DOI:
10.1039/D4CY00820K
(Review Article)
Catal. Sci. Technol., 2024,
14, 6466-6495
Enhancing light-driven photocatalytic reactions through solid solutions of bismuth oxyhalide/bismuth rich photocatalysts: a systematic review
Received
2nd July 2024
, Accepted 1st October 2024
First published on 17th October 2024
Abstract
The pursuit of sustainable environmental remediation strategies has led to intensive research in photocatalysis. Photocatalysts are a class of compounds with exceptional properties, making them suitable for various applications in environmental remediation. They are effective against multiple organic and inorganic pollutants and are also used as semiconductors for green energy production. Among the various photocatalytic semiconductor materials explored, bismuth oxyhalide (BiOY, Y = F, Cl, Br, or I) and bismuth-rich (BixOyYz, Y = F, Cl, Br, or I) are particularly notable. However, unmodified/pristine BiOY and BixOyYz exhibit inherent limitations of low photocatalytic performance owing to unsuitable band gaps and low efficiency in separating carriers. Hence, this review outlines the BiOY and BixOyYz structures, wherein modifiable halogen layers may offer favorable conditions for creating solid solutions with improved intrinsic properties and catalytic performance. This systematic review also explores the unique attributes and challenges associated with tuning the photocatalytic performance of BiOY and BixOyYz solid solutions to enhance solar-driven reactions. The distinctive feature of this review lies in the versatile nature of the BiOY and BixOyYz solid solution materials. These materials offer the advantage of harnessing a broad light spectrum, including solar and UV radiation. This review further explores the strategies and techniques employed to optimize BiOY and BixOyYz photocatalysts by forming solid solution and their application in water treatment processes. Furthermore, it highlights the ongoing challenges and opportunities in developing high-performance BiOY and BixOyYz solid solution photocatalysts, illustrating their potential to drive a more sustainable and energy-efficient future through enhanced light-driven reactions.
1. Introduction
The worldwide population explosion, technological advancement increase, environmental degradation, and rising energy requirements have presented humanity with profound challenges, including energy deficits, ecological contamination, and climate change.1 Environmental contamination resulting from volatile organic compounds, heavy metals, polymer residues, microplastics, and biological pollutants has become a substantial socioeconomic issue stemming from industrialization and urbanization.2,3 Ecosystem function and public health may be profoundly imperiled by the presence of organic xenobiotics in the environment, which can be very harmful, even at trace concentrations. These organic compounds cannot be completely removed from environmental matrices via conventional contaminant remediation methodologies, such as filtration, precipitation, adsorption, and centrifugation. These methods transform these organic compounds into solid sludge rather than breaking them entirely.4
On the other hand, the finite reserves of carbonaceous fossil fuel feedstocks are being rapidly depleted due to the continuous growth of the global human population, rendering energy production one of the preeminent challenges that humanity will confront in the proximate future. Consequently, the paramount issue of how to employ scientific methodologies to resolve these multifaceted issues and ensure the sustainable development of human civilization emerges. The exploration and harnessing of sustainable, renewable energy modalities, such as wind, tidal, solar power, and ocean thermal, garner substantial interest and investment. Therefore, developing efficient and environmentally benign techniques and technologies is paramount. Renewable energy sources should power these methods and aim to transform harmful contaminants into harmless substances without causing secondary pollution.5
Among the most valuable gifts bestowed by nature is the abundant and clean source of solar light that stands out as an unparalleled source of thermal and non-thermal energy. Semiconductor photocatalysis, a viable technology for harnessing solar energy, holds immense promise for various applications, including environmental remediation, energy generation, organic synthesis, and medical treatments.6 The field of semiconductor photocatalysis was inaugurated by pioneering studies conducted in 1972, which demonstrated the process of photolytic water decomposition using titanium dioxide (TiO2) anodes under ultraviolet illumination.7 Since then, this field has entered a substantial growth phase marked by significant advancements in developing efficient photocatalysts. Notably, a variety of materials, such as metallic oxides (e.g., TiO2, ZnO, Ta2O5, Fe2O3), nitrides (e.g., Ta3N5, TaON), and sulfides (e.g., CdS, MoS2), have been extensively studied and explored for their photocatalytic properties.8–11
To date, this has sparked widespread enthusiasm for intensive exploration in developing high-performance semiconductor photocatalysts, aiming to mitigate the energy crisis and address environmental challenges. Bismuth-based photocatalysts with layered structures have attracted substantial research attention in photocatalysis. This heightened interest stems from their distinctive characteristics, including a layered crystal structure, diverse compositions, intricate atomic coordination, and an enticing hybrid electronic band structure. These qualities provide a versatile platform for crystal structure design, control over morphology and facets, band structure regulation, defect formation, and a host of other modifications to enhance solar energy conversion efficiency.12,13
Some reviews have documented the use of bismuth-based photocatalysts in diverse photocatalytic light-driven processes.14–21 These reviews have detailed various modification methodologies, including oxygen vacancies14,15 ternary nanocomposites16,17 manipulation of crystal vacancy defects,18 establishment of heterojunctions, employing sensitizers, consideration of facet effects, regulation of microstructure, incorporation of surface plasmonic resonance, implementation of strain engineering, control of morphology, and elemental doping.19–21 However, to the best of our knowledge, there is no reported review dedicated exclusively to the tuning/modification of BiOY and BixOyYz photocatalyst materials through the formation of solid solutions. Therefore, this review highlights enhancing BiOY and BixOyYz photocatalysts through solid solution tuning for better practical use in diverse photocatalytic processes, exploring structural customization's impact on efficacy. In addition, it reports the strategies for improving the intrinsic properties of BiOY and BixOyYz solid solutions. Furthermore, it highlights the ongoing challenges and opportunities in the quest for high-performance photocatalysts, illustrating their potential to drive a more sustainable and energy-efficient future through enhanced light-driven reactions (LDR).
