Insight into the development and proceedings of Au@Al–CeVO4 catalysts for water splitting: an advanced outlook for hydrogen generation with sunlight†
Received
24th November 2023
, Accepted 11th January 2024
First published on 11th January 2024
Abstract
In this study, we report a promising approach and new catalysts, namely Au@Al–CeVO4 photocatalysts, for sustainable hydrogen production from water splitting. Al doping into CeVO4 was done hydrothermally to enhance the electron quenching centres. The structural and optical properties of as-synthesized catalysts were evaluated with XRD, SEM, BET, AFM, EIS and UV-vis–DRS techniques. The purity and chemical compositions were confirmed with EDX and XPS techniques. Electron impedance spectroscopy and photoluminescence were used to ensure the charge transfer mechanism for water splitting on Au@Al–CeVO4. Photocatalytic reactions were done in a quartz reactor, whereas hydrogen generation activities were monitored on a GC-TCD (Shimadzu-2010/Japan). The presence of Au cocatalysts on Al–CeVO4 surfaces offers more highly localized electrons to accelerate water reduction. The results of this study show that Au1.0@Al–CeVO4 was the most active catalyst, delivering 52.93 mmol g−1 h−1 of hydrogen compared to bare CeVO4 or Al–CeVO4 catalysts that deliver 2.57 and 8.69 mmol g−1 h−1, respectively. It has been found that gold loading and Al doping reduce the back reaction by localized surface plasmon electrons and electron quenching, respectively. In addition, gold cocatalysts and Al doping enhanced the catalytic sites and light harvesting ability of Au@Al–CeVO4. On the basis of these results, it can be concluded that the aforementioned approach shows eventual promise to replace conventional technologies used for hydrogen generation.
Introduction
In the current era, the exploitation of water as a renewable source for the production of hydrogen has gained significant attention from researchers. Water splitting using photocatalysts is the most recent way to produce hydrogen by the conversion of solar energy into chemical energy.1 In the search for renewable energy sources and environmental sustainability, hydrogen has emerged as a promising alternative fuel. However, current hydrogen generation sources are fossil fuels that are non-renewable and have adverse environmental impacts.2 To resolve this issue, scientists have introduced photocatalytic water splitting for the production of hydrogen energy which is eco-friendly. Recently, great attention has been paid to produce hydrogen energy via a water splitting reaction.3 It offers numerous advantages, such as scalability, environmental friendliness and ability to operate under ambient conditions.4,5 The development of efficient and stable photocatalysts holds immense potential for unlocking the full power of solar energy in driving the sustainable production of hydrogen.6
Photocatalysts play an important role in photoreactions for the generation of hydrogen. Various photocatalysts, including CdS, TiO2, ZnO, and WO3 have been found to be efficient catalysts for hydrogen production.7,8 In 1972, Fujishima and Honda for the first time in history reported H2 production from water splitting using a TiO2 catalyst in the presence of electricity and light.9 Similarly, several photocatalysts have been developed and exposed for photocatalytic hydrogen generation. Metal vanadates, including BiVO4, CrVO4, FeVO4 and Zn3V2O8, have also been reported for photocatalytic water splitting reactions.10–17 CeVO4 is an efficient photocatalyst with a suitable bandgap of 2.8 eV, and it is a commercially available and cost-effective material.18 Some drawbacks were found in these photocatalysts, including rapid recombination of charge carriers and insufficient charge transfer.19 These drawbacks result in the low activity of photocatalysts for hydrogen production.20
The activity of photocatalysts can be improved by several approaches, including the most efficient approach which is the formation of composite materials.21 This approach involves the combination of two or more materials to produce heterostructures that take advantage of the properties of each material. Photocatalysts can be designed to improve charge transfer and separation, increase light absorption range and reduce recombination of charge carriers.22 For example, a composite of CdS and TiO2 has been found to be efficient for enhancing the photocatalytic performance of both materials, due to improved charge transfer and separation.23–26 Recently, metal organic framework (MOF) derived composites have been synthesized and utilized for clean energy production.27,28 Metal loading and doping are also very promising strategies for the development of catalysts for photocatalytic applications.29,30
Numerous materials have been synthesized for photoreactions, and among them, rare earth metal vanadates have been widely used due to their excellent optical, electrical, and catalytic properties.31 In particular, CeVO4 exhibits remarkable optical properties and a redox nature. It has a tetragonal zircon-like structure which stabilizes cerium ions under oxidizing conditions.32,33 CeVO4 has attracted attention due to its well-defined size and morphology, which were obtained during synthesis of this material by a hydrothermal method. This material is mostly used for the oxidation and dehydrogenation of organic compounds.34 Scientists have developed CeVO4 with different morphologies by applying the hydrothermal method and have used it for the photocatalytic reduction of CO2 and degradation of hazardous organic compounds.35
The formation of an Au@Al–CeVO4 nanocomposite is a promising strategy for improving the effectiveness and efficiency of photocatalysts with potential applications in a wide range of fields, including water treatment and solar energy conversion.36,37 Herein, we synthesized CeVO4 nanoparticles, and to improve their photocatalytic activity, we doped Al particles into CeVO4 and loaded Au metal over it. Au@Al–CeVO4 photocatalysts with 1% overall Au loading exhibit 52.93 mmol g−1 h−1 H2 production, twenty times higher than that of pristine CeVO4 due to the LSPR of both (Au, Al) metals. Al doping enhances the transfer of electrons from the semiconductor valence band towards the conduction band due to its electron quenching ability. Further, we have studied the role of sacrificial agents and factors affecting the activity of photocatalysts.
Experimental
Materials
Ammonium cerium nitrate 98% (Sigma Aldrich-CAS No. 16774-21-3), iron nitrate 96% (Sigma Aldrich-CAS No. 7782-61-8), ammonium vanadate 99% (Sigma Aldrich-CAS No. 7803-55-6), aluminium nitrate nonahydrate 98% (Sigma Aldrich-CAS No. 7784-27-2), HAuCl4 acid (Sigma Aldrich-CAS No. 27988-77-8), NaBH4 98% (Sigma Aldrich-CAS No. 16940-66-2), hydrogen peroxide 99% (Sigma Aldrich-CAS No. 7722-84-1), hydrochloric acid 37% (Sigma Aldrich-CAS No. 7647-01-0), NaOH 98% (Sigma Aldrich-CAS No. 1310-73-2), methanol 98% (Sigma Aldrich-CAS No. 67-56-1) and deionized water 99% (ZYC-Sichuan Zhuoyue Ltd) (Fig. 1).
