantly reduced by the engineered mutant enzyme in thickness, biomass and surface area. Good functionalization strategy for catheters or implants to yield a new generation of bioactive biomaterials. |
56
|
5 lytic bacteriophages (vB_PaeM_USP_1, vB_PaeM_USP_2, vB_PaeM_USP_3, vB_PaeM_USP_18, and vB_PaeM_USP_25) |
Polystyrene plates and circular endotracheal tube specimens |
15 strains of P. aeruginosa (including multidrug-resistant ATCC 2108, ATCC 2110, ATCC 2112, and ATCC 2113) |
Remarkable mature biofilm reduction through biofilm disruption and production of cell debris. The antibiofilm action could be attributed to deep penetration within the biofilm thanks to the degradation of the polysaccharide matrix. The lytic effect was significant in biofilm control on infected endotracheal tubes. |
77
|
Specifically, the first one is to prevent bacterial adhesion on the surface through non-adhesive coatings, that are predominantly based on antifouling moieties.39–41 However, this mechanism can also exploit the modulation of the surface physical properties,42–47 such as wettability or roughness, as shown by Encinas et al. that reported the antiadhesive potential of micrometer and sub-micrometer sized patterned surfaces due to deformation stresses within the bacteria and also to piercing of their cell membranes by these superficial nano- and micro-structures, as long as their length scales fall just below the size of bacterial cells. Furthermore, once bacteria have adhered on the surface, antimicrobial coatings containing antibacterial and antibiofilm agents can still threaten microorganisms survival by interfering with their metabolism, physical integrity, and quorum sensing,48 thus hindering their proliferation and biofilm formation, while a third possibility relies on disrupting biofilm at its early stages.49 The second and third defence mechanism are usually achieved through integration of active molecules within the coating, such as antibiotics,50 antimicrobial peptides,51 metallic nanoparticles,52,53 quorum sensing blockers54–56 or degradative enzymes,57,58 where the biocidal components could either be immobilized on the surface or a specific leaching action could be designed for the coating.27,49 Advances in research have proven the benefits of combining these three defence methods in effectively fighting bacterial infections located in complex physiological environments.27,59–62
Responsive self-defensive antibacterial nanocoatings
Regardless of the specific strategy employed, contemporary trends in antimicrobial biomaterials emphasize the novelty of smart self-defensive coatings capable of reacting to specific changes in the surrounding microenvironment triggered by bacterial colonization.78
More generally, triggers for smart antimicrobial coatings can be (1) external, such as temperature,79 electromagnetic field,80 exposition to specific wavelengths of light54,61,81 or to ultrasound,82 and (2) internal, including changes of pH or presence of specific biological molecules/ions.79,83,84 When the stimulation originates internally and is associated with the presence of adhering and proliferating bacteria, it leads to an on-demand, targeted antibacterial response initiated solely from the coating. This response should occur only when a bacterial infection is actively developing, and the surface of the biomaterial is indeed colonized by microorganisms.85 As a result, this triggered response enables a sustained retention of efficacy over time, preventing premature loss of activity. Moreover, it enhances the biosafety of the coating by minimizing secondary negative effects on adjacent healthy cells and reducing the risk of developing multidrug-resistant bacterial strains.86,87 These coatings are commonly known as self-defensive coatings because they are engineered to activate their antibacterial properties when threatened by the onset and proliferation of bacterial colonies. Particularly advantageous is their responsiveness to local pH variations or the presence of virulence factors.88 During their metabolism, several strains of bacteria for example secrete organic acids (e.g., lactic acid by S. aureus, acetic acid by E. coli) that produce a local acidification of pH. Additionally, bacteria utilize various cellular structures, molecules, and regulatory systems known as virulence factors, which aid in their colonization of the host at the cellular level.89,90 Among the various biomolecules released, degradative enzymes can be utilized to trigger a response and subsequent activation of antibacterial potency in self-defensive coatings. This mechanism can potentially result in the delamination of the coating, consequently removing attached bacteria and early-stage biofilm (a process known as self-polishing).88
Therefore, smart design and manufacturing of micro- and nano-structured coatings on the surfaces of medical devices are crucial for achieving these responsive mechanisms. This is accomplished by fine-tuning the physical and chemical properties of the coating, such as maximizing the surface-to-volume ratio and exploring various options for grafting bioactive moieties.85,91
In the next sections of this review, we will discuss the current applications of three techniques to develop responsive self-defensive antibacterial coatings: Layer-by-Layer (LbL) self-assembly, hierarchical polymer brushes and mussel-inspired adhesive coatings (Fig. 3).
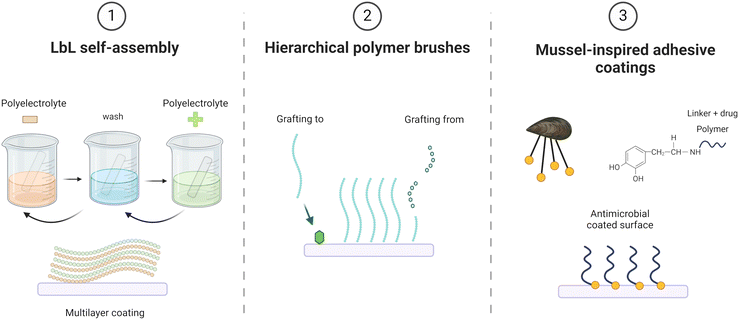 |
| Fig. 3 Schematic representation of suitable coating techniques to achieve responsive self-defensive antibacterial coatings. (1) LbL electrostatic self-assembled nanocoatings are achieved through alternate deposition of oppositely charged polyelectrolytes, (2) Hierarchical polymer brushes can either be polymerized directly on the surface thanks to an initiator (graft from) or polymeric chains can later be grafted to the surface via a linker (graft to), (3) Polydopamine-based coatings can adhere to several surfaces via their cathecol groups while their amino groups can be exploited to bind an antibacterial polymer or a linker-drug complex. | |
All these coating technologies incorporate bioinspired features, whether through their highly organized architecture (polymer brushes), akin to the multifunctional and adaptable surfaces found in biological materials, or through the natural inspiration behind their building blocks (biomimetic mussel adhesive proteins) and their bottom-up construction methods (LbL self-assembled nanoscale systems).
Layer-by-layer (LbL) nanostructured coatings
Overview of the technology and its antibacterial applications.
Layer-by-layer assembly of complementary building blocks is a particularly versatile bottom-up approach for the fabrication of nanostructured coatings. These coatings can be manufactured on any type of substrate composition and geometry, by alternating polyelectrolyte layers, which are bound together through specific interactions. Examples of possible driving forces for assembly include electrostatic attraction, hydrogen bonding, hydrophobic interactions, covalent bonding, biologically driven assembly, depending on the specific materials used.92 The multilayered coating can be designed and assembled to target several applications, because the LbL assembly can be tuned to achieve the required thicknesses and topographies through the incorporation of bioactive compounds.93 Thus, the accurate selection of building blocks, particularly such as inorganic nanoparticles, synthetic and natural polymers or their combination as polyelectrolytes, as well as biomolecules like peptides, drugs, antioxidants, can tailor the structure and physico-chemical properties of the resulting coating.94 The deposition technology can also be chosen from a wide range of possibilities, each yielding distinct features in the final film. The assembly could be performed through traditional dipping, spinning, or spraying of polyelectrolytes solutions, but also through modern 3D printing or microfluidics systems. With this array of possibilities and technology advancements, the deposition process has become increasingly suitable for automation and, therefore, easily scalable from an industrial perspective.95,96
Layer-by-layer coatings offer a wide range of possibilities when implementing an antibacterial strategy, which can be divided into two main categories: (1) encapsulation of antimicrobial compounds and (2) intrinsic antifouling/antibacterial activity.97
Integration of active antibacterial substances (e.g., antibiotics, antimicrobial peptides (AMPs), metallic nanoparticles) during construction of LbL coatings leads to the establishment of a good reservoir and to the possibility of sustained release over time. To achieve this, therapeutics can either be complexed with polyelectrolytes or directly alternated as PEMs during the deposition process, allowing for tunability of their concentration and release profile based on the number and conformation of layers.98–100
Self-defensive antibacterial LbL coatings.