1.1. Structures of BiOY and BixOyYz materials
Several diverse bismuth-based photocatalysts including bismuth oxyhalides (BiOY),22 bismuth vanadate (BiVO4),23 bismuth titanates (BixTiyOz),24 bismuth tungstate (Bi2WO6),25 bismuth oxycarbonates (Bi2O2CO3),26 bismuth silicates (BixSiyOz),27 bismuth ferrites (BiFeO3),28 bismuth molybdates (Bi2MoO6),29 bismuth iodates (Bi(IO3)3),30 bismuth niobites (BiNbO4),31 bismuth phosphate (BiPO4),32 have been fabricated. This range of materials benefits from the orbital hybridization between Bi 6s in Bi(III) and O 2p, resulting in a broader hybridized state. This, in turn, yields a narrower bandgap for improved photoabsorption and highly dispersive band structures, facilitating rapid charge migration.33,34 Bismuth oxyhalide, a remarkable photocatalyst with a layered structure containing [Y–Bi–O–Bi–Y] slabs interspersed by dual-halogen atom slabs, is a Bi-based photocatalyst that has gained significant attention globally.35
Several notable and intriguing findings have emerged from studies on BiOY photocatalysts. The internal static electric field within this multilayered structure (BiOY photocatalysts) has been found to facilitate the separation of electron–hole pairs, thereby enhancing photocatalytic performance.28,29 The layers composed of [Y–Bi–O–Bi–Y] joined by van der Waals forces to create a tetragonal matlockite crystal lattice,36 as illustrated in Fig. 1. Historically, BiOY substances have been used extensively as storage and ferroelectric materials, catalysts, and pigments. Nevertheless, in recent years, novel applications have been discovered across diverse domains, encompassing CO2 photoreduction,37–39 photocatalytic cleansing of wastewater,40–43 water splitting,44 alcohol oxidation, and organic synthesis.45–47
 |
| Fig. 1 Structure of BiOY systems featuring Y–Bi–O–Bi–Y slabs arranged in a stoichiometric manner along the (a) a, (b) b, (c) c-axis, and (d) standard orientation of crystal shape. | |
The considerable charge transfer dynamics resulting from the indirect bandgap characteristics of these semiconductors, in conjunction with their well-defined crystalline structures, render these materials highly promising candidates for H2 production applications. Furthermore, the induction of a perpendicular internal electric field across the (001) crystallographic plane of BiOY photocatalysts can facilitate enhanced charge carrier separation along this preferred orientation.48 A key advantage of BiOY materials is their broad range of tunable bandgap energies, spanning from 3.3 eV for BiOCl to 1.8 eV for BiOI. This wide bandgap tunability enables the utilization of these photocatalysts under visible light illumination.49,50 Since Zhang et al. 2006 (ref. 51) documented the production of three-dimensional microspheres consisting of BiOY on two-dimensional nanoplates using solvothermal synthesis to degrade methyl orange, many researchers have focused on investigating the photocatalytic capabilities of BiOY materials.43,52–56
1.2. Properties of BiOY and BixOyYz materials