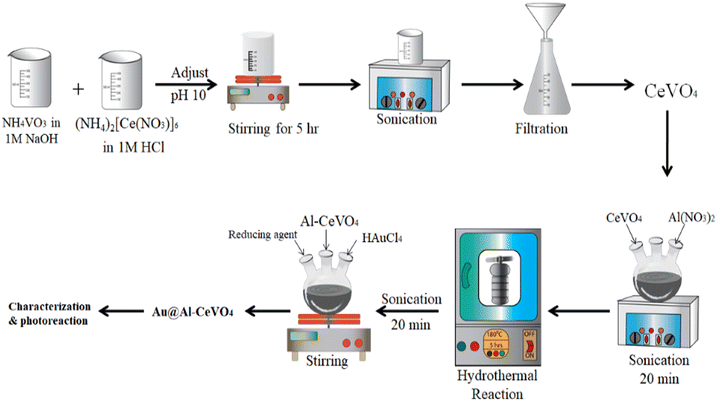 |
| Fig. 1 Schematic of the synthesis of the catalysts. | |
Synthesis of photocatalyst
First, an 8 mM solution of ammonium cerium nitrate (4.38 g) in 30 mL of distilled water was prepared. Then, 3 mL of hydrogen peroxide (H2O2 6%) was added to the ammonium cerium nitrate solution and stirred for 0.5 h after which 5 mL of HCl solution was added. Then, we added 0.936 g of NH4VO3 to 30 mL of NaOH (1 M) to prepare the 8 mM solution. Further, ammonium cerium nitrate solution was added dropwise into the NH4VO3 solution with a dropper under continuous stirring and heating. The solution mixture was sonicated for 30 minutes and stirred and heated for 1 h. Later, 1 M NaOH solution was added to the reaction mixture by dropper to maintain the pH of the reaction mixture at 9. The reaction mixture was again stirred. During stirring, brown precipitates appeared; these precipitates were washed and filtered. We placed the reaction mixture in a Teflon-lined stainless steel autoclave reactor at 180 °C for the hydrothermal reaction. After hydrothermal treatment, the precipitates were washed with distilled water several times to remove impurities. Aluminium nitrate was dissolved in distilled water, then this prepared solution was added to the CeVO4 powder and the solution mixture was continuously stirred for 3 h to make a homogeneous dispersion of aluminium. The resultant suspension was placed in a Teflon-lined stainless steel autoclave reactor for hydrothermal reaction at 180 °C. After that, we separated the Al-doped CeVO4 product by filtration and washed the obtained product with distilled water to remove undesirable by-products. For the deposition of Au, we prepared a solution of chloroauric acid and added it to Al-doped CeVO4, then stirred the solution mixture for 2 h to ensure the contact of Au metal with Al-doped CeVO4. Further, NaBH4 solution was added dropwise and the mixture was stirred to reduce the Au metal. Finally, we filtered out the precipitates by vacuum filtration, dried them in an oven for 2 h at 70 °C, and collected the brown powder for calcination at 500 °C for 5 h.
Hydrogen evolution experiment
The hydrogen evolution experiment is a scientific procedure used to study the production of hydrogen gas from water. The experiment involves the use of a photocatalyst, which is a material that absorbs light and initiates a chemical reaction.38 When a photocatalyst is illuminated with light, it generates an electron (e−) and hole (h+) pair, which can be utilized to split water molecules into hydrogen and oxygen molecules.39 The hydrogen evolution experiment was carried out in a gas chromatogram (GC) furnished with a thermal conductivity detector (TCD). Typically, a GC consists of a reaction vessel, a light source, and a gas collection system. The reaction vessel was filled with an aqueous solution, including the photocatalyst and a sacrificial reagent of 5% ethanol and water mixture.40 Solar radiation was used as a light source to initiate the photocatalytic reaction, and a gas collection system was used to measure the amount of hydrogen produced. The results obtained from hydrogen evolution experiments can be used to evaluate the performance of photocatalysts for hydrogen evolution. Several factors, such as the intensity of light and photocatalyst reaction conditions, can affect the efficiency of photocatalysts for hydrogen production.41 By optimizing these factors, it is possible to improve the performance of photocatalysts to increase the rate of hydrogen generation. The hydrogen evolution experiment has numerous applications, including the development of sustainable energy sources, water purification, and wastewater treatment.42,43 The actual quantum efficiency of the hydrogen evolution experiment is measured by the number of hydrogen molecules produced per photon absorbed by the system. The equation for quantum efficiency is given below:
To determine the number of moles of hydrogen produced, convert the volume of hydrogen gas produced by the hydrogen evolution reaction into the number of moles by using the ideal gas equation.44 To determine the number of photons absorbed, measure the intensity of light using a calibrated photodetector and the area of photocatalyst (electrode) surface. After obtaining these measurements, the quantum efficiency can be calculated by plugging them into the above equation. It is worth noting that calculating the quantum efficiency of a hydrogen evolution experiment requires careful control over experimental conditions to accurately measure the number of moles of hydrogen produced and the number of photons absorbed.
Characterization
XRD analyses was performed on a Siemens D5000 diffractometer with an X-ray source Cu Kα (λ = 1.54 Å, 40 mA, 40 kV) fitted with a curved graphite filter. A Bruker Alpha Platinum ATR instrument was used for Fourier transform infrared (FTIR) analyses (spectral range: 7200–390 cm−1). For optical properties, UV-vis/DRS results were obtained on an SP-IUV7 spectrophotometer (wavelength accuracy = +0.5 nm, wavelength range = 180–1050 nm). SEM analysis was conducted on an FEI Nova 470 Nano SEM furnished with EDX analysis for the elemental composition of catalysts. Atomic force microscopy (AFM) was done with a PARK NX10 instrument. XPS results were obtained with a Kratos Axis Ultra DLD fitted with a hemispherical electron energy analyser. For EIS analysis, a Solartron impedance analyser was used (frequency range = 0.1 Hz−1 MHz, amplitude = 0.5–110 mV). Photoluminescence (PL) results were obtained with an MFLI Lock-in Amplifier (Band width = 500 kHz). A conventional three-electrode system potentiostat (Artisan Tech-CHI 660) was used to conduct photocurrent tests (visible light irradiation λ > 420 nm).
Results and discussion
XRD analysis
To determine the crystal structure, particle size and phase composition45 of as-synthesized catalysts, X-ray diffraction (XRD) analysis was performed. For the obtained XRD patterns of CeVO4, Al–CeVO4 and Au@Al–CeVO4, see Fig. 2(a). The 2 theta range for the CeVO4 patterns was 10–80°. The major XRD patterns of pure CeVO4 were observed at 24.94°, 33.28°, 35.50°, 44.64°, 49.05°, 50.64°, 57.09°, 61.67°, 63.25°, 72.69° and 78.53° corresponding to hkl values (200), (113), (202), (103), (400), (213), (402), (336), (206), (440) and (404), respectively. The crystallite size of CeVO4 was calculated using the Scherrer formula, and the obtained size of CeVO4 was 22.17 nm. The crystal structure of CeVO4 is tetragonal and its density is 4.83 g cm−3.46 The XRD patterns of Al-doped CeVO4 were similar to those of CeVO4, and no distinct peaks of Al were observed due to its low concentration. However, an increase in the base line intensity of the diffraction patterns was observed that confirms Al doping. Au-loaded Al–CeVO4 XRD patterns are exhibited in Fig. 2(a) where an extra peak of Au was observed at 38.18° corresponding to hkl value (111). No Au metal peak in the XRD patterns was obvious due to its low concentration. The crystallite size of the Au@Al–CeVO4 photocatalyst was 23.27 nm.