Significant advances have been made in the development of self-defensive antibacterial LbL coatings. The most investigated bacteria-related triggers are acidic pH and degradative enzymes, such as hyaluronidase (HAS) or chymotrypsin (CMS), commonly secreted by bacterial colonies.85 The integration of enzymatic substrates as LbL building blocks triggers a degradative action that leads to partial delamination of the coating, resulting in both gradual release of therapeutic cargo and detachment of previously adhered biofilm-forming bacteria along with the most superficial layers.88 Several cases have been presented in literature using hyaluronic acid (HA) as substrate for HAS. For instance, Wu et al.101 alternated two polycations, 1,2-ethanediamine (EDA)-modified polyglycerol methacrylate (PGMA) and lysozyme in a LbL coating containing HA as polyanion to coat antibiotic-loaded silica nanoparticles. The action of hyaluronidase broke down hyaluronic acid, leading to coating superficial delamination. The polycations provided both bacteriolytic (lysozyme) and membrane-disruptive (cationic EDA-modified PGMA) action against bacteria. Furthermore, coating delamination also triggered the release of amoxicillin (AMO) drug only when AMO-resistant E. coli and S. aureus bacteria were present (both in vitro and in vivo). A similar approach, without the release of antibiotics, was proposed by Yao et al.,102 in which a LbL coating was developed containing HA alternated with chitosan (CHI) initially and with polylysine (PLL) in the last layers. PLL and HA provided the coating with susceptibility to both CMS and HAS degradation, while PLL and CHI acted synergistically as bactericidal compounds. The enzymatic delamination of the coating reduced both E. coli and S. aureus adhesion, improving and prolonging the bactericidal action of PLL and CHI of the underlying exposed layers (as demonstrated by LIVE/DEAD staining up to 72 h).
Another HA-containing LbL coating was developed by Wang et al.,103 which was deposited on medical silicone substrates. The alternating polyelectrolytes were montmorillonite (MMT) and HA, both electrostatically interacting with positively charged gentamicin sulfate (GS) to yield high loading of antibiotic within the coating (MMT/HA-GS)n (Fig. 4). The progressive degradation by HAS led to a dual antibiofilm-antimicrobial action due to a combination of gradual drug release and controlled film peeling. MMT has been found to be interesting in LbL antibacterial coating applications thanks to its high loading and retention properties of antimicrobial compounds, encapsulating small molecular antibiotics and possessing low thickness combined with high specific surface area. Therefore, it is extensively featured in related literature, for example in a similar work by Xu et al.104 in which MMT was loaded with GS and alternated with PLL to be degraded by CMS.
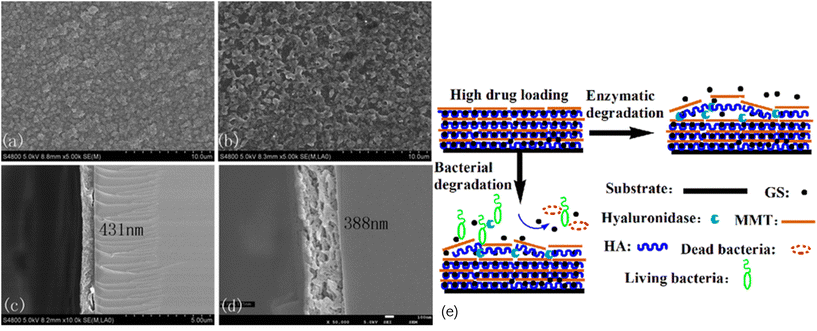 |
| Fig. 4 SEM images of surface topography and thickness before (a and c) and after (b and d) GS release for 48 h in 105 CFU mL−1 of E. coli. (e) Graphical representation of the (MMT/HA-GS)n self-assembled coating and enzymatically triggered delamination with release of GS. Reprinted with permission of B. Wang, et al., Construction of High Drug Loading and Enzymatic Degradable Multilayer Films for Self-Defense Drug Release and Long-Term Biofilm Inhibition, Biomacromolecules, 2018, 19(1), 85–93, https://doi.org/10.1021/acs.biomac.7b01268. Copyright © 2018, American Chemical Society. | |
This interesting self-polishing ability of responsive antibacterial coatings can also be triggered by a pH variation by integrating pH-cleavable linkages between layers, as some works have already investigated exploiting imine links obtained through Schiff base chemistry.105,106 Alternatively, responsiveness towards acidification of local pH can be easily achieved through integration of weak polyelectrolytes that are usually pH sensitive in terms of swelling, decomposition and/or permeability.84 The enhanced permeability of the film could either release antimicrobial agents integrated within the coating or, more simply, expose specific bioactive dominions of the molecules making up the layers.
Several research efforts in this field have been made by Prof. Sukhishvili's research team using different pH-responsive polyelectrolytes. Tannic acid, a natural polyphenol and weak polyelectrolyte (pKa = 8.5) often used in LbL antimicrobial coatings due to its intrinsic antibacterial properties,107–109 was integrated in PEMs thanks to both electrostatic interactions and hydrogen bonding with several cationic antibiotics (gentamicin, tobromycin and polymyxin B) at neutral pH. No antibiotic release was observed at neutral conditions up to 4 weeks but, upon acidification, tannic acid became strongly less ionized triggering the release of antibiotic molecules until electroneutrality was reached. Following this principle, a further decrement of pH would have again triggered antibiotic release, so to not deplete the drug all at once.110 Another interesting strategy investigated was LbL hydrogel coatings with a hydrated, open molecular structure able to host antibiotic and display antiadhesive properties. The hydrogel was composed of cross-linked poly methacrylic acid (PMAA) and loaded via electrostatic interaction with positively charged antibiotics or AMPs until complete neutralization. The pH-triggered release was fast in this case, demonstrating no diffusional constraints from the coating.111,112 Hydrogel coatings composed of poly (2-alkylacrylic) acids were also investigated for their intrinsic bactericidal properties related to their hydrophobicity. The antibacterial effect was attributed to the conformational transition from coil to globule of the polyacid chains caused by the pH drop, thus exposing hydrophobic moieties that penetrated within the bacterial membrane.113 The same researchers also exploited inorganic polyelectrolytes like MMT114 and polyphosphazenes115 for self-defensive antibacterial coating development.
Developing a coating responsive to multiple triggers in such a complex environment like the bacterial niche can be advantageous, as some have already started to investigate by developing a pH-responsive multilayer coating with an enzyme-responsive outer shell. Wang et al.116 have designed a pH-responsive multilayer composed of antibiotic (gentamicin), silver nanoparticles (Ag NPs) and tannic acid on magnetic nanoparticles. The coating was finally covered by a hyaluronic acid external layer to improve the biocompatibility of the system while also imparting responsivity towards HAS. Such magnetic nanoparticles are supposed to be selectively guided within the bacterial biofilm and respond to the bacterial infection microenvironment by releasing the antimicrobial agents on-demand.
These latest strategies represent the current desirable solutions for tackling bacterial infections because they can perform on-demand delivery of antimicrobial agents while also overpassing biofilm.
Hierarchical polymer brushes
Overview of the technology and its antibacterial applications.
Polymer brushes represent a particular coating technique able to produce very thin and well-organized polymeric films, tightly bound to solid substrates and possessing very high mechanical and chemical robustness. Additionally, this technique allows for the simple tuning of parameters such as superficial grafting density, final thickness, and polymeric chain chemistry.117 It also offers the possibility of post modifications with additional functional groups. As a result, the coating displays finely regulated interfacial properties such as wettability, surface energy, capability of molecules adsorption/binding, cell adhesion, and rheological behaviour. These properties are related to the nanoscale architecture of the generated polymeric patterns, which is typical of the native ECM of biological tissues, making them highly suitable for biomedical applications.118 The deposition process can be achieved through either a ‘grafting-to’ or a ‘grafting-from’ strategy. In the first case, previously polymerized chains are anchored to the surface via physisorption or chemisorption, while in the second the monomers start to polymerize and form chains on the substrate due to its functionalization with an initiator molecule, a process also known as surface-initiated polymerization. This method can be achieved through a multitude of controlled polymerization techniques.119 Surface-initiated atom transfer radical polymerization (SI-ATRP) is one of the most popular methods to obtain biomolecule-functionalized polymer brushes.120
Furthermore, polymer brushes can serve as a powerful tool in the development of antibacterial surfaces, and several efforts have already been made in this direction, where the main antibacterial strategies are: (1) actively biocidal brushes, (2) non-biofouling brushes and (3) their combination.121 Biocidal polymer brushes can either encompass intrinsic biocidal polymeric blocks, like poly-β-peptides,122 quaternized polymers,123,124 polyguanidines,125,126 cationic127 and fluorinated polymers128 or be used as reservoirs of antimicrobial agents.129,130 Another successful antibacterial strategy is the inhibition of bacterial adhesion and biofilm formation on the surface, exploiting the non-biofouling properties of specific brushes131–133 linked to their high hydrophilicity or zwitterionic nature, which weakens attractive forces between colonizing bacteria and substrate, thus delaying biofilm growth.134,135 The synergistic antimicrobial-antifouling action of specifically combined polymer brushes is also promising because it allows prolonging the coating's effectiveness.136
Self-defensive antibacterial polymer brush coatings.
The conformation and structure of polymer brushes can be easily tuned by a wide range of external triggers, resulting in a responsive surface that offers significant potential for the development of smart antibacterial coatings. The stimuli may include e.g. temperature,137 pH,138 type of solvent,139 and the presence of ions.140
Considering in particular pH variations, the brushes composed of charged groups in their repeating units are defined as polyelectrolyte brushes. When subjected to a specific pH, weak polyelectrolyte brushes undergo changes in the number and density of charges, a behaviour that can result in polymeric chains swelling/collapse.141 The stimulus of pH acidification is the most investigated and exploited in self-defensive antibacterial polymer brushes. Over the last decade, several works have been published following this strategy, wherein the bactericidal activity is either related to the selected polymers or to the loading of antimicrobial agents.