Compared to the BiOY class of photocatalysts, the BixOyYz materials also exhibit a layered crystal structure characterized by robust interlayer covalent bonding and weak interlayer non-bonding van der Waals interactions. Taking Bi4O5Br2 as an illustrative example, Fig. 2 provides a schematic representation of the crystallographic arrangement of this representative BixOyYz compound. The crystal structure of Bi4O5Br2 is composed of [Bi–O] layers positioned between two bromine ion slabs, resulting in a distinctly layered architectural motif. Interestingly, the charge density surrounding the [Bi–O] layers is found to be greater than that of the adjacent double bromine slabs in the Bi4O5Br2 structure. This non-uniform charge distribution between the [Bi–O] and double bromine layers induces a static internal electric field (IEF) within the material, which can facilitate the efficient separation of photogenerated electron–hole pairs.57–59
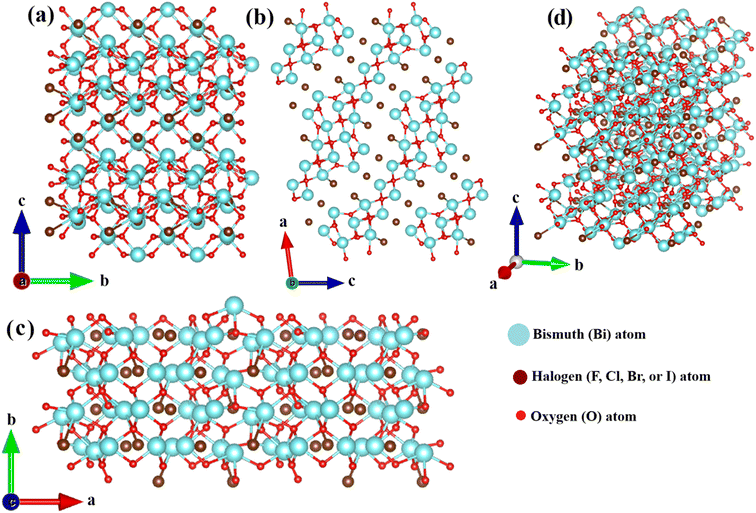 |
| Fig. 2 The structural model of Bi4O5Br2 along (a) a, (b) b, (c) c-axis, and (d) standard orientation of crystal shape. | |
The directional growth of the layered Bi4O5Br2 aligns with the [101] direction, leading to an internal electric field direction in the [101] orientation, which deviates from the [001] direction observed in BiOY. Density functional theory (DFT) calculations and empirical findings have consistently demonstrated the increased photon absorption efficiency of BixOyYz. This observation was corroborated by previous studies.60,61 Facilitating charge carrier separation efficiency is a critical factor in optimizing the performance of semiconductor-based photocatalytic systems. In the case of BixOyYz photocatalysts, the primary mechanism driving the efficacy of charge separation is the intrinsic internal electric field situated between its distinctive layered structural layers.57
Generally, for semiconductor-based photocatalytic systems, increased internal electric field intensity typically corresponds to enhanced efficiency in the spatial separation of photogenerated charge carriers. The strength of this internal electric field is determined by the magnitude of the polarization space and the material's polarization force. The substantial interlayer spacing and pronounced dipole moment characteristics inherent to the layered BixOyYz crystal structure contribute to these materials' improved polarization space and polarization force. Furthermore, increasing the ratio of Bi to the halogen element (X) not only boosts the optical absorption capabilities but also fortifies the hybridization of the conduction band, thereby facilitating electron migration and promoting the spatial separation of photogenerated electron–hole pairs.58 As a result of these advantageous structural and electronic features, the BixOyYz class of photocatalytic materials has demonstrated outstanding performance in a wide range of applications. Since the initial report of Bi3O4Cl photocatalysts by Lin et al.62 in 2006, a series of additional BixOyYz photocatalysts, including Bi24O31Cl10, Bi12O17Cl2, Bi12O15Cl6, Bi3O4Cl, Bi3O4Br, Bi12O17Br2, Bi24O31Br10, Bi4O5Br2, Bi5O7Br, Bi4O5I2, Bi7O9I3, and Bi5O7I, have been extensively studied and documented in the review by Jin et al.63 The tunable bandgap energies of BixOyYz nanocrystals, spanning from 2.0 to 3.6 eV, enable their efficient utilization under a broad range of illumination conditions, including the UV-visible light spectrum.63 However, further improvements in the properties and performance of both BiOY and BixOyYz photocatalyst materials will be necessary to facilitate their widespread commercial and industrial adoption.