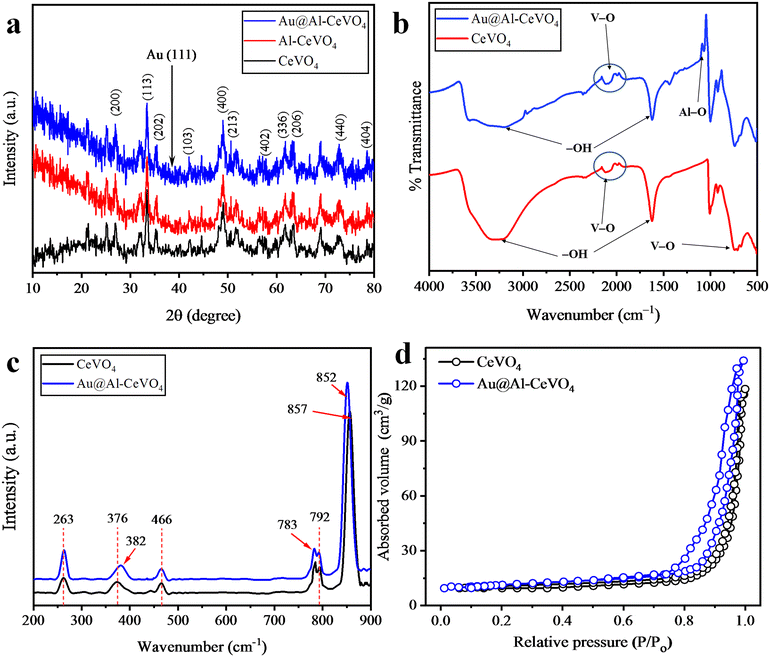 |
| Fig. 2 (a) XRD patterns of as-synthesized CeVO4, Al–CeVO4 and Au@Al–CeVO4, (b) FTIR analyses, (c) Raman results and (d) BET analyses. | |
FTIR and Raman analysis
FTIR analysis was conducted to analyse the linkage of the elements of photocatalysts.47Fig. 2(b) reveals that the V–O bond linkage in VO4 tetrahedron exhibited two distinct bending and stretching vibration peaks at 785 and 2125 cm−1, respectively, and the Al–O bond linkage was observed at 1070 cm−1. Two strong bending and stretching vibrations of –OH were observed at 1620 and 3420 cm−1, respectively, due to the presence of moisture in the photocatalytic material.48 No distinct peak for Au was observed because it exists in a metallic form. Raman analysis of the as-synthesized photocatalyst was performed to analyse the vibrational modes of the catalyst particles.49 The Raman spectrum of CeVO4 is presented in Fig. 2(c) in the scan range 200–900 cm−1. A1g symmetric vibrations of the V–O bond appeared at 857 cm−1, and Eg and B2g antisymmetric vibrations of the V–O bond appeared at 783 and 792 cm−1. Vibrational peaks appearing at 376 and 466 cm−1 correspond to B2g and B1g deformations in CeVO4. After Au loading, a peak shift was observed from 857 to 852 cm−1 due to the photoconfinement effect of Au metal.
BET analysis
The catalyst surface and pore structure are the most important factors affecting the activity of a photocatalyst.50 The nitrogen gas adsorption/desorption method was employed to measure the surface area of CeVO4 and Au@Al–CeVO4. The pore diameter of CeVO4 was measured to be 29.53 nm, while the surface area was determined to be 8.67 m2 g−1. The Au@Al–CeVO4 catalyst follows desorption/adsorption type II isotherm; see Fig. 2(d). The surface area and pore diameter of Au@Al–CeVO4 were calculated to be 10.70 m2 g−1 and 32.56 nm, respectively.
SEM and EDX analyses
The surface morphology and elemental composition of as-synthesized photocatalysts were determined from SEM images and the EDX spectrum respectively.51 The SEM images were observed at 10 and 1 μm resolution, as shown in Fig. 3(a and b). Decent nanosheets of CeVO4 were obtained with an uneven distribution of Au particles and some Al particles were embedded in the semiconductor material; see Fig. 3(b). Calcination of CeVO4 at 550 °C caused the formation of nanosheets, resulting in an increase in the surface area of the semiconductor material. The elemental composition of the photocatalyst was determined by EDX analysis. Fig. 3(c) confirms that all elements of photocatalysts are present, and no foreign element was found in the EDX spectrum, which confirms that the as-synthesized material is pure. Al and Au metals were added in small quantities, due to which their percentage in the EDX spectrum is low.
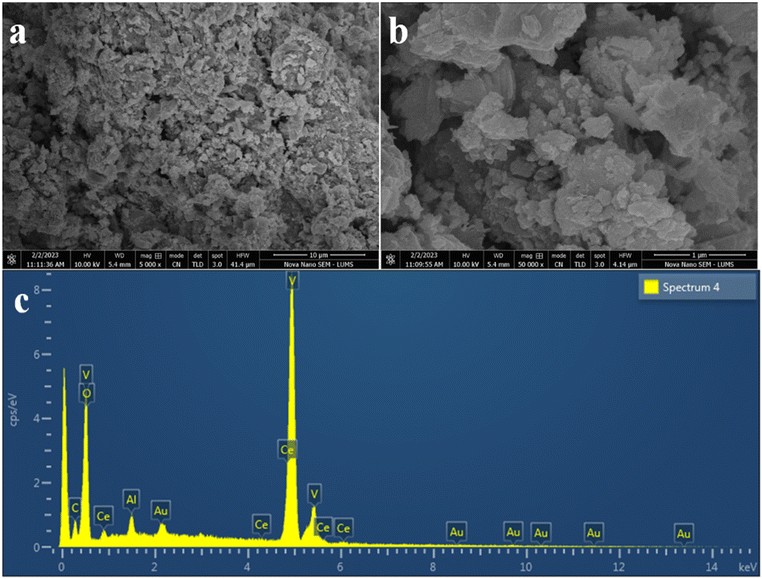 |
| Fig. 3 (a and b) SEM images and (c) EDX spectra of Au@Al–CeVO4 photocatalysts. | |
AFM analysis
AFM analysis was performed to determine the height and thickness of the as-synthesized photocatalyst. Two-dimensional (2D) and three-dimensional (3D) images of the Au@Al–CeVO4 catalyst are exhibited in Fig. 4(a, b, d and e). The height of the photocatalyst measured by AFM analysis is 500 nm. The height and surface roughness of the photocatalyst are clearly exhibited in the three-dimensional (3D) images. The scan area for the measurement of height and thickness of the photocatalyst were 0.0–0.5 μm and 6.04 × 6.04 μm. The surface of the photocatalyst is rough and its particle distribution is random. For a histogram representing the overall height of photocatalysts, see Fig. 4(c).
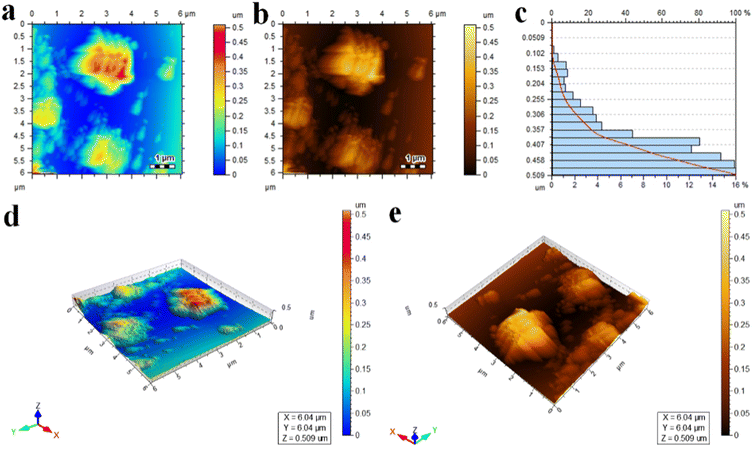 |
| Fig. 4 AFM analysis (a and b) 2D images, (c) size measurement, and (d and e) 3D images of Au@Al–CeVO4 catalysts. | |
XPS studies
X-ray photon spectroscopy is an excellent technique to identify the chemical state of the atoms present in a photocatalyst.52 Two multiples of Ce 3d binding energies arose due to spin–orbit coupling of 3d5/2 at 880.3 eV and 882.6 eV, whereas 3d3/2 binding energies were observed at 897.0 eV and 903.0 eV. Four peaks for the binding energies of Ce were observed and ascribed to the Ce3+ oxidation state; see Fig. 5(a). Two peaks of V 2p binding energies were observed at 516.1 and 524.2 eV assigned to V 2p3/2 and V 2p1/2, which are characteristic of the V5+ oxidation state, as illustrated in Fig. 5(b). The binding energy of O 1s was observed in the high-resolution spectrum of XPS (see Fig. 5(c)) with peaks obtained at 529.0 and 532.5 eV, suggesting that O was present in the CeVO4 material and Al–O. An Al metal peak was observed at 72.9 eV and was ascribed to the 2p characteristic state, as shown in Fig. 5(d). Au metal peaks were observed at 83.3 and 87.4 eV for Au 4f7/2 and 4f5/2, respectively; see Fig. 5(e).