Moreover, to avoid toxicity and premature depletion of the biocidal component, the active layer is typically shielded by an outer biocompatible and antifouling polymeric layer made of zwitterionic or highly hydrophilic polymers. For instance, Liu et al.142 developed a hierarchical polymer brushes system for universal polymeric substrates. They utilized a hydrophilic outer layer of poly(hydroxyethyl methacrylate) (pHEMA) to hinder bacterial adhesion at neutral pH. Melittin (MLT), a cationic AMP, was electrostatically adsorbed onto an inner anionic layer of poly(2,3-dimethylmaleic anhydride) (PDMMA). The amide bonds of PDMMA were cleaved upon bacterial acidification of local pH, triggering a charge-conversion (from negative to positive) release mechanism of MLT. Similarly, Yan et al.143 utilized a pH-responsive hydrophilic outer layer composed of poly(methacrylic acid) (PMAA) combined with a different AMP (cecropin B) covalently bound to an inner poly(2-vinyl 4,4-dimethyl azlactone) (PVDMA) layer. In this case, the hydrated PMAA chains collapsed at lower pH values due to their increasing hydrophobic nature, thereby exposing the AMPs bound to the inner layer. This allowed a smart reversible switch between antifouling and bactericidal coating behaviour over time, without the need of additional reloading of antibacterial agent, making it potentially suitable for reusable surgical devices (Fig. 5). Another feasible solution for pH-responsive polymer brushes is the incorporation of acid-labile Schiff base linkages within the coating. Jin et al.144 implemented a hierarchical polymer coating for infected bone defect therapy, made of ethanediamine-functionalized poly(glycidyl methacrylate) (PGED) brushes conjugated with the antibiotic GS though Schiff base bonds. These bonds were reduced in acidic pH but remained completely stable in a neutral environment. PGED exhibited very low cytotoxicity, in agreement with in vivo applications, and the release of GS was a self-adaptive response, allowing for a sustainable action over time, as demonstrated by recycling antibacterial assays. Similarly, Zhang et al.145 developed a switchable antifouling/bactericidal polymer brush coating exploiting the cleavage of Schiff base bonds at low pH. The strategy involved a dual layer polymer brush for catheter biomaterials (e.g., polyurethanes), with the inner layer comprising bactericidal quaternary ammonium poly[2-(dimethyl decyl ammonium)ethyl methacrylate] (PQDMAEMA) and the outer layer made of antifouling polyethylene glycol (PEG). While quaternary ammonium polymer brushes are effective antibacterial agents, they have intrinsic cytotoxicity and are prone to fouling due to their positive charge, necessitating coverage with an antifouling and biocompatible layer.
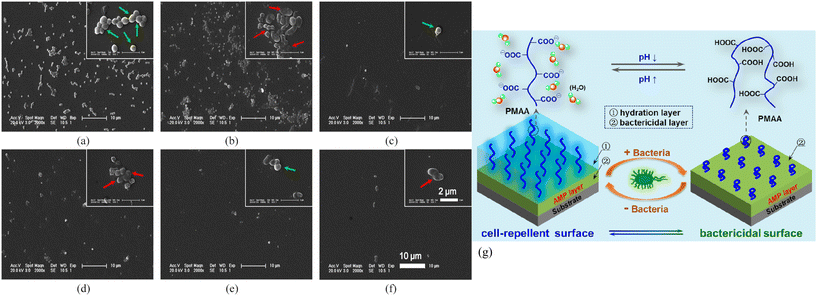 |
| Fig. 5
S. Aureus attachment (106 cells per ml for 24 h) for (a) unmodified Si (b) AMP-grafted Si (c) one-layer PMAA (d) AMP-grafted one-layer P(VDMA-co-MAA) (e) AMP-grafted hierarchical PVDMA-b-PHEMA (f) AMP-grafted hierarchical PVDMA-b-PMAA. Red arrows indicate damaged bacteria cells. (g) Schematic representation of the reversible nature of the coating. Reprinted with permission of S. Yan, et al., Nonleaching Bacteria-Responsive Antibacterial Surface Based on a Unique Hierarchical Architecture, ACS Appl. Mater. Interfaces, 2016, 8(37), 24471–24481, https://doi.org/10.1021/acsami.6b08436. Copyright © 2016, American Chemical Society. | |
Using the same type of biocidal polymers, Sun et al.146 have reported an interesting approach for polymeric implants surfaces (e.g., polypropylene meshes) that exploited a different bacteria-related trigger. They converted poly(2-dimethyl amino) ethyl methacrylate (PDMAEMA) brushes into a zwitterionic structure through quaternization and phosphorylation reactions, and then covered it with an upper layer of zwitterionic poly(sulfobetaine methacrylate) (PSBMA), providing non-adhesive and biocompatible properties. Bacterial phosphatase, one of the key enzymatic virulence factors produced during bacterial colonization and biofilm formation, cleaved phosphatase side groups present on PDMAEMA chains, exposing bactericidal polycations. During the bacteria killing process, PSBMA synergistically prevented the attachment of dead bacteria and shielded the cytotoxic polycations.
Mussel-inspired adhesive coatings
Overview of the technology and its antibacterial applications.
Mussel adhesive proteins have proven to offer a simple and versatile approach for biomaterials functionalization, leading to the development of conformal thin polydopamine (PDA) coatings. Their adhesive nature draws inspiration from the way in which mussels securely attach to surfaces underwater, achieved through a combination of catechol (3,4-dihydroxybenzene) and amino groups (primary and secondary) present in their foot proteins. Catechols are attributed to dopamine (DOPA), while ammines to lysine (Lys) and histidine residues.147 This adhesive strength is derived from strong intramolecular interactions formed at the substrate interface, including hydrogen bonding, π–π stacking, electrostatic interactions, catechol-metal coordination, and covalent reactions. It stands out as one of the first single-step, material-independent surface chemistries that have found immense success in surface modifications across several engineering applications, owing to its high long-term stability, mild reaction conditions, low cost and substrate adaptability.148
The most basic coating approach requires no pre-functionalization steps and simply relies on the immersion of the sample to be coated in a basic solution of DOPA, followed by spontaneous polymerization of PDA on the surface in an appropriate reaction time. The resulting coating can be used as-is or further functionalized, serving as a primer coating for the grafting of several biomolecules and/or polymers to achieve multifunctional surfaces.149 In biomedical applications, PDA coatings exhibit low cytotoxicity and excellent biocompatibility, making them powerful tools for modifying biomaterials. Among these applications, antibacterial strategies have gained popularity, owing to both intrinsic antimicrobial properties of PDA coatings and the potential to develop PDA-based composites with enhanced antibacterial capabilities.150
Stand-alone PDA exhibits surface-contact bacterial lysis action due to the high content of Lys residues in mussel foot protein-5 (Mpf-5), along with great oxidative killing capacity thanks to H2O2 production.151–153 Furthermore, PDA coatings offer notable bactericidal potential through photothermal therapy.154,155 The synergistic activity of PDA with other antibacterial agents has also been widely investigated, leading to the development of PDA-assisted co-deposition of layers with outstanding multifunctional antimicrobial performance. Particularly, the combination with metallic ions, cationic and zwitterionic polymers has enabled the implementation of anti-adhesive coatings with bactericidal potential.156–159
Self-defensive antibacterial mussel-inspired coatings.
The integration of a bacteria-responsive feature in antimicrobial mussel-inspired coatings represents a very significant innovation towards substrate-independent, simple and smart antibacterial strategies. Some recent works have already been published, exploiting dynamic covalent chemistry to impart responsive features and PDA-like polymers to grant simplicity and substrate adaptability. Yang et al.160 conducted research on mussel-inspired oxidative polymerization of 5,6-dihydroxyindole (DHI), a typical monomer of melanins, which presented catechol groups to ensure the formation of a stable adhesive first layer on the surface. Then, sequential layers of formylphenylboronic acid linkers (FPBA) and aminoglycosides antibiotics (AGs) were deposited through boronate-catechol complexation and Schiff base reaction. The resulting bonds, boronate esters and imines, were both reversible and pH-sensitive, capable of being cleaved in acidic microenvironment during bacterial infections, thereby achieving triggered on-demand AGs release. The technology is completely substrate-independent, and the authors have tested it on both polymeric and ceramic surfaces. Concurrently, the same group161 presented another coating approach (Fig. 6) that utilized solely natural molecules and reversible pH-sensitive bonds, deposited on organic, inorganic and metallic substrates (e.g. polyethersulfone PES, polyvinyl chloride PVC catheters and titanium bone nails). The two main constituents, AGs (e.g tobramycin TOB) and protocatechualdehyde (PA), both exhibited antibacterial action. PA are polyphenolic molecules with aldehyde functionalities that provide a polycatechol structure with strong affinity and adhesion to several surfaces through mussel-inspired enzymatic polymerization catalyzed by horseradish peroxidase (HRP) in presence of H2O2. The investigated process was a one-pot reaction that also involved the formation of multiple dynamic imine bonds between the pendant aldehydes of PA and the amino groups of AGs via Schiff base reaction. The coating degradation and thus release profile of TOB could be tuned by addition of cathecol (CAT) monomer for copolymerization. Moreover, the coating could easily be cleaned without altering the original surface properties and substrates could be coated again. It is a strategy that requires very mild conditions, low-cost natural materials, and simple instrumentation setups, while providing the coating with a smart antimicrobial performance.