2. Fabrication of BiOY and BixOyYz photocatalytic material
The methodologies employed for synthesis significantly contribute to the effective fabrication of nano- and micro-structures in accordance with specific requirements. The pivotal factors governing the production of BiOY nanomaterials are enhanced through facile synthesis. Recently, several techniques have been established for the synthesis of BiOY and its composites by using hydrolysis, hydrothermal, ion exchange, co-precipitation, deposition–precipitation, photodeposition, thermal reduction, direct combination, electrostatic self-assembly, template-assisted, and microwave-assisted methods.64 As advancements in traditional BiOY continue, bismuth-rich and oxyhalides exhibit discernible differences in local atomic structure, electronic configuration, optical properties, and electrical conductivity compared to BiOY. Consequently, the physicochemical attributes and photocatalytic behavior can be effectively tailored. Given this context, it is crucial to summarize65–67 effective strategies for the controlled synthesis of BixOyYz and delve into the intrinsic mechanisms governing its formation processes. The preparation of BixOyYz materials through a high-temperature solid-state reaction is straightforward. This process involves combining precursors with specific elemental stoichiometric ratios subjected to elevated temperatures to yield the desired components of BixOyYz, as shown in Fig. 3. For instance, the synthesis of Bi3O4Cl entails blending Bi2O3 and BiOCl powders in a stoichiometric proportion, followed by calcination at 700 °C in an air environment for 24 h.62
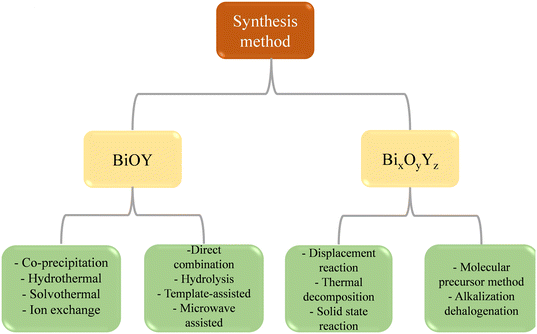 |
| Fig. 3 Various synthetic techniques to produce BiOY and Bi-rich BixOyYz materials. | |
3. Recent advances in characterization techniques
Significant progress has been made in characterization approaches for BiOY and BixOyYz materials in recent studies, especially in understanding their structural, electrical, and catalytic properties. These materials are becoming more and more popular because of their possible uses in electrical devices, photocatalysis, and energy storage.21 The combination of machine learning and conventional characterization methods, such as X-ray absorption spectroscopy, is one of the major developments. By speeding up the study of sophisticated materials like BiOY and BixOyYz, this method makes it possible to forecast chemical speciation and electrical structure with more accuracy-predictions that are essential for maximizing the material's performance in a variety of applications.63
Furthermore, studies have concentrated on the synthesis and mechanistic comprehension of these substances, particularly concerning their stability and effectiveness in energy-related uses. For example, new synthesis techniques and sophisticated characterization instruments are being used to produce materials that are more stable in real-world settings. Applications in hydrogen storage and transformation, where the stability and effectiveness of materials like BiOY and BiOY and BixOyYz are crucial, demand for this in particular.68 Overall, the practical application of BiOY and BixOyYz materials in next-generation technologies is being made possible by the combination of cutting-edge characterization techniques and creative synthesis approaches.
Structural characterization is achieved through X-ray diffraction (XRD), which provides insights into crystallite size and phase purity, while scanning electron microscopy (SEM) and transmission electron microscopy (TEM) reveal surface and internal morphology, respectively. Compositional analysis is performed using energy dispersive X-ray spectroscopy (EDS/EDX) and X-ray photoelectron spectroscopy (XPS), which detail elemental composition and surface chemical states. Raman spectroscopy (RS) and Fourier transform infrared spectroscopy (FTIR) offer information on bonding and molecular vibrations. Optical and electronic properties are explored with UV-visible spectroscopy and electrical conductivity measurements. Finally, Brunauer–Emmett–Teller (BET) surface area analysis and thermogravimetric nalysis (TGA) assess surface area and thermal stability, respectively. These techniques collectively provide a comprehensive understanding of the materials' characteristics, as summarized in Fig. 4.
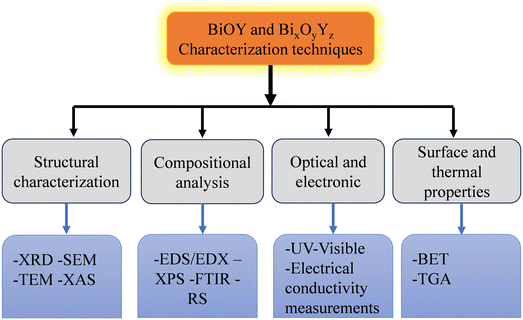 |
| Fig. 4 Overview of characterization techniques for BiOY and BixOyYz compounds. | |
4. BiOY and BixOyYz modification strategies
The photon absorption efficiency and carrier separation are critical challenges for semiconductor photocatalysts. Despite the strong visible-light photocatalytic properties exhibited by BiOY and BixOyYz materials, their practical application is hindered by their low overall photocatalytic efficiency, primarily attributed to photo-induced carriers' rapid recombination. Additionally, three common types of BiOY (BiOCl, BiOBr, and BiOI) respond differently to visible light. Therefore, to optimize the solar energy utilization of BiOY and BixOyYz in various photocatalytic light-driven processes, photocatalysts must exhibit a robust response to visible light, which constitutes a higher percentage of solar irradiation. This photocatalytic enhancement has been achieved through various modification strategies, such as coupling, doping, cocatalyst usage, and solid solutions, to improve charge separation.63,69
Various strategies, as depicted in Fig. 5, have been comprehensively reported in different reviews.14–21 Creating solid solutions is a promising avenue among the various strategies explored to enhance the properties of BiOY and BixOYz photocatalysts. Therefore, the present review provides a novel contribution to the current body of knowledge by elucidating the intricate details of solid solutions. This topic has yet to be extensively explored in existing literature. This review exclusively reports methods used to enhance the photocatalytic performance of BiOY and BixOyYz solid solutions materials and their application in the degradation of various water contaminants.