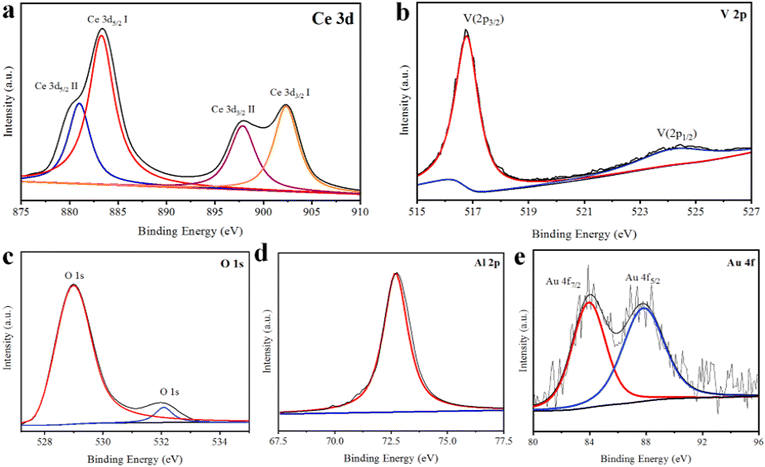 |
| Fig. 5 XPS analysis: (a) Ce binding energy peaks, (b) V binding energy peaks, (c) O binding energy peaks, (d) Al binding energy peaks and (e) Au binding energy peaks of Au@Al–CeVO4 catalysts. | |
UV-vis–DRS measurements
UV-vis/DRS analyses were performed to evaluate the optical absorption properties of pristine CeVO4 and as-synthesized photocatalysts, and the results are illustrated in Fig. 6(a and b). CeVO4 exhibits no absorption peak in the visible region; however, Al–CeVO4 and Au@Al–CeVO4 catalysts exhibit strong absorption peaks between 250 and 350 nm. The absorption of a photocatalyst is relevant to the band gap absorption of CeVO4 (2.93 eV). Extended absorption was observed in the region 400–700 nm. This extended absorption was due to the localized surface plasmon resonance (LSPR) of loaded Au metal;53 see Fig. 6(a). The effect of Al doping and Au loading on the band gap was observed with band gap measurement studies; see Fig. 6(b). After metal loading, the band gap of CeVO4 shifts towards the visible region, and CeVO4 becomes an active photocatalyst for water splitting under visible light.
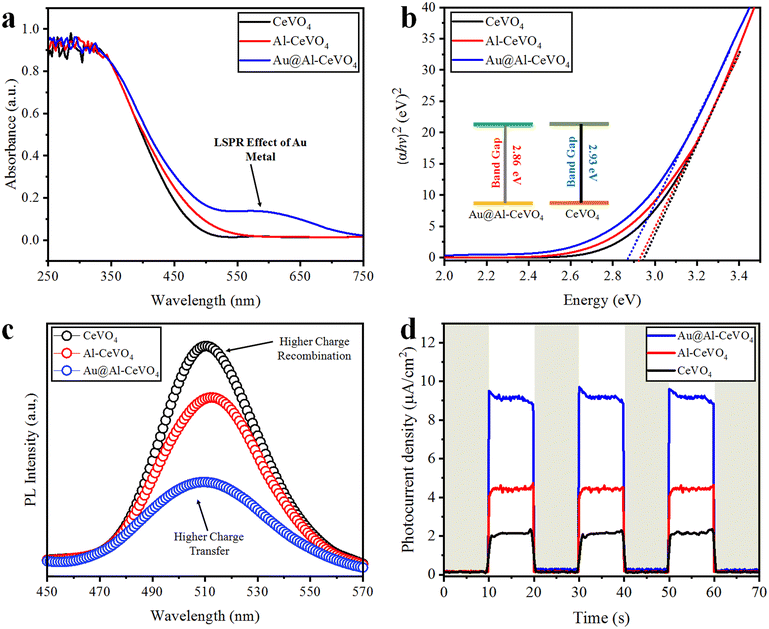 |
| Fig. 6 (a and b) UV-vis/DRS analysis and band gap measurements; (c and d) PL and photocurrent results of as-synthesised catalysts, respectively. | |
PL, EIS and photocurrent analyses
Photoluminescence (PL), electron impedance spectroscopy (EIS) and photocurrent analyses were performed to investigate charge separation, charge transfer efficiency and charge recombination processes.53 Pristine CeVO4 has a low photoresponse and a high recombination rate; see Fig. 6(c). To reduce the charge recombination rate, Au metal was loaded onto the semiconductor and for higher charge transfer, Al metal was doped into CeVO4. In Au@Al–CeVO4 catalyst, low charge recombination and high charge transfer efficiency were confirmed by PL and photocurrent responses; see Fig. 6(c and d). After Au loading, charge recombination was suppressed, lowering the intensity of PL emission. Moreover, electron impedance spectroscopy (EIS) analyses were conducted to measure the charge transfer efficiency of pristine CeVO4, Al–CeVO4 and Au@Al–CeVO4 photocatalysts. EIS analyses revealed that Au@Al–CeVO4 photocatalysts exhibit higher charge transfer efficiency than pristine CeVO4; see Fig. 7(a).
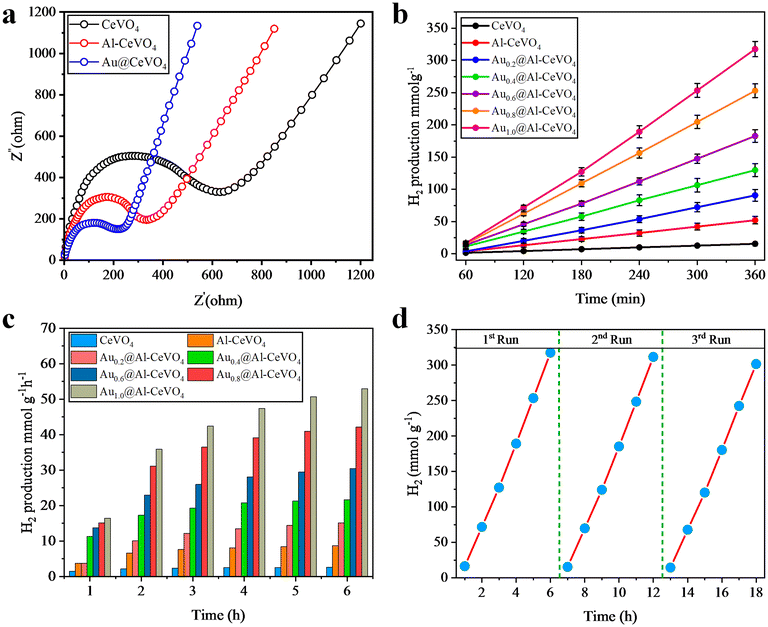 |
| Fig. 7 (a) EIS analyses, (b and c) H2 generation activities and (d) recyclability tests of catalysts. | |
Hydrogen generation activities
All as-synthesized photocatalysts with different Au (wt%) loading on Al–CeVO4 were utilized for the hydrogen generation experiment. Each photocatalyst was utilized for photoreaction in the presence of visible light, and to evaluate hydrogen generation activities of the photocatalyst, each photoreaction was performed for 6 h. In the absence of a photocatalyst or light, no hydrogen was produced. Although CeVO4 is a stable photocatalyst, it produces a very low amount of H2 due to high charge (e−/h+) recombination. After Al doping on CeVO4, the hydrogen generation activity increased because Al promotes charge transfer from the valence band to the conduction band of a semiconductor due to the electron-deficient nature of Al metal. To evaluate the effect of Au metal, a series of photocatalysts with different Au wt% were employed for photoreaction. Overall, the 1% wt Au-loaded photocatalyst (Au1.0@Al–CeVO4) demonstrated the highest activity, producing 52.93 mmol g−1 h−1 of hydrogen. This was greater than the hydrogen generation observed for Au1.2@Al–CeVO4 and Au1.4@Al–CeVO4 photocatalysts in the series (refer to Fig. S6†). Hydrogen generation activity was monitored in units of mmol g−1 h−1 and mmol g−1, and hydrogen generation activities of all photocatalysts are illustrated and tabulated in Fig. 7(b and c) and Table 1. Furthermore, wavelength-dependent activities of the Au1.0@Al–CeVO4 catalyst were measured to confirm the Au metal LSPR phenomenon. Various band filters, such as 400, 500, 600 and 700 nm, were used and centred at 10 nm. Hydrogen generation activities observed during these experiments are illustrated in the ESI;† see Fig. S5. The hydrogen generation activity of photocatalysts at 700 nm was low, but at 600 nm the activity of catalysts for hydrogen generation starts increasing, due to the LSPR of Au metal.