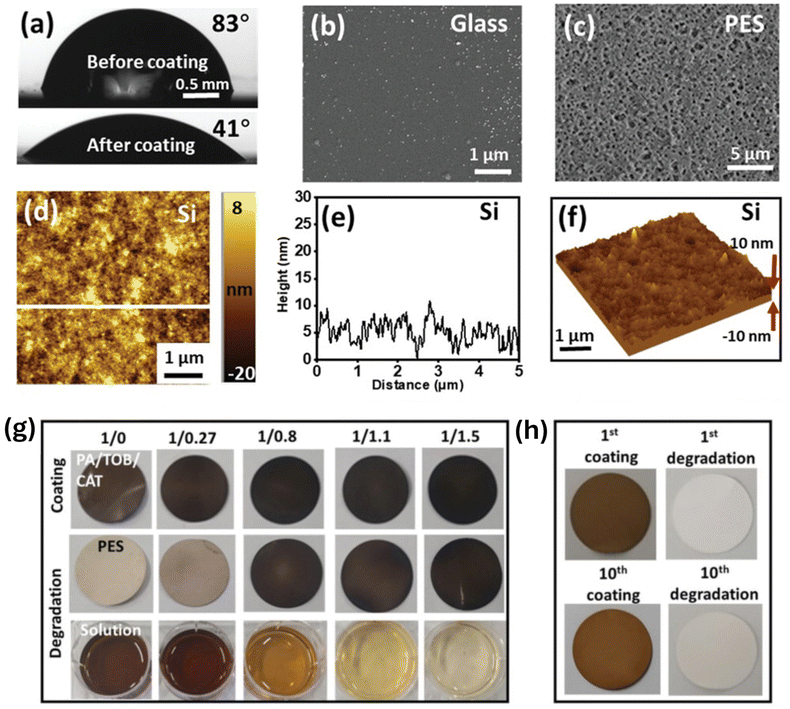 |
| Fig. 6 (a) Water contact angle of glass w/(top) and w/o (bottom) PA/TOB coating (b and c) SEM images of PA/TOB coated glass and PES (d) AFM image of PA/TOB coated Si (e) coating height fluctuation derived from AFM images (f) 3D AFM coated surface reconstruction (g) coating appearance and degradation for different PA/CAT ratios (h) recyclability of the antibacterial coating. Reprinted with permission of L. Yang, et al., Bioinspired Integration of Naturally Occurring Molecules towards Universal and Smart Antibacterial Coatings, Adv Funct Mater, 2022, 32(4), 1–10, https://doi.org/10.1002/adfm.202108749. Copyright © 2021, Wiley-VCH GmbH. | |
Interestingly, such dynamic covalent bonds can be exploited also for a self-cleaning strategy, rather than self-defensive. Asha et al.162 developed a smart antibacterial and antifouling coating with dual responsiveness. The primer adhesive layer was composed of PDA, onto which a zwitterionic and a cationic polymer were covalently grafted. Specifically synthesized for the application, 2-methacryloyloxyethyl phosphorylcholine (MPC) copolymerized with 5-methacrylamido-1,2-benzoxaborole (MAABO) resulted in the bioinspired zwitterionic (poly(MPC-st-MAABO)), chosen to impart antifouling properties, while the copolymer of quaternary ammonium cationic poly(2-(methacryloyloxy)ethyl trimethylammonium) and MAABO (poly(META-st-MAABO)) exerted bactericidal action. The benzoxaborole pendant groups contained in the polymers could be complexed with catechols, forming reversible covalent boronate ester bonds, responsive to both pH and sugars.163 In this case, the bactericidal and antifouling activity was already solid and did not need a trigger to be activated but could greatly benefit from a cleaning step where all the dead bacteria and negatively charged proteins attached to the cationic brushes were detached from the surface. The self-cleaning action was related to dissociation of the boronate ester bonds and could be triggered either by bacteria-related acidification of pH or by addition of a competitive molecule containing cis-diols, so for example after administration of sugars/saccharides solutions. The coating had also regeneration ability if subjected to freshly prepared poly(MPC-st-MAABO) and poly(META-st-MAABO) solutions at physiological pH, thanks to a high affinity between benzoxaborole groups and cis-diols of the remaining PDA layer, demonstrating capacity for sustained long-term antibacterial performances.
Conclusions and future perspectives
The advancement of on-demand responsive antibacterial coatings stands out as a compelling frontier in the ongoing battle against microbial pathogens that holds the potential to revolutionize infection control strategies. Moving forward, the logical step appears to be a multifaceted approach that integrates various triggers for antibacterial activity within a single coating, as some of the previously cited works have already started to investigate, in favour of broadening the applicability of such coatings towards a multitude of different microorganisms, each one with specific pathogenic mechanisms that do not necessarily involve all the triggers discussed or not in equal measure. Thus, the strategic line of action to enhance the coating efficacy in eradicating bacterial colonization comes across as exerting a synergistic antibacterial action evoked by pH fluctuations, secretion of virulence factors by bacteria but also of reactive oxygen species (ROS) by proinflammatory immune cells in the infected tissue.164–166 On this note, the complex case of polymicrobial infections that involve different bacterial strains could also be tackled by such a comprehensive approach.
Another significant improvement worthy of investigation would be the integration of active targeting mechanisms within the coating, so to refine the specificity of its action. Indeed, by selectively targeting bacterial cells while sparing healthy host tissues, these coatings have the potential to minimize off-target effects and mitigate the risk of collateral damage. This targeted approach not only enhances the efficacy of antibacterial treatments but also holds profound implications for the pressing global health concern of reducing the emergence of antibiotic-resistant strains. One possibility is offered by microbial lectins,167 which are proteins expressed on bacterial membranes and biofilms to bind with host glycans, and their high affinity towards sugars and specifically multivalent polysaccharides.168,169 The interaction between lectins and glycan-mimicking ligands within the coating could secure the immobilization of the microorganisms and promote the action of the antibacterial agents, as was proved in this work by Ye et al.170 in which an active targeting mechanism based on dextran-lectin interactions enabled greater bioavailability of pH- and ROS-responsive nanoparticles containing antibiotic (rifampicin) and a cationic polymer while also promoting their selective internalization to also target intracellular infections.
Besides the implementation of potential improvements, another pivotal aspect to be addressed is how to facilitate the technological transfer of these coatings on commercially available medical devices. The translation of these advancements from the laboratory bench to clinical practice necessitates rigorous validation platforms that closely mimic the complex infection microenvironment.171 Incorporating co-cultures of bacteria and cells into validation studies could enable researchers to assess the actual efficacy, biocompatibility, and safety profiles of these coatings under conditions that more accurately replicate real-world scenarios. Such validation efforts are paramount for ensuring the reliability and effectiveness of these coatings in clinical settings, where the infection control and patient care are highly important.
Finally, the development and refinement of on-demand responsive antibacterial coatings represent a crucial step towards addressing the challenges posed by antimicrobial resistance and infectious diseases. Through interdisciplinary collaboration and innovative design strategies, these coatings offer a promising avenue for advancing infection control measures and improving patient outcomes in healthcare settings. By harnessing the power of targeted antibacterial action and adapting to the dynamic nature of microbial infections, these coatings could herald a new era in the fight against antibiotic-resistant pathogens, bringing in a future where effective infection control is within reach.
Author contributions
Conceptualization – M. A. Cassa, I. Carmagnola; writing (original draft) – M. A. Cassa, P. Gentile, J. Girón-Hernández; writing (review and editing) – M. A. Cassa, I. Carmagnola, G. Ciardelli, P. Gentile, J. Girón-Hernández; supervision – G. Ciardelli.
Data availability
No primary research results, software or code have been included and no new data were generated or analysed as part of this review.
Conflicts of interest
There are no conflicts to declare.
Acknowledgements
Schematic figures were created with BioRender.com.
M.A. Cassa would like to thank the EU Horizon 2020 ALTERNATIVE project [grant no. 101037090] for co-funding her PhD scholarship.
References
- K. I. Okuda, R. Nagahori, S. Yamada, S. Sugimoto, C. Sato, M. Sato, T. Iwase, K. Hashimoto and Y. Mizunoe, Front. Microbiol., 2018, 9, 182 CrossRef PubMed.
- H. Elgharably, S. T. Hussain, N. K. Shrestha, E. H. Blackstone and G. B. Pettersson, Semin. Thorac. Cardiovasc. Surg., 2016, 28, 56–59 CrossRef PubMed.