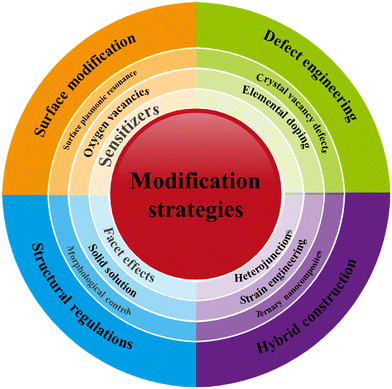 |
| Fig. 5 Classification of proposed tuning strategies for BiOY and Bi-rich BixOyYz materials. | |
4.1. Solid solutions
Solid solutions, which are homogeneous crystalline structures formed by the dissolution of two or more crystalline phases, can be created under solid-state conditions and using wet chemistry methods. Unlike a mere physical mixture of solid powders, a solid solution retains a singular phase, with constituent elements hybridizing at the atomic level. The structures and properties of these solid solutions can be altered by varying their constituent components, offering the potential for tailored electronic properties. For example, in certain cases, the band gap of a solid solution can be narrower than that of the individual components, making them highly attractive for applications requiring precise electronic characteristics.70 Solid solutions have been successfully applied across various domains, serving as electrocatalysts and photocatalysts with exceptional activity, selectivity, and stability as catalyst substrates. Examples include well-known materials like gallium arsenide (GaAs), gallium phosphide (GaP), aluminum arsenide (AlAs), and indium arsenide (InAs), where the selection of component solids is based on the desired characteristics of the resulting solid solution.71
In the field of photocatalysis, the construction of solid solutions from bismuth oxyhalides (BiOX, where X = Cl, Br, and I) and bismuth-rich compounds (BixOyYz) has garnered significant attention. The halogen atoms in BiOX vary, leading to unique band gaps in these materials. By producing solid solutions, such as BiOClxBry and BiOBrxIy, the band gaps can be precisely controlled to absorb a broader range of the solar spectrum, particularly extending into the visible light region. This redshifting of the absorption edge, which occurs when halides are mixed, enables the photocatalyst to harness more visible light, a crucial factor for solar-powered applications like pollution degradation and water splitting.72,73
The photocatalytic activity of these solid solutions can be finely tuned by adjusting the ratios of the Cl−, Br−, and I− ion components. BiOY (where Y = Cl, Br, I) displays different photo responses due to the distinct band gaps of BiOCl (3.3 eV), BiOBr (2.7 eV), and BiOI (1.8 eV). Because of their similar structural characteristics and atomic arrangements, Y can be arbitrarily adjusted to form BiOX–BiOY (X, Y = Cl, Br, I, and X + Y = 1) solid solutions (Fig. 6a), allowing for precise regulation of the photo response and optimizing the photocatalytic activity.74 This construction of solid solutions from BiOY and BixOyYz has garnered considerable attention over the years, as shown in Fig. 6b.74
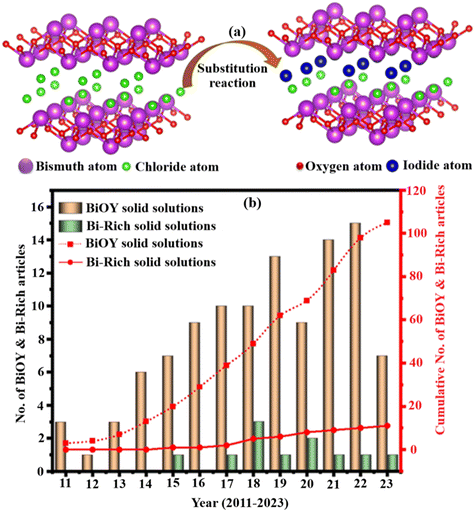 |
| Fig. 6 a) Formation mechanism of BiOY and BixOyYz solid solutions and b) the reported number of research articles on the modification of BiOY and BixOyYz into solid solutions (Sources Web of Science, 2011–2023). | |
Moreover, solid solutions offer a multidimensional strategy for enhancing photocatalytic performance by adjusting band gaps, improving charge carrier dynamics, introducing beneficial imperfections, and increasing structural stability. The creation of heterojunctions within solid solutions, which serve as recombination barriers, facilitates the separation of photogenerated electron–hole pairs, thereby increasing the efficiency of photocatalytic reactions.75 These advantages make solid solutions a robust and adaptable platform for photocatalysis, particularly in applications where high efficiency and long-term stability under sunlight are essential.76