Table 1 H2 generation activities of synthesized photocatalysts
Photocatalysts 
|
Au loading (wt%) on CeVO4 |
H2 evolution (mmol g−1) |
H2 evolution (mmol g−1 h−1) |
Where = 5 mg of each catalyst for photoreaction, 30 mL of water in quartz reactor having volume of 150 mL, and reaction time (6 h). |
CeVO4 |
0.0 |
15.45 ± 3.86 |
2.57 |
Al–CeVO4 |
0.0 |
52.14 ± 5.82 |
8.69 |
Au0.2@Al–CeVO4 |
0.2 |
90.71 ± 9.07 |
15.11 |
Au0.4@Al–CeVO4 |
0.4 |
129.86 ± 9.98 |
21.64 |
Au0.6@Al–CeVO4 |
0.6 |
182.71 ± 9.83 |
30.45 |
Au0.8@Al–CeVO4 |
0.8 |
252.93 ± 10.64 |
42.15 |
Au1.0@Al–CeVO4 |
1.0 |
317.58 ± 11.87 |
52.93 |
Recyclability test
The stability and reusability of photocatalysts after the photoreaction were determined by a recyclability test.54 The most active Au1.0@Al–CeVO4 catalyst was used for this experiment, and three concordant runs of experiments were carried out (each run for 6 h). The results obtained from these experiments are illustrated in Fig. 7(d). After each run, a slight decrease in H2 evolution was observed, but the overall H2 evolution rate remained stable. The results obtained from the recyclability test revealed that our as-synthesized photocatalyst is stable and reusable. A slight decrease in the hydrogen production rate was attributed to the loss of photocatalyst during the recovery process and aggregation of catalyst particles that reduces the penetration of light.55
Photocatalytic mechanism
The band gap of semiconductor material CeVO4 was determined from UV-vis absorption spectra.56 The band gap is an important factor that affects the photocatalytic activity of catalysts. The band gap value of CeVO4 is 2.87 eV which is suitable to drive a photocatalytic reaction under solar light. Under sunlight irradiation, Au@Al–CeVO4 absorbs photons and generates electrons and holes in the conduction band and valence band of the semiconductor, respectively.57–59 The photogenerated electrons in the conduction band of the semiconductor react with water molecules adsorbed onto their active sites to form hydroxyl radicals (˙OH) and hydrogen ions (H+). These produced (H+) ions are converted into H2 as a result of a catalytic reduction reaction at active centres of the catalyst.60,61 Hydroxyl radicals react with water molecules to form additional hydrogen ions and molecular oxygen (O2). Conversely, the photogenerated holes in the valence band of CeVO4 react with water molecules to form hydroxyl radicals. Holes created in the valence band are consumed by sacrificial reagents and converted into an oxidized product as a result of photocatalytic oxidation,62,63 and the mechanism is shown in Scheme 1 and Fig. 8. Au metal electrons transfer to active sites by localized surface plasmon resonance (LSPR)64–66 and reduce H+ ions into hydrogen (H2). Al particles present in CeVO4 are electron deficient and have electron quenching ability due to which the population of electrons on active centres increases. Al doping promotes the transfer of electrons to active centres, which results in greater generation of hydrogen.
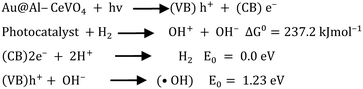 |
| Scheme 1 | |
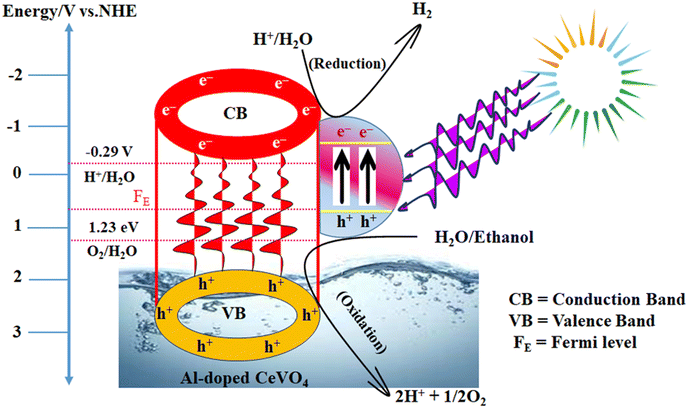 |
| Fig. 8 Mechanism of photocatalytic water splitting reaction. | |
Role of sacrificial reagent
When a photocatalyst absorbs light, it generates charges (e−/h+).67 Further, these charges tend to recombine with each other and inhibit the activity of the photocatalyst.68 To prevent the recombination of these charges, sacrificial reagents were used in the photocatalytic reaction mixture.69 Methanol, ethanol, lactic acid, EDTA, Na2S and cyanide can be used as sacrificial reagents.70 We used a 5% ethanol/water mixture for this purpose. Ethanol is a cost-effective and commercially available reagent. These sacrificial reagents scavenge the holes (h+), and as the holes are consumed by sacrificial reagents, the recombination of charges becomes less, and the photocatalytic activity of the catalyst is enhanced. These reagents after scavenging the holes are converted into oxidized products. Various amounts of sacrificial reagents were tested, and it was observed that 5% was the most effective for hydrogen generation (Table S1†). As we increased the concentration of sacrificial reagents, they occupy the surface of photocatalysts and inhibit the evolution of hydrogen.71
Factors influencing the activity of the photocatalyst
Various factors influence the activity of photocatalysts for hydrogen generation, such as temperature, pH, light and photocatalyst amount. The activity of the photocatalyst varies with variation in these factors. The conditions of the photocatalytic reaction change with variation in factors which cause variation in the activity of the photocatalyst. In this study, all these factors were optimized and fixed for maximum hydrogen generation from water splitting. The influence of these factors is given in the ESI.†
Conclusions
In conclusion, the study reported herein represents an excellent new approach for the production of renewable hydrogen from water using visible light as an irradiation source. In this study, all catalysts were hydrothermally prepared via a chemical reduction method. To evaluate the optical and structural properties, catalysts were characterized with XRD, SEM, AFM, UV-vis/DRS, BET, PL, EIS, and photocurrent studies. Using similar conditions, photoreactions were done in a quartz reactor (150 mL/Velp-UK), whereas hydrogen generation activities were monitored on a GC-TCD (Shimadazu-2010). Overall, 52.93 mmol g−1 h−1 of hydrogen was obtained on 5 mg of Au1.0@Al–CeVO4 catalyst that was found to be the most active catalyst of the series. The results show that the incorporation of Au and Al metal into CeVO4 not only promotes stability but also enhances catalytic performance during photoreaction. Higher activities were attributed to the contributions of LSPR electrons (of Au) during photoreaction. Conversely, due to its electron-deficient characteristics, Al enhanced the quenching of electrons to make an electron pool on semiconductor surfaces. Thus, the existence of higher localized electrons on CeVO4 favours higher proton reduction for hydrogen evolution. Additionally, the results of recyclability tests depict excellent stability and reusability for sustainable water splitting. On the basis of these results, it can be concluded that as-synthesised catalysts have the potential to replace costly and conventional catalysts used for hydrogen generation applications.