- P. Y. Litzler, L. Benard, N. Barbier-Frebourg, S. Vilain, T. Jouenne, E. Beucher, C. Bunel, J. F. Lemeland and J. P. Bessou, J. Thorac. Cardiovasc. Surg., 2007, 134, 1025–1032 CrossRef PubMed.
- N. Abdullah, O. S. Erdmann and B. E. Borges, Res. Soc. Dev., 2021, 10, 16 Search PubMed.
- G. Rezende-Pereira, J. P. Albuquerque, M. C. Souza, B. A. Nogueira, M. G. Silva, R. Hirata, A. L. Mattos-Guaraldi, R. S. Duarte and F. P. G. Neves, Aesthetic Surg. J., 2021, 41, 1144–1151 CrossRef PubMed.
- J. Spałek, P. Deptuła, M. Cieśluk, A. Strzelecka, D. Łysik, J. Mystkowska, T. Daniluk, G. Król, S. Góźdź, R. Bucki, B. Durnaś and S. Okła, Pathogens, 2020, 9, 1–17 CrossRef PubMed.
- A. Kumar, M. K. Seenivasan and A. Inbarajan, Cureus, 2021, 13, 11 Search PubMed.
-
S. Hahnel, in Biofilms and Implantable Medical Devices, ed. Y. Deng and W. Lv, Woodhead Publishing, 1st edn, 2017, part 2, ch. 5, pp. 117–140 Search PubMed.
- S. J. McConoughey, R. Howlin, J. F. Granger, M. M. Manring, J. H. Calhoun, M. Shirtliff, S. Kathju and P. Stoodley, Future Microbiol., 2014, 9, 987–1007 CrossRef CAS PubMed.
- N. Ielapi, E. Nicoletti, C. Lorè, G. Guasticchi, T. Avenoso, A. Barbetta, S. de Franciscis, M. Andreucci, P. Sapienza and R. Serra, Rev. Recent Clin. Trials, 2020, 15, 22–27 Search PubMed.
- D. J. Stickler, J. Intern. Med., 2014, 276, 120–129 CrossRef CAS PubMed.
- S. Baidya, S. Sharma, S. K. Mishra, H. P. Kattel, K. Parajuli and J. B. Sherchand, BioMed Res. Int., 2021, 8817700 CAS.
- S. Kackar, E. Suman and M. S. Kotian, Indian J. Med. Microbiol., 2017, 35, 80–84 CrossRef PubMed.
- E. Adler, D. Miller, O. Rock, O. Spierer and R. Forster, Br. J. Ophthalmol., 2018, 102, 1602–1606 CrossRef PubMed.
- Y. Su, J. T. Yrastorza, M. Matis, J. Cusick, S. Zhao, G. Wang and J. Xie, Adv. Sci., 2022, 9, 29 Search PubMed.
- Z. Khatoon, C. D. McTiernan, E. J. Suuronen, T.-F. Mah and E. I. Alarcon, Heliyon, 2018, 4, e01067 CrossRef PubMed.
-
K. A. Floyd, A. R. Eberly and M. Hadjifrangiskou, in Biofilms and Implantable devices, ed. Y. Deng and L. Wei, Woodhead Publishing, 1st edn, 2017, part 1, ch. 3, pp. 47–95 Search PubMed.
- R. B. McFee, Disease-a-Month, 2009, 55, 422–438 CrossRef PubMed.
- M. H. Kollef, A. Torres, A. F. Shorr, I. Martin-Loeches and S. T. Micek, Crit. Care Med., 2021, 49, 169–187 CrossRef PubMed.
- M. Cámara, W. Green, C. E. MacPhee, P. D. Rakowska, R. Raval, M. C. Richardson, J. Slater-Jefferies, K. Steventon and J. S. Webb, npj Biofilms Microbiomes, 2022, 8, 42 CrossRef PubMed.
- P. G. Bowler, J. Wound Care, 2018, 27, 273–277 CrossRef PubMed.
-
National Action Plans and Monitoring and Evaluation (NPM) and Antimicrobial Resistance Division (AMR), Global Action Plan on Antimicrobial Resistance, World Health Organization, 2015, ISBN: 9789241509763 Search PubMed.
- J. S. Fernandes, P. Gentile, R. A. Pires, P. V. Hatton and R. L. Reis, Acta Biomater., 2017, 59, 2–11 CrossRef CAS PubMed.
- M. A. Bonifacio, S. Cometa, A. Cochis, P. Gentile, A. M. Ferreira, B. Azzimonti, G. Procino, E. Ceci, L. Rimondini and E. De Giglio, Carbohydr. Polym., 2018, 198, 462–472 CrossRef CAS PubMed.
- J. M. Vasquez, A. Idrees, I. Carmagnola, A. Sigen, S. Mcmahon, L. Marlinghaus, G. Ciardelli, U. Greiser, H. Tai, W. Wang, J. Salber and V. Chiono, Front. Bioeng. Biotechnol., 2021, 9, 712435 Search PubMed.
- R. Laurano, V. Chiono, C. Ceresa, L. Fracchia, A. Zoso, G. Ciardelli and M. Boffito, Eng. Regen., 2021, 2, 263–278 Search PubMed.
- X. Ding, S. Duan, X. Ding, R. Liu and F. J. Xu, Adv. Funct. Mater., 2018, 28, 1–19 Search PubMed.
- K. Yang, J. Shi, L. Wang, Y. Chen, C. Liang, L. Yang and L.-N. Wang, J. Mater. Sci. Technol., 2022, 99, 82–100 CrossRef CAS.
- P. D. Frymier, R. M. Ford, H. C. Berg and P. T. Cummings, Proc. Natl. Acad. Sci. U. S. A., 1995, 92, 6195–6199 CrossRef CAS PubMed.
- H. Wang, M. Sodagari, Y. Chen, X. He, B. Z. Newby and L. K. Ju, Colloids Surf., B, 2011, 87, 415–422 CrossRef CAS PubMed.
- M. Katsikogianni, Y. F. Missirlis, L. Harris and J. Douglas, Eur. Cells Mater., 2004, 8, 37–57 CrossRef CAS PubMed.
- A. M. Towell, C. Feuillie, P. Vitry, T. M. da Costa, M. Mathelié-Guinlet, S. Kezic, O. M. Fleury, M. A. McAleer, Y. F. Dufrêne, A. D. Irvine and J. A. Geoghegan, Proc. Natl. Acad. Sci. U. S. A., 2021, 118, 1 CrossRef PubMed.
- W. Chin, C. Yang, V. W. L. Ng, Y. Huang, J. Cheng, Y. W. Tong, D. J. Coady, W. Fan, J. L. Hedrick and Y. Y. Yang, Macromolecules, 2013, 46, 8797–8807 CrossRef CAS.
- K. A. Brogden, Nat. Rev. Microbiol., 2005, 3, 238–250 CrossRef CAS PubMed.
- A. J. Wolf and D. M. Underhill, Nat. Rev. Immunol., 2018, 18, 243–254 CrossRef CAS PubMed.
- X. L. Zhang, G. Báti, C. Li, A. Guo, C. Yeo, H. Ding, K. B. Pal, Y. Xu, Y. Qiao and X. W. Liu, J. Am. Chem. Soc., 2024, 146, 7400–7407 CrossRef CAS PubMed.
- P. Koch, S. Schmitt, A. Heynisch, A. Gumpinger, I. Wüthrich, M. Gysin, D. Shcherbakov, S. N. Hobbie, S. Panke and M. Held, BMC Biol., 2022, 20, 1–21 CrossRef PubMed.
- D. Zhao, W. Feng, X. Kang, H. Li, F. Liu, W. Zheng, G. Li and X. Wang, J. Mater. Chem. B, 2023, 11, 2958–2971 RSC.
- L. Liu, W. Li and Q. Liu, Wiley Interdiscip. Rev.: Nanomed. Nanobiotechnol., 2014, 6, 599–614 CAS.
- A. M. Telford, M. James, L. Meagher and C. Neto, ACS Appl. Mater. Interfaces, 2010, 2, 2399–2408 CrossRef CAS PubMed.
- F. Song, L. Zhang, R. Chen, Q. Liu, J. Liu, J. Yu, P. Liu, J. Duan and J. Wang, ACS Appl. Mater. Interfaces, 2021, 13, 33417–33426 CrossRef CAS PubMed.
- N. Encinas, C. Y. Yang, F. Geyer, A. Kaltbeitzel, P. Baumli, J. Reinholz, V. Mailänder, H. J. Butt and D. Vollmer, ACS Appl. Mater. Interfaces, 2020, 12, 21192–21200 CrossRef CAS PubMed.
- Q. Pan, Y. Cao, W. Xue, D. Zhu and W. Liu, Langmuir, 2019, 35, 11414–11421 CrossRef CAS PubMed.
- J. K. Oh, X. Lu, Y. Min, L. Cisneros-Zevallos and M. Akbulut, ACS Appl. Mater. Interfaces, 2015, 7, 19274–19281 CrossRef CAS PubMed.