4.1.1. Bismuth oxyhalide solid solution.
Recent progress in solid solution synthesis represents a substantial advancement in bandgap engineering, facilitating the customization of compounds to enhance the sensitization of photocatalysts in the visible light region. This engineering achievement allows for the narrowing of the bandgaps by either lowering the conduction band (CB) level or raising the valence band (VB) level.77 For instance, BiOY solid solutions involve the partial substitution of halogen atoms in a supercell with other exogenous halogen atoms. Owing to their electronic structure and atomic arrangement, halogen atoms can readily replace each other within the BiOY layered structure, allowing for a nearly arbitrary variation of halogen ions.78 Currently, various BiOY solid solutions compositions, such as, BiOIxCl1−x, BiOClxBryIz BiOClxBr1−x, BiO(OH)xI1−x, BiO(ClBr)(1−x)/2Ix, and BiOBrxI1−x have been synthesized and demonstrated to exhibit enhanced activity compared to their pure counterparts. Zhang et al. proposed that the heightened photocatalytic activity of BiOY solid solutions is contingent upon the modulation of band gaps. DFT computations have revealed that band gaps decrease with increasing concentrations of heavier halogen atoms, resulting in a substantial red shift of the adsorption spectra and greater utilization of sunlight.79 The variation of EV (vs. NHE) with the composition, x, which is based on the theoretical and experimental band gaps, is shown in Fig. 7. Furthermore, the VB constitution for BiOY comprises primary O 2p and X np orbitals,54 leading to more positively charged VB tops with an increase in lighter halogens, indicating an enhanced oxidation potential.79,80
 |
| Fig. 7 Variation in Ev (vs. NHE) against composition of x for the a) computed and b) experimental band gaps of BiOY solid solutions. Reprinted with permission from ref. 79. Copyright (2012) Royal Society of Chemistry. | |
BiOY exhibits remarkable electron–hole pair separation, a crucial characteristic shared among these materials.81 This phenomenon arises from the hybridization of X-np states, resulting in a reduced valence band maximum (VBM) and increased chemical disorder within the alloyed BiOY structure. The introduction of disorder and variation in VBM generates defect levels serve as trap centers for photogenerated holes. Consequently, this impedes the mobility of the photoexcited holes within the alloyed BiOX, thereby reducing electron–hole recombination. Wang et al.82 demonstrated that BiOBr0.85I0.15, rich in oxygen vacancies (OVs), exhibited the highest photocurrent density and the smallest radius in the electrochemical impedance spectra compared to other compositions. This finding suggests a prolonged lifetime of the photoinduced charge carriers in BiOBr0.85I0.15. Essentially, the formation of a solid solution in BiOY results in a reduction of the e−/h+ recombination rate, consequently enhancing the photocatalytic activity. Moreover, BiOY solid solutions can easily be tailored to form OVs, which act as capture traps for photogenerated electrons, further facilitating the separation of charge carriers.
A new family of BiO(ClxBr1−x) solid solutions synthesized via the hydrothermal method exhibited a 3 times increase in the rate of aqueous RhB removal under visible-light irradiation compared to Degussa P25. Some studies have reported the correlation between various experimental parameters and the characteristics of BiOY solid solutions. For instance, BiOCl0.875Br0.125 synthesized through a simple precipitation method at room temperature, involved the complete dissolution of Bi(NO3)3·5H2O in glacial acetic acid to yield [Bi2O2]2+ intermediate species. Glacial acetic acid facilitated the reaction between Bi3+, halide anions, and water. Cetyltrimethylammonium chloride (CTAC) and cetyltrimethylammonium bromide (CTAB) are common structure-directing agents and halide sources.83 Yang et al. conducted studies on various BiOClxBr1−x compositions to investigate the influence of the raw materials and solvents. Their findings revealed that NH4Cl, KBr, and water favored the formation of simple stacked nanoplates, whereas polyacrylamide (C-PAM), CTAB, and glycol promoted the formation of 3D hierarchical flower-like microspheres. In principle, the formed BiOY hierarchical flower-like structure possesses a larger specific surface area and an increased number of active sites, thereby enhancing photocatalytic reactions.84 These studies established the significant impact of fabrication methods and the inherent properties of source materials on the photocatalytic efficiency of BiOY solid solutions.