Conflicts of interest
Authors declare no conflict of interest.
Acknowledgements
This research work was funded by HEC of Pakistan with grant # 377/IPFP-II/Batch-I/SRGP/NAHE/HEC/2022/27 Islamabad. Catalysts preparation and hydrogen performances were done at Inorganic Materials Laboratory 52S, Institute of Chemistry, The Islamia University of Bahawalpur Pakistan. Dr. E. H. thanks to Carnegie Mellon University (USA) for instrumental access that is mandatory for the success of this work.
References
- Y. Horiuchi, T. Toyao, M. Takeuchi, M. Matsuoka and M. Anpo, Recent advances in visible-light-responsive photocatalysts for hydrogen production and solar energy conversion–from semiconducting TiO2 to MOF/PCP photocatalysts, Phys. Chem. Chem. Phys., 2013, 15, 13243–13253 RSC.
- T. Mao, B. Israelow, M. A. Peña-Hernández, A. Suberi, L. Zhou, S. Luyten, M. Reschke, H. Dong, R. J. Homer and W. M. Saltzman, Unadjuvanted intranasal spike vaccine elicits protective mucosal immunity against sarbecoviruses, Science, 2022, 378, eabo2523 CrossRef CAS PubMed.
- P. Hota, A. Das and D. K. Maiti, A short review on generation of green fuel hydrogen through water splitting, Int. J. Hydrogen Energy, 2023, 48, 523–541 CrossRef CAS.
- C. S. Gopinath and N. Nalajala, A scalable and thin film approach for solar hydrogen generation: a review on enhanced photocatalytic water splitting, J. Mater. Chem. A, 2021, 9, 1353–1371 RSC.
- S. G. Newman and K. F. Jensen, The role of flow in green chemistry and engineering, Green Chem., 2013, 15, 1456–1472 RSC.
- D. Hao, Y. Liu, S. Gao, H. Arandiyan, X. Bai, Q. Kong, W. Wei, P. K. Shen and B.-J. Ni, Emerging artificial nitrogen cycle processes through novel electrochemical and photochemical synthesis, Mater. Today, 2021, 46, 212–233 CrossRef CAS.
- S. G. Kumar and K. K. Rao, Comparison of modification strategies towards enhanced charge carrier separation and photocatalytic degradation activity of metal oxide semiconductors (TiO2, WO3 and ZnO), Appl. Surf. Sci., 2017, 391, 124–148 CrossRef CAS.
- J. Liu, Z. Luo, X. Mao, Y. Dong, L. Peng, D. Sun-Waterhouse, J. V. Kennedy and G. I. Waterhouse, Recent Advances in Self-Supported Semiconductor Heterojunction Nanoarrays as Efficient Photoanodes for Photoelectrochemical Water Splitting, Small, 2022, 18, 2204553 CrossRef CAS PubMed.
- A. Fujishima and K. Honda, Electrochemical Photolysis of Water at a Semiconductor Electrode, Nature, 1972, 238, 37–38 CrossRef CAS PubMed.
- A. Malathi, J. Madhavan, M. Ashokkumar and P. Arunachalam, A review on BiVO4 photocatalyst: activity enhancement methods for solar photocatalytic applications, Appl. Catal., A, 2018, 555, 47–74 CrossRef CAS.
- G. Bera, A. Mishra, P. Mal, A. Sankarakumar, P. Sen, A. Gangan, B. Chakraborty, V. Reddy, P. Das and G. Turpu, Multifunctionality of partially reduced graphene oxide–CrVO4 nanocomposite: electrochemical and photocatalytic studies with theoretical insight from density functional theory, J. Phys. Chem. C, 2018, 122, 21140–21150 CrossRef CAS.
- B. Ozturk and G. S. P. Soylu, Synthesis of surfactant-assisted FeVO4 nanostructure: characterization and photocatalytic degradation of phenol, J. Mol. Catal. A: Chem., 2015, 398, 65–71 CrossRef CAS.
- M. Z. Abid, K. Rafiq, A. Rauf, S. S. Ahmad Shah, R. Jin and E. Hussain, Synergism of Co/Na in BiVO4 microstructures for visible-light driven degradation of toxic dyes in water, Nanoscale Adv., 2023, 5, 3247–3259 RSC.
- M. Jalil, K. Rafiq, M. Z. Abid, A. Rauf, S. Wang, S. Iqbal and E. Hussain, Facile transfer of surface plasmon electrons of Au-NPs to Zn3V2O8 surfaces: a case study of sunlight driven H2 generation from water splitting, Nanoscale Adv., 2023, 5, 3233–3246 RSC.
- A. Ilyas, K. Rafiq, M. Z. Abid, A. Rauf and E. Hussain, Growth of villi-microstructured bismuth vanadate (Vm-BiVO4) for photocatalytic degradation of crystal violet dye, RSC Adv., 2023, 13, 2379–2391 RSC.
- U. Quyyum, K. Rafiq, M. Z. Abid, F. Ahmad, A. Rauf and E. Hussain, Tunable
sulphur doping in CuFe2O4 for the efficient removal of arsenic through arsenomolybdate complex adsorption: kinetics, isothermal and mechanistic studies, Environ. Sci.: Water Res. Technol., 2023, 9, 1147–1160 RSC.
- A. Aslam, M. Z. Abid, K. Rafiq, A. Rauf and E. Hussain, Tunable sulphur doping on CuFe2O4 nanostructures for the selective elimination of organic dyes from water, Sci. Rep., 2023, 13, 6306 CrossRef CAS PubMed.
- S. Ghotekar, S. Pansambal, K.-Y. A. Lin, D. Pore and R. Oza, Recent advances in synthesis of CeVO4 nanoparticles and their potential scaffold for photocatalytic applications, Top. Catal., 2023, 66, 89–103 CrossRef CAS.
- S. J. Moniz and J. Tang, Charge Transfer and Photocatalytic Activity in CuO/TiO2 Nanoparticle Heterojunctions Synthesised through a Rapid, One-Pot, Microwave Solvothermal Route, ChemCatChem, 2015, 7, 1659–1667 CrossRef CAS.
- Slamet, D. Tristantini, Valentina and M. Ibadurrohman, Photocatalytic hydrogen production from glycerol–water mixture over Pt-N-TiO2 nanotube photocatalyst, Int. J. Energy Res., 2013, 37, 1372–1381 CrossRef CAS.
- B. Akhsassi, A. Bouddouch, Y. Naciri, B. Bakiz, A. Taoufyq, C. Favotto, S. Villain, F. Guinneton and A. Benlhachemi, Enhanced photocatalytic activity of Zn3(PO4)2/ZnO composite semiconductor prepared by different methods, Chem. Phys. Lett., 2021, 783, 139046 CrossRef CAS.
- J. Jiang, X. Zhang, P. Sun and L. Zhang, ZnO/BiOI heterostructures: photoinduced charge-transfer property and enhanced visible-light photocatalytic activity, J. Phys. Chem. C, 2011, 115, 20555–20564 CrossRef CAS.
- M. Antoniadou, V. M. Daskalaki, N. Balis, D. I. Kondarides, C. Kordulis and P. Lianos, Photocatalysis and photoelectrocatalysis using (CdS-ZnS)/TiO2 combined photocatalysts, Appl. Catal., B, 2011, 107, 188–196 CrossRef CAS.