- A. H. A. Lutey, L. Gemini, L. Romoli, G. Lazzini, F. Fuso, M. Faucon and R. Kling, Sci. Rep., 2018, 8, 1–10 CAS.
- K. Doll, I. Yang, E. Fadeeva, N. Kommerein, S. P. Szafrański, G. Bei der Wieden, A. Greuling, A. Winkel, B. N. Chichkov, N. S. Stumpp and M. Stiesch, ACS Appl. Mater. Interfaces, 2019, 11, 23026–23038 CrossRef CAS PubMed.
- M. Amin, S. Rowley-Neale, L. Shalamanova, S. Lynch, J. T. Wilson-Nieuwenhuis, M. El Mohtadi, C. E. Banks and K. A. Whitehead, ACS Appl. Mater. Interfaces, 2020, 12, 21057–21069 CrossRef CAS PubMed.
- S. T. Asma, K. Imre, A. Morar, V. Herman, U. Acaroz, H. Mukhtar, D. Arslan-Acaroz, S. R. A. Shah and R. Gerlach, Life, 2022, 12, 1–31 Search PubMed.
- K. Bruellhoff, J. Fiedler, M. Möller, J. Groll and R. E. Brenner, Int. J. Artif. Organs, 2010, 33, 646–653 CrossRef CAS PubMed.
- M. Zilberman and J. J. Elsner, J. Controlled Release, 2008, 130, 202–215 CrossRef CAS PubMed.
- A. Di Somma, A. Moretta, C. Canè, A. Cirillo and A. Duilio, Biomolecules, 2020, 10, 1–15 CrossRef PubMed.
- D. de Lacerda Coriolano, J. B. de Souza, E. V. Bueno, S. M.F. R. D. S. Medeiros, I. D. L. Cavalcanti and I. M. F. Cavalcanti, Braz. J. Microbiol., 2021, 52, 267–278 CrossRef CAS PubMed.
- M. A. Velazco-medel, L. A. Camacho-cruz, H. Magaña, K. Palomino and E. Bucio, Molecules, 2021, 26, 1–16 CrossRef PubMed.
- Z. Chen, Z. Wang, J. Ren and X. Qu, Acc. Chem. Res., 2018, 51, 789–799 CrossRef CAS PubMed.
- H. Z. Asfour, J. Microsc. Ultrastruct., 2018, 6, 1–10 CrossRef PubMed.
- J. Y. Chow, Y. Yang, S. B. Tay, K. L. Chua and W. S. Yew, Antimicrob. Agents Chemother., 2014, 58, 1802–1805 CrossRef PubMed.
- J. B. Kaplan, Int. J. Artif. Organs, 2018, 32, 545–554 CrossRef PubMed.
- R. Ramakrishnan, A. K. Singh, S. Singh, D. Chakravortty and D. Das, J. Biol. Chem., 2022, 298, 9 CrossRef PubMed.
- X. Chu, F. Yang and H. Tang, Chin. J. Chem., 2022, 40, 2988–3000 CrossRef CAS.
- Y. Zou, K. Lu, Y. Lin, Y. Wu, Y. Wang, L. Li, C. Huang, Y. Zhang, J. L. Brash, H. Chen and Q. Yu, ACS Appl. Mater. Interfaces, 2021, 13, 45191–45200 CrossRef CAS PubMed.
- Y. Wang, Y. Zou, Y. Wu, T. Wei, K. Lu, L. Li, Y. Lin, Y. Wu, C. Huang, Y. Zhang, H. Chen and Q. Yu, ACS Appl. Mater. Interfaces, 2021, 13, 48403–48413 CrossRef CAS PubMed.
- T. Y. Liao, C. D. Easton, H. Thissen and W. B. Tsai, ACS Biomater. Sci. Eng., 2020, 6, 3349–3360 CrossRef CAS PubMed.
- F. Woitschach, M. Kloss, K. Schlodder, A. Rabes, C. Mörke, S. Oschatz, V. Senz, A. Borck, N. Grabow, E. C. Reisinger and M. Sombetzki, Front. Bioeng. Biotechnol., 2021, 9, 1–11 Search PubMed.
- C. Ceresa, F. Tessarolo, D. Maniglio, E. Tambone, I. Carmagnola, E. Fedeli, I. Caola, G. Nollo, V. Chiono, G. Allegrone, M. Rinaldi and L. Fracchia, Molecules, 2019, 24, 3843 CrossRef CAS PubMed.
- J. Zhang, M. Wu, P. Peng, J. Liu, J. Lu, S. Qian and J. Feng, ACS Appl. Mater. Interfaces, 2022, 14, 56097–56109 CrossRef CAS PubMed.
- B. L. Leigh, E. Cheng, L. Xu, A. Derk, M. R. Hansen and C. A. Guymon, Langmuir, 2019, 35, 1100–1110 CrossRef CAS PubMed.
- K. AbouAitah, M. Bil, E. Pietrzykowska, U. Szałaj, D. Fudala, B. Sokołowska, W. Swieszkowski, M. Lojkowski, B. Woźniak, J. Nasiłowska, W. Swieszkowski, A. Swiderska-Sroda and W. Lojkowski, Nanomaterials, 2021, 11, 1690 CrossRef CAS PubMed.
- D. Zyskind, D. Steinberg, A. Stabholz, M. Friedman and M. N. Sela, J. Oral Rehabil., 1990, 17, 61–66 CrossRef CAS PubMed.
- M. Zumtobel, O. Assadian, M. Leonhard, M. Stadler and B. Schneider, BMC Microbiol., 2009, 9, 150 CrossRef PubMed.
- J. Le Low, P. H.-N. Kao, P. A. Tambyah, G. L. E. Koh, H. Ling, K. A. Kline, W. S. Cheow and S. S. J. Leong, Biotechnol. Notes, 2021, 2, 1–10 CrossRef.
- C. De La Fuente-Núñez, V. Korolik, M. Bains, U. Nguyen, E. B. M. Breidenstein, S. Horsman, S. Lewenza, L. Burrows and R. E. W. Hancock, Antimicrob. Agents Chemother., 2012, 56, 2696–2704 CrossRef PubMed.
- H. H. Lara, L. Ixtepan-Turrent, M. Jose Yacaman and J. Lopez-Ribot, ACS Appl. Mater. Interfaces, 2020, 12, 21183–21191 CrossRef CAS PubMed.
- F. A. Qais, I. Ahmad, M. Altaf and S. H. Alotaibi, ACS Omega, 2021, 6, 16670–16682 CrossRef CAS PubMed.
- K. Zomorodian, H. Veisi, S. M. Mousavi, M. S. Ataabadi, S. Yazdanpanah, J. Bagheri, A. P. Mehr, S. Hemmati and H. Veisi, Int. J. Nanomed., 2018, 13, 3965–3973 CrossRef CAS PubMed.
- M. Hentzer, K. Riedel, T. B. Rasmussen, A. Heydorn, J. B. Andersen, M. R. Parsek, S. A. Rice, L. Eberl, S. Molin, N. Høiby, S. Kjelleberg and M. Givskov, Microbiology, 2002, 148, 87–102 CrossRef CAS PubMed.
- Y. Shen, T. Köller, B. Kreikemeyer and D. C. Nelson, J. Antimicrob. Chemother., 2013, 68, 1818–1824 CrossRef CAS PubMed.
- V. C. Oliveira, F. L. Bim, R. M. Monteiro, A. P. Macedo, E. S. Santos, C. H. Silva-Lovato, H. F. O. Paranhos, L. D. R. Melo, S. B. Santos and E. Watanabe, Front. Microbiol., 2020, 11, 580779 CrossRef PubMed.
- T. Wei, Q. Yu and H. Chen, Adv. Healthcare Mater., 2019, 8, 3 Search PubMed.
- D. Schmaljohann, Adv. Drug Delivery Rev., 2006, 58, 1655–1670 CrossRef CAS PubMed.
- B. M. Geilich, I. Gelfat, S. Sridhar, A. L. van de Ven and T. J. Webster, Biomaterials, 2017, 119, 78–85 CrossRef CAS PubMed.
- M. Mathiyazhakan, W. Chan, C. D. Ohl and C. Xu, J. Visualized Exp., 2016, 108, e53619 Search PubMed.
- L. J. Delaney, D. Macdonald, J. Leung, K. Fitzgerald, A. M. Sevit, R. Eisenbrey, N. Patel, F. Forsberg, C. K. Kepler, T. Fang, S. M. Kurtz and N. J. Hickok, Acta Biomater., 2019, 93, 12–24 CrossRef CAS PubMed.
- K. Glinel, C. Déjugnat, M. Prevot, B. Schöler, M. Schönhoff and R. V. Klitzing, Colloids Surf., A, 2007, 303, 3–13 CrossRef CAS.
- K. Sato, K. Yoshida, S. Takahashi and J.-I. Anzai, Adv. Drug Delivery Rev., 2011, 63, 809–821 CrossRef CAS PubMed.