4.1.2. Bismuth-rich solid solution.
The comparable atomic configurations of BixOyXz, such as Bi3O4Br and Bi3O4Cl, facilitate the incorporation of various halogens into the crystal structure, substituting other halogens and forming solid solutions. This substitution leads to significant changes in the electronic structure of the resultant solid solutions, accompanied by variations in their atomic arrangements.85 In addition to adjusting the band edge positions, variations in the electrostatic potential between the [Bi–O] and [X] layers can be induced by the non-uniform charge distribution, allowing for the modulation of the internal electric field. For instance, Sun et al. synthesized a range of Bi4O5BrxI2x solid solutions using a simple homogeneous precipitation method, including Bi4O5Br2, Bi4O5–Br1.8I0.2, Bi4O5Br1.4I0.6, Bi4O5BrI, Bi4O5Br0.6I1.4, Bi4O5Br0.2I1.8, and Bi4O5I2.86
These solid solutions exhibited gradually adjustable band gap structures, as determined by UV-vis diffuse reflectance spectra, with Bi4O5Br0.6I1.4 demonstrating optimal photocatalytic activity for resorcinol degradation, which was 1.80 and 2.77 times higher than Bi4O5I2 and Bi4O5Br2, respectively. The enhanced photocatalytic performance of Bi4O5Br0.6I1.4 is attributed to the improved charge separation efficiency resulting from the formation of an internal electric field within the Bi4O5Y2 solid solutions, which polarizes the photogenerated charges, thereby enhancing the photocatalytic activity. Substituting I with Br in Bi4O5Br0.6I1.4 leads to a more non-uniform charge distribution, further optimizing the charge separation and increasing the photocatalytic activity. Liquid chromatography-mass spectrometry (LC-MS) analysis identified the degradation intermediates and proposed a potential degradation pathway for resorcinol. Other solid solutions, such as Bi5O7Br0.5I0.5 and Bi24O31ClxBr10−x, have also been synthesized and applied for photocatalytic pollutant removal, demonstrating the effectiveness of solid solution preparation in optimizing the photocatalytic performance.87,88
4.2. Influence of constructing solid solutions on the intrinsic properties of the material
The construction of solid solutions exerts a profound influence on the material's intrinsic properties, impacting factors such as the band structure, electronic properties, and charge carrier dynamics. By introducing foreign atoms into the crystal lattice, solid solutions alter the atomic arrangement and electronic configuration, leading to modifications in the band-edge positions and band-gap energies. Moreover, forming solid solutions can induce variations in the charge distribution within the material, affecting the polarization of photogenerated charges and enhancing the charge separation efficiency. These alterations in intrinsic properties play a pivotal role in dictating the photocatalytic performance of the material, making solid solution formation a promising strategy for optimizing the photocatalytic activity.89
4.2.1. Modulation of energy band structure.
Unlike conventional doping, solid solution formation can introduce novel impurity levels, broadening the light absorption spectrum and augmenting the photocatalytic efficiency.51 Different studies have explored the impact of different solid-solution compositions on the energy band structure of BiOY90–96 Xie et al.90 revealed that by adjusting the Cl/I ratio, there is a systematic shift in the VB position, consequently altering the bandgap and optimizing the light absorption efficiency. With an increase in the I content, the VB position of BiOClxI1−x moves upward, reducing the band gap and widening the visible light absorption range.
Some studies have employed theoretical calculation approaches to investigate the influence of solid solution formation on energy bands. Al-Keisy et al.91 examined the energy band structure of BiOI0.7F0.3 and BiOI, revealing a notable increase in the conduction band minimum (CBM) at point Z (Fig. 8a) induced by I/F solid solution formation. Zhang et al.92 elucidated the role of the Cl/I solid solution in modifying the energy-band structure, highlighting the impact of the Cl/I alloy on the top of the valence band (VB) of I0.25-BiOCl. Guo et al.93 prepared different nanostructured solid solutions, including nanosheets, nanoplates, and microspheres, according to the schematic illustrated in Fig. 8b. The band structures of the three nanostructured solid solutions (BiOBr0.7Cl0.3, BiOBr0.7Cl0.3, and BiOBr0.7Cl0.3) are shown in Fig. 8c. Changing the solvent, that is, using water or ethylene glycol, could effectively regulate the bandgap (Eg), conduction band energy (ECB), and valence band energy (EVB) of the samples.