- H. Ge, F. Xu, B. Cheng, J. Yu and W. Ho, S-scheme heterojunction TiO2/CdS nanocomposite nanofiber as H2-production photocatalyst, ChemCatChem, 2019, 11, 6301–6309 CrossRef CAS.
- M. Sabir, K. Rafiq, M. Z. Abid, U. Quyyum, S. S. A. Shah, M. Faizan, A. Rauf, S. Iqbal and E. Hussain, Growth of tunable Au-BaO@TiO2/CdS heterostructures: Acceleration of hydrogen evolution from water splitting, Fuel, 2023, 353, 129196 CrossRef CAS.
- K. U. Sahar, K. Rafiq, M. Z. Abid, A. Rauf, U. ur Rehman, M. A. Nadeem, R. Jin and E. Hussain, Sun-light driven hydrogen generation by Pd/Rb2O cocatalysts: Escalate the utility of rutile TiO2 for photocatalytic water splitting, Colloids Surf., A, 2023, 131942 CrossRef CAS.
- F. Ahmad, K. Rafiq, T. Najam, E. Hussain, M. Sohail, M. Z. Abid, A. Mahmood, M. S. Javed and S. S. A. Shah, Metal-organic frameworks for electrocatalytic water-splitting: Beyond the pyrolysis, Int. J. Hydrogen Energy, 2023, 48(90), 35075–35111 CrossRef CAS.
- W. Wang, D. Wang, H. Song, D. Hao, B. Xu, J. Ren, M. Wang, C. Dai, Y. Wang and W. Liu, Size effect of gold nanoparticles in bimetallic ZIF catalysts for enhanced photo-redox reactions, Chem. Eng. J., 2023, 455, 140909 CrossRef CAS.
- D. Hao, J. Ren, Y. Wang, H. Arandiyan, M. Garbrecht, X. Bai, H. K. Shon, W. Wei and B.-J. Ni, A green synthesis of Ru modified g-C3N4 nanosheets for enhanced photocatalytic ammonia synthesis, Energy Mater. Adv., 2021, 2021, 9761263 Search PubMed.
- A. Shahzad, K. Rafiq, M. Zeeshan Abid, N. Ahmad Khan, S. Shoaib Ahmad Shah, R. H. Althomali, A. Rauf and E. Hussain, Escalating the synergism on CdZnS via Ag2S/Cu2S co-catalysts: Boosts hydrogen evolution from water splitting under sunlight, J. Catal., 2024, 429, 115210 CrossRef CAS.
- H. Noh, J. Lee, H. Ma, J. Shin, I. Roh, J. Yang and T. Yu, Synthesis of Pt-CeVO4 nanocomposites and their enhanced photocatalytic hydrogen evolution activity under sunlight, J. Ind. Eng. Chem., 2023, 125, 277–283 CrossRef CAS.
- Y. Shen, Y. Huang, S. Zheng, X. Guo, Z.-X. Chen, L. Peng and W. Ding, Nanocrystals of CeVO4 doped by metallic heteroions, Inorg. Chem., 2011, 50, 6189–6194 CrossRef CAS PubMed.
- S. Ahlawat and S. Lata, Electrochemical synthesis of graphene rooted with prepared cerium vanadate liberating GO@CeVO4 composite for super-capacitive energy reservoir, furthered with photocatalytic degradation of Rose Bengal dye, J. Energy Storage, 2023, 67, 107645 CrossRef.
- H. Liu, J. Guan, S. Yang, Y. Yu, R. Shao, Z. Zhang, M. Dou, F. Wang and Q. Xu, Metal–Organic-Framework-Derived Co2P Nanoparticle/Multi-Doped Porous Carbon as a Trifunctional Electrocatalyst, Adv. Mater., 2020, 32, 2003649 CrossRef CAS PubMed.
- G. Lu, B. Song, Z. Li, H. Liang and X. Zou, Photocatalytic degradation of naphthalene on CeVO4 nanoparticles under visible light, Chem. Eng. J., 2020, 402, 125645 CrossRef CAS.
- L. Sun, X. Ye, Z. Cao, C. Zhang, C. Yao, C. Ni and X. Li, Upconversion enhanced photocatalytic conversion of lignin biomass into valuable product over CeVO4/palygorskite nanocomposite: Effect of Gd3+ incorporation, Appl. Catal., A, 2022, 648, 118923 CrossRef CAS.
- L. Zhang, X. Hao, J. Li, Y. Wang and Z. Jin, Unique synergistic effects of ZIF-9 (Co)-derived cobalt phosphide and CeVO4 heterojunction for efficient hydrogen evolution, Chin. J. Catal., 2020, 41, 82–94 CrossRef CAS.
- Y. Yang, P. Gao, X. Ren, L. Sha, P. Yang, J. Zhang, Y. Chen and L. Yang, Massive Ti3+ self-doped by the injected electrons from external Pt and the efficient photocatalytic hydrogen production under visible-Light, Appl. Catal., B, 2017, 218, 751–757 CrossRef CAS.
- A. Reshak, Novel photocatalytic water splitting solar-to-hydrogen energy conversion: CdLa2S4 and CdLa2Se4 ternary semiconductor compounds, Phys. Chem. Chem. Phys., 2018, 20, 8848–8858 RSC.
- H. Liu, J. Yuan, Z. Jiang, W. Shangguan, H. Einaga and Y. Teraoka, Novel photocatalyst of V-based solid solutions for overall water splitting, J. Mater. Chem., 2011, 21, 16535–16543 RSC.
- Y. Yang, P. Gao, Y. Wang, L. Sha, X. Ren, J. Zhang, Y. Chen, T. Wu, P. Yang and X. Li, A direct charger transfer from interface to surface for the highly efficient spatial separation of electrons and holes: The construction of Ti–C bonded interfaces in TiO2-C composite as a touchstone for photocatalytic water splitting, Nano Energy, 2017, 33, 29–36 CrossRef CAS.
- S. K. Gahlaut, P. Devi and J. Singh, Self-sustainable and recyclable Ag nanorods for developing Ag-Ag2S nano heterostructures using sewage gas: Applications in photocatalytic water purification, hydrogen evolution, SERS and antibacterial activity, Appl. Surf. Sci., 2020, 528, 147037 CrossRef CAS.
- D. Pant, A. Singh, G. Van Bogaert, S. I. Olsen, P. S. Nigam, L. Diels and K. Vanbroekhoven, Bioelectrochemical systems (BES) for sustainable energy production and product recovery from organic wastes and industrial wastewaters, RSC Adv., 2012, 2, 1248–1263 RSC.
-
G. Song, A. Atrens and D. StJohn, An hydrogen evolution method for the estimation of the corrosion rate of magnesium alloys, in Essential Readings in Magnesium Technology, 2016, pp. 565–572 Search PubMed.
- H. D. Jang, S.-K. Kim and S.-J. Kim, Effect of particle size and phase composition of titanium dioxide nanoparticles on the photocatalytic properties, J. Nanopart. Res., 2001, 3, 141–147 CrossRef CAS.
- V. Panchal, S. López-Moreno, D. Santamaría-Pérez, D. Errandonea, F. Manjón, P. Rodríguez-Hernandez, A. Muñoz, S. Achary and A. Tyagi, Zircon to monazite phase transition in CeVO4: X-ray diffraction and Raman-scattering measurements, Phys. Rev. B: Condens. Matter Mater. Phys., 2011, 84, 024111 CrossRef.
- Z. Jin, H. Li, H. Gong, K. Yang and Q. Guo, Eosin Y-sensitized rose-like MoSx and CeVO4 construct a direct Z-scheme heterojunction for efficient photocatalytic hydrogen evolution, Catal. Sci. Technol., 2021, 11, 4749–4762 RSC.