- P. C. Balaure and A. M. Grumezescu, Nanomaterials, 2020, 10, 1–53 Search PubMed.
- W. Zhou, Y. Li, J. Yan, P. Xiong, Q. Li, Y. Cheng and Y. Zheng, Sci. Rep., 2018, 8, 13432 CrossRef PubMed.
- H. S. Lee, S. S. Dastgheyb, N. J. Hickok, D. M. Eckmann and R. J. Composto, Biomacromolecules, 2015, 16, 650–659 CrossRef CAS PubMed.
- D. Alkekhia, P. T. Hammond and A. Shukla, Annu. Rev. Biomed. Eng., 2020, 22, 1–24 CrossRef CAS PubMed.
- A. S. Cross, Crit. Care, 2008, 12, 196 CrossRef PubMed.
- A. Casadevall and L. A. Pirofski, J. Water Health, 2009, 7, 2–18 CrossRef PubMed.
- A. M. Grumezescu and C. M. Chifiriuc, Curr. Med. Chem., 2014, 21, 3311–3311 CrossRef CAS PubMed.
- J. Borges and J. F. Mano, Chem. Rev., 2014, 114, 8883–8942 CrossRef CAS PubMed.
- S. Zhang, M. Xing and B. Li, Int. J. Mol. Sci., 2018, 19, 1641 CrossRef PubMed.
- K. Yoshida, Y. Hasebe, S. Takahashi, K. Sato and J. I. Anzai, Mater. Sci. Eng., C, 2014, 34, 384–392 CrossRef CAS PubMed.
- Z. Zhang, J. Zeng, J. Groll and M. Matsusaki, Biomater. Sci., 2022, 10, 4077–4094 RSC.
- J. J. Richardson, M. Björnmalm and F. Caruso, Science, 2015, 348, 6233 CrossRef PubMed.
- A. Escobar, N. Muzzio and S. E. Moya, Pharmaceutics, 2021, 13, 1–23 Search PubMed.
- J. S. Moskowitz, M. R. Blaisse, R. E. Samuel, H. P. Hsu, M. B. Harris, S. D. Martin, J. C. Lee, M. Spector and P. T. Hammond, Biomaterials, 2010, 31, 6019–6030 CrossRef CAS PubMed.
- E. D. de Avila, A. G. B. Castro, O. Tagit, B. P. Krom, D. Löwik, A. A. van Well, L. J. Bannenberg, C. E. Vergani and J. J. J. P. van den Beucken, Appl. Surf. Sci., 2019, 488, 194–204 CrossRef CAS.
- M. M. Hasani-Sadrabadi, S. Pouraghaei, E. Zahedi, P. Sarrion, M. Ishijima, E. Dashtimoghadam, N. Jahedmanesh, S. Ansari, T. Ogawa and A. Moshaverinia, J. Dent. Res., 2021, 100, 1161–1168 CrossRef CAS PubMed.
- Y. Wu, Y. Long, Q. L. Li, S. Han, J. Ma, Y. W. Yang and H. Gao, ACS Appl. Mater. Interfaces, 2015, 7, 17255–17263 CrossRef CAS PubMed.
- Q. Yao, Z. Ye, L. Sun, Y. Jin, Q. Xu, M. Yang, Y. Wang, Y. Zhou, J. Ji, H. Chen and B. Wang, J. Mater. Chem. B, 2017, 5, 8532–8541 RSC.
- B. Wang, H. Liu, L. Sun, Y. Jin, X. Ding, L. Li, J. Ji and H. Chen, Biomacromolecules, 2018, 19, 85–93 CrossRef CAS PubMed.
- Q. Xu, X. Li, Y. Jin, L. Sun, X. Ding, L. Liang, L. Wang, K. Nan, J. Ji, H. Chen and B. Wang, Nanoscale, 2017, 9, 19245–19254 RSC.
- H. Cai, P. Wang and D. Zhang, J. Drug Delivery Sci. Technol., 2019, 54, 101251 CrossRef CAS.
- G. Xu, P. Liu, D. Pranantyo, K. G. Neoh and E. T. Kang, ACS Sustainable Chem. Eng., 2018, 6, 3916–3926 CrossRef CAS.
- A. Zhou, Y. Zhang, X. Zhang, Y. Deng, D. Huang, C. Huang and Q. Qu, Int. J. Biol. Macromol., 2022, 201, 448–457 CrossRef CAS PubMed.
- B. Kaczmarek, M. Wekwejt, K. Nadolna, A. Owczarek, O. Mazur and A. Palubicka, J. Mech. Behav. Biomed. Mater., 2020, 110, 103916 CrossRef CAS PubMed.
- B. Hu, Y. Shen, J. Adamcik, P. Fischer, M. Schneider, M. J. Loessner and R. Mezzenga, ACS Nano, 2018, 12, 3385–3396 CrossRef CAS PubMed.
- I. Zhuk, F. Jariwala, A. B. Attygalle, Y. Wu, M. R. Libera and S. A. Sukhishvili, ACS Nano, 2014, 8, 7733–7745 CrossRef CAS PubMed.
- V. Albright, I. Zhuk, Y. Wang, V. Selin, B. van de Belt-Gritter, H. J. Busscher, H. C. van der Mei and S. A. Sukhishvili, Acta Biomater., 2017, 61, 66–74 CrossRef CAS PubMed.
- S. Pavlukhina, Y. Lu, A. Patimetha, M. Libera and S. Sukhishvili, Biomacromolecules, 2010, 11, 3448–3456 CrossRef CAS PubMed.
- Y. Lu, Y. Wu, J. Liang, M. R. Libera and S. A. Sukhishvili, Biomaterials, 2015, 45, 64–71 CrossRef CAS PubMed.
- S. Pavlukhina, I. Zhuk, A. Mentbayeva, E. Rautenberg, W. Chang, X. Yu, B. Van De Belt-Gritter, H. J. Busscher, H. C. Van Der Mei and S. A. Sukhishvili, NPG Asia Mater., 2014, 6, 1–10 Search PubMed.
- V. Albright, D. Penarete-Acosta, M. Stack, J. Zheng, A. Marin, H. Hlushko, H. Wang, A. Jayaraman, A. K. Andrianov and S. A. Sukhishvili, Biomaterials, 2021, 268, 120586 CrossRef CAS PubMed.
- X. Wang, J. Wu, P. Li, L. Wang, J. Zhou, G. Zhang, X. Li, B. Hu and X. Xing, ACS Appl. Mater. Interfaces, 2018, 10, 34905–34915 CrossRef CAS PubMed.
- A. M. Bhayo, Y. Yang and X. He, Prog. Mater. Sci., 2022, 130, 101000 CrossRef.
- M. Krishnamoorthy, S. Hakobyan, M. Ramstedt and J. E. Gautrot, Chem. Rev., 2014, 114, 10976–11026 CrossRef CAS PubMed.
- S. Edmondson, V. L. Osborne and W. T. S. Huck, Chem. Soc. Rev., 2004, 33, 14–22 RSC.
- H. Jiang and F. J. Xu, Chem. Soc. Rev., 2013, 42, 3394–3426 RSC.
- R. Barbey, L. Lavanant, D. Paripovic, N. Schüwer, C. Sugnaux, S. Tugulu and H. A. Klok, Chem. Rev., 2009, 109, 5437–5527 CrossRef CAS PubMed.
- X. Xiao, S. Zhang, S. Chen, Y. Qian, J. Xie, Z. Cong, D. Zhang, J. Zou, W. Zhang, Z. Ji, R. Cui, Z. Qiao, W. Jiang, Y. Dai, Y. Wang, X. Shao, Y. Sun, J. Xia, J. Fei and R. Liu, Biomater. Sci., 2020, 8, 6883–6889 RSC.
- E. Koufakis, T. Manouras, S. H. Anastasiadis and M. Vamvakaki, Langmuir, 2020, 36, 3482–3493 CrossRef CAS PubMed.
- A. Wang, S. Duan, X. Ding, N. Zhao, Y. Hu, X. Ding and F. Xu, Adv. Funct. Mater., 2021, 31, 18 Search PubMed.
- Y. Rao, X. Zou, X. Shen, H. Zhang, S. Gao, J. Guo and H. Chen, Biomacromolecules, 2024, 25, 89–103 CrossRef CAS PubMed.
- N. Li, H.-K. Luo, A. X. Chen, J. P. K. Tan, C. Yang, M. J. Y. Ang, H. Zeng and Y. Y. Yang, ACS Appl. Mater. Interfaces, 2023, 15, 354–363 CrossRef CAS PubMed.
- S. Dhingra, A. Joshi, N. Singh and S. Saha, Mater. Sci. Eng., C, 2021, 118, 111465 CrossRef CAS PubMed.
- A. Wang, S. Duan, Y. Hu, X. Ding and F. Xu, ACS Appl. Mater. Interfaces, 2022, 14, 44173–44182 CrossRef CAS PubMed.