- M. Hakimi and M. Alikhani, Characterization of α-Fe2O3 Nanoparticles Prepared from a New [Fe(Ofloxacin)2Cl2] Precursor: A Heterogeneous Photocatalyst for Removal of Methylene Blue and Ciprofloxacin in Water, J. Inorg. Organomet. Polym. Mater., 2020, 30, 504–512 CrossRef CAS.
- A. Garg, T. Singhania, A. Singh, S. Sharma, S. Rani, A. Neogy, S. R. Yadav, V. K. Sangal and N. Garg, Photocatalytic degradation of bisphenol-A using N, Co codoped TiO2 catalyst under solar light, Sci. Rep., 2019, 9, 765 CrossRef PubMed.
- M. A. Carreon, S. Y. Choi, M. Mamak, N. Chopra and G. A. Ozin, Pore architecture affects photocatalytic activity of periodic mesoporous nanocrystalline anatase thin films, J. Mater. Chem., 2007, 17, 82–89 RSC.
- D. A. Erdogan and E. Ozensoy, Hierarchical synthesis of corrugated photocatalytic TiO2 microsphere architectures on natural pollen surfaces, Appl. Surf. Sci., 2017, 403, 159–167 CrossRef CAS.
- G. Divya, G. Jaishree, T. Sivarao and K. D. Lakshmi, Microwave assisted sol–gel approach for Zr doped TiO2 as a benign photocatalyst for bismark brown red dye pollutant, RSC Adv., 2023, 13, 8692–8705 RSC.
- J. Li, G. Xie, J. Jiang, Y. Liu, C. Chen, W. Li, J. Huang, X. Luo, M. Xu and Q. Zhang, Enhancing photodegradation of Methyl Orange by coupling piezo-phototronic effect and localized surface plasmon resonance, Nano Energy, 2023, 108, 108234 CrossRef CAS.
- Q. Li, H. Zhang, Y. Yan, Z. Yang, Y. Wang, G. Liu and T. Ni, Tunable and sustainable photocatalytic activity of photochromic Y-WO3 under visible light irradiation, RSC Adv., 2021, 11, 1147–1152 RSC.
- M. Fereidooni, F. Esmaeilzadeh and A. Zandifar, Innovatively-synthesized CeO2/ZnO photocatalysts by sono-photochemical deposition: catalyst characterization and effect of operational parameters on high efficient dye removal, J. Mater. Sci., 2022, 57, 16228–16244 CrossRef CAS.
- M. Wang, Y. Guo, Z. Wang, H. Cui, T. Sun and Y. Tang, Simple glycerol-assisted and morphology controllable solvothermal synthesis of CeVO4/BiVO4 hierarchical hollow microspheres with enhanced photocatalytic activities, Mater. Chem. Front., 2021, 5, 6522–6529 RSC.
- H. Liu, P. Su, Z. Jin and Q. Guo, A sea-urchin-structured NiCo2O4 decorated Mn0.05Cd0.95S p–n heterojunction for enhanced photocatalytic hydrogen evolution, Dalton Trans., 2020, 49, 13393–13405 RSC.
- E. Hussain, I. Majeed, M. A. Nadeem, A. Badshah, Y. Chen, M. A. Nadeem and R. Jin, Titania-supported palladium/strontium nanoparticles (Pd/Sr-NPs@P25) for photocatalytic H2 production from water splitting, J. Phys. Chem. C, 2016, 120, 17205–17213 CrossRef CAS.
- K. U. Sahar, K. Rafiq, Z. Abid, U. ur Rehman, A. Rauf and E. Hussain, Surface sensitization of g-C3N4/TiO2 via Pd/Rb2O cocatalysts: Accelerating water splitting reaction for green fuel production in the absence of organic sacrificial agents, React. Chem. Eng., 2023, 8, 2522–2536 RSC.
- Y. Z. Voloshin, A. Belov, A. Vologzhanina, G. Aleksandrov, A. Dolganov, V. Novikov, O. Varzatskii and Y. N. Bubnov, Synthesis, structure, properties and immobilization on a gold surface of the monoribbed-functionalized tris-dioximate cobalt (II) clathrochelates and an electrocatalytic hydrogen production from H+ ions, Dalton Trans., 2012, 41, 6078–6093 RSC.
- F. Saleem, M. Z. Abid, K. Rafiq, A. Rauf, K. Ahmad, S. Iqbal, R. Jin and E. Hussain, Synergistic effect of Cu/Ni cocatalysts on CdS for sun-light driven hydrogen generation from water splitting, Int. J. Hydrogen Energy, 2023, 52(Part A), 305–319 Search PubMed.
- S. Iguchi, K. Teramura, S. Hosokawa and T. Tanaka, Effect of the chloride ion as a hole scavenger on the photocatalytic conversion of CO2 in an aqueous solution over Ni–Al layered double hydroxides, Phys. Chem. Chem. Phys., 2015, 17, 17995–18003 RSC.
- E. Hussain, I. Majeed, M. A. Nadeem, A. Iqbal, Y. Chen, M. Choucair, R. Jin and M. A. Nadeem, Remarkable effect of BaO on photocatalytic H2 evolution from water splitting via TiO2 (P25) supported palladium nanoparticles, J. Environ. Chem. Eng., 2019, 7, 102729 CrossRef CAS.
- Z. Abid, A. Ilyas, K. Rafiq, M. A. Nadeem, A. Rauf, A. Wasim and E. Hussain, Simultaneous elimination of toxic dyes, ciprofloxacin and Cr (VI) contents from polluted water: Escalating Surface plasmon electrons of Ag cocatalysts on BiVO4 microstructures†, Environ. Sci.: Water Res. Technol., 2023, 9, 2238–2252 RSC.
- H. Sheng, J. Wang, J. Huang, Z. Li, G. Ren, L. Zhang, L. Yu, M. Zhao, X. Li, G. Li, N. Wang, C. Shen and G. Lu, Strong synergy between gold nanoparticles and cobalt porphyrin induces highly efficient photocatalytic hydrogen evolution, Nat. Commun., 2023, 14, 1528 CrossRef CAS PubMed.
- N. Wajid, K. Rafiq, M. Z. Abid, A. Ilyas, T. Najam, A. Rauf and E. Hussain, Dual role of Au-NPs for Schottky effect and SPR electron injection over CdS surfaces for photocatalytic applications, Mater. Chem. Phys., 2023, 306, 128062 CrossRef CAS.
- A. Rahman and M. M. Khan, Chalcogenides as photocatalysts, New J. Chem., 2021, 45, 19622–19635 RSC.
- Y. Yan, M. Han, A. Konkin, T. Koppe, D. Wang, T. Andreu, G. Chen, U. Vetter, J. R. Morante and P. Schaaf, Slightly hydrogenated TiO2 with enhanced photocatalytic performance, J. Mater. Chem. A, 2014, 2, 12708–12716 RSC.
- A. A. Ismail and D. W. Bahnemann, Photochemical splitting of water for hydrogen production by photocatalysis: A review, Sol. Energy Mater. Sol. Cells, 2014, 128, 85–101 CrossRef CAS.
- F. Guzman, S. S. Chuang and C. Yang, Role of methanol sacrificing reagent in the photocatalytic evolution of hydrogen, Ind. Eng. Chem. Res., 2013, 52, 61–65 CrossRef CAS.
- X.-J. Lv, S.-X. Zhou, C. Zhang, H.-X. Chang, Y. Chen and W.-F. Fu, Synergetic effect of Cu and graphene as cocatalyst on TiO2 for enhanced photocatalytic hydrogen evolution from solar water splitting, J. Mater. Chem., 2012, 22, 18542–18549 RSC.
|
This journal is © The Royal Society of Chemistry 2024 |
Click here to see how this site uses Cookies. View our privacy policy here.