- M. Ramstedt, N. Cheng, O. Azzaroni, D. Mossialos, H. J. Mathieu and W. T. S. Huck, Langmuir, 2007, 23, 3314–3321 CrossRef CAS PubMed.
- Y. C. Tai, J. McGuire and J. A. Neff, J. Colloid Interface Sci., 2008, 322, 104–111 CrossRef CAS PubMed.
- I. Fundeanu, H. C. van der Mei, A. J. Schouten and H. J. Busscher, Colloids Surf., B, 2008, 64, 297–301 CrossRef CAS PubMed.
- Q. Ye, B. He, Y. Zhang, J. Zhang, S. Liu and F. Zhou, ACS Appl. Mater. Interfaces, 2019, 11, 39171–39178 CrossRef CAS PubMed.
- M. R. Nejadnik, A. F. Engelsman, I. C. Saldarriaga Fernandez, H. J. Busscher, W. Norde and H. C. van der Mei, J. Antimicrob. Chemother., 2008, 62, 1323–1325 CrossRef CAS PubMed.
- M. R. Nejadnik, H. C. Van Der Mei, H. J. Busscher and W. Norde, Appl. Environ. Microbiol., 2008, 74, 916–919 CrossRef CAS PubMed.
- M. R. Nejadnik, H. C. van der Mei, W. Norde and H. J. Busscher, Biomaterials, 2008, 29, 4117–4121 CrossRef CAS PubMed.
- Y. He, X. Wan, W. Lin, J. Li, Z. Li, F. Luo, J. Li, H. Tan and Q. Fu, Biomater. Sci., 2020, 8, 6890–6902 RSC.
- S. Nastyshyn, Y. Stetsyshyn, J. Raczkowska, Y. Nastishin, Y. Melnyk, Y. Panchenko and A. Budkowski, Polymers, 2022, 14, 4245 CrossRef CAS PubMed.
- V. Yadav, Y. A. Jaimes-Lizcano, N. K. Dewangan, N. Park, T.-H. Li, M. L. Robertson and J. C. Conrad, ACS Appl. Mater. Interfaces, 2017, 9, 44900–44910 CrossRef CAS PubMed.
- B. Xu, C. Feng, J. Hu, P. Shi, G. Gu, L. Wang and X. Huang, ACS Appl. Mater. Interfaces, 2016, 8, 6685–6692 CrossRef CAS PubMed.
- J. Yang, H. Chen, S. Xiao, M. Shen, F. Chen, P. Fan, M. Zhong and J. Zheng, Langmuir, 2015, 31, 9125–9133 CrossRef CAS PubMed.
- G. J. Dunderdale, J. Patrick and A. Fairclough, Langmuir, 2013, 29, 3628–3635 CrossRef CAS PubMed.
- T. Liu, S. Yan, R. Zhou, X. Zhang, H. Yang, Q. Yan, R. Yang and S. Luan, ACS Appl. Mater. Interfaces, 2020, 12, 42576–42585 CrossRef CAS PubMed.
- S. Yan, H. Shi, L. Song, X. Wang, L. Liu, S. Luan, Y. Yang and J. Yin, ACS Appl. Mater. Interfaces, 2016, 8, 24471–24481 CrossRef CAS PubMed.
- X. Jin, Y. H. Xiong, X. Y. Zhang, R. Wang, Y. Xing, S. Duan, D. Chen, W. Tian and F. J. Xu, Adv. Funct. Mater., 2019, 29, 1–12 Search PubMed.
- Y. Zhang, X. Zhang, Y. Q. Zhao, X. Y. Zhang, X. Ding, X. Ding, B. Yu, S. Duan and F. J. Xu, Biomater. Sci., 2020, 8, 997–1006 RSC.
- L. Sun, L. Song, X. Zhang, S. Yuan and S. Luan, J. Mater. Sci. Technol., 2022, 126, 191–202 CrossRef CAS.
- Y. H. Ding, M. Floren and W. Tan, Biosurf. Biotribol., 2016, 2, 121–136 CrossRef CAS PubMed.
- J. F. Rocha, L. H. Hasimoto and M. Santhiago, Anal. Bioanal. Chem., 2023, 415, 3799–3816 CrossRef CAS PubMed.
- J. H. Ryu, P. B. Messersmith and H. Lee, ACS Appl. Mater. Interfaces, 2018, 10, 7523–7540 CrossRef CAS PubMed.
- Z. Xu, T. Wang and J. Liu, Int. J. Mol. Sci., 2022, 23, 7278 CrossRef CAS PubMed.
- R. Su, H. Yan, P. Li, B. Zhang, Y. Zhang and W. Su, Photodiagn. Photodyn. Ther., 2021, 35, 1572–1000 Search PubMed.
- P. Kord Forooshani, E. Polega, K. Thomson, M. S. A. Bhuiyan, R. Pinnaratip, M. Trought, C. Kendrick, Y. Gao, K. A. Perrine, L. Pan and B. P. Lee, Front. Chem., 2019, 7, 631 CrossRef PubMed.
- G. Fichman, C. Andrews, N. L. Patel and J. P. Schneider, Adv. Mater., 2021, 33, 40 CrossRef PubMed.
- L. Han, P. Li, P. Tang, X. Wang, T. Zhou, K. Wang, F. Ren, T. Guo and X. Lu, Nanoscale, 2019, 11, 15846–15861 RSC.
- D. He, T. Yang, W. Qian, C. Qi, L. Mao, X. Yu, H. Zhu, G. Luo and J. Deng, Appl. Mater. Today, 2018, 12, 415–429 CrossRef.
- Y. Zhang, W. Jiang, L. Lei, Y. Wang, R. Xu, L. Qin and Q. Wei, Langmuir, 2022, 38, 7157–7167 CrossRef CAS PubMed.
- S. Mao, D. Zhang, X. He, Y. Yang, I. Protsak, Y. Li, J. Wang, C. Ma, J. Tan and J. Yang, ACS Appl. Mater. Interfaces, 2021, 13, 3089–3097 CrossRef CAS PubMed.
- Y. J. Fan, M. T. Pham and C. J. Huang, Langmuir, 2019, 35, 1642–1651 CrossRef CAS PubMed.
- A. B. Asha, Y. Chen, H. Zhang, S. Ghaemi, K. Ishihara, Y. Liu and R. Narain, Langmuir, 2019, 35, 1621–1630 CrossRef CAS PubMed.
- L. Yang, L. Li, H. Li, T. Wang, X. Ren, Y. Cheng, Y. Li and Q. Huang, Adv. Healthcare Mater., 2022, 11, 1–9 Search PubMed.
- L. Yang, C. Wang, L. Li, F. Zhu, X. Ren, Q. Huang, Y. Cheng and Y. Li, Adv. Funct. Mater., 2022, 32, 1–10 Search PubMed.
- A. B. Asha, Y. Y. Peng, Q. Cheng, K. Ishihara, Y. Liu and R. Narain, ACS Appl. Mater. Interfaces, 2022, 14, 9557–9569 CrossRef CAS PubMed.
- S. D. Bull, M. G. Davidson, J. M. H. Van Den Elsen, J. S. Fossey, A. T. A. Jenkins, Y. B. Jiang, Y. Kubo, F. Marken, K. Sakurai, J. Zhao and T. D. James, Acc. Chem. Res., 2013, 46, 312–326 CrossRef CAS PubMed.
- M. Mittal, M. R. Siddiqui, K. Tran, S. P. Reddy and A. B. Malik, Antioxid. Redox Signaling, 2014, 20, 1126–1167 CrossRef CAS PubMed.
- K. Rajamäki, T. Nordström, K. Nurmi, K. E. O. Åkerman, P. T. Kovanen, K. Öörni and K. K. Eklund, J. Biol. Chem., 2013, 288, 13410–13419 CrossRef PubMed.
- A. P. West, I. E. Brodsky, C. Rahner, D. K. Woo, H. Erdjument-Bromage, P. Tempst, M. C. Walsh, Y. Choi, G. S. Shadel and S. Ghosh, Nature, 2011, 472, 476–480 CrossRef CAS PubMed.
- S. Lee, S. Inzerillo, G. Y. Lee, E. M. Bosire, S. K. Mahato and J. Song, Trends Microbiol., 2022, 30, 254–267 CrossRef CAS PubMed.
- V. Wittmann and R. J. Pieters, Chem. Soc. Rev., 2013, 42, 4492 RSC.
- B. Bouvier, J. Chem. Inf. Model., 2016, 56, 1193–1204 CrossRef CAS PubMed.
- M. Ye, Y. Zhao, Y. Wang, M. Zhao, N. Yodsanit, R. Xie, D. Andes and S. Gong, Adv. Mater., 2021, 33(9), 9 CrossRef PubMed.
- G. Crivello, L. Fracchia, G. Ciardelli, M. Boffito and C. Mattu, Nanomaterials, 2023, 13, 904 CrossRef CAS PubMed.
|
This journal is © The Royal Society of Chemistry 2024 |
Click here to see how this site uses Cookies. View our privacy policy here.