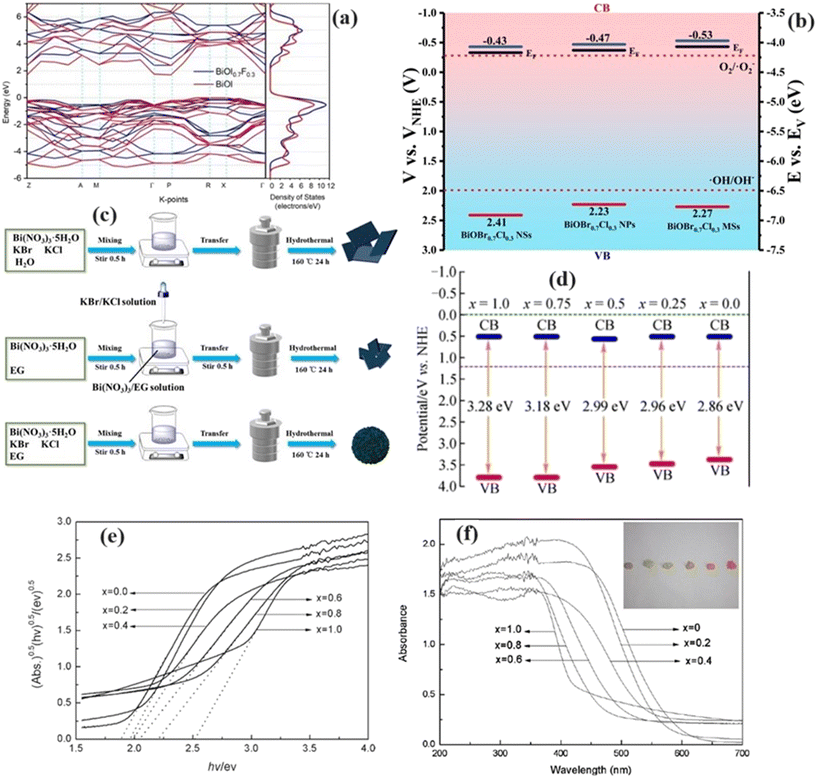 |
| Fig. 8 a) Band structure of BiOI0.7F0.3 and BiOI according to DFT calculations. Reprinted with permission from ref. 91. Copyright (2020) John Wiley and Sons; b) band structures of nanosheets, nanoplates, and microspheres nanostructures, c) schematic illustrations of the preparation. Reprinted with permission from ref. 93. Copyright (2022) Elsevier; d) band structures of BiOClxBr1−x solid solution. Reprinted with permission from ref. 94. Copyright (2017) Springer Nature; e) band gaps, f) UV-vis diffuse reflectance spectra BiOBrxI1−x, with color variation shown in the inset. Reprinted with permission from ref. 95. Copyright (2017) American Chemical Society. | |
The experimental determination of the band gaps of solid solution-based binary halogen systems, such as BiOClxBr1−x,94 BiOBrxI1−x,95 and BiOClxIy,96 confirms the theoretical predictions, demonstrating the effectiveness of solid solution engineering in continuously regulating the band gap. The construction of solid solutions also alters the light absorption capacity of the catalyst by modifying the band energy structure. With increasing Br content in BiOClxBr1−x, the bandgap gradually decreased (Fig. 8d), enhancing the light absorption ability of the catalyst and thus improving its photocatalytic activity. Similarly, as the x value decreased in BiOBrxI1–x, the band gap decreased (Fig. 8e), resulting in darker coloration and enhanced absorption of visible light (Fig. 8f). These findings confirm that solid solution engineering can adjust the bandgap width, thereby expanding the range of visible light absorption, akin to the behavior observed in binary halogen solid solutions.
4.2.2. Regulation of photogenerated charge carriers.
During solid-solution synthesis, structural imperfections can emerge within the crystalline lattice, acting as capture sites for photogenerated charge carriers, thereby influencing their dynamics. The disparity in mobility between different charge carriers plays a crucial role in reducing the recombination rates of photogenerated electron–hole pairs. Wang et al.82 demonstrated that the formation of a solid solution in BiOBrxI1−x leads to the hybridization of halogen np orbitals, which diminishes the mobility of photogenerated holes. In contrast, the migration of photogenerated electrons remains unaffected. This differential mobility between electrons and holes significantly lowers the carrier recombination rate.
The transfer pathway is crucial in determining the behavior of photogenerated charge carriers. Huang et al.97 employed a novel method to reduce photogenerated carrier recombination by increasing the band gap through solid-solution formation. By incorporating bromine into BiOI, the valence band (VB) position shifts downward, lengthening the electron transfer path from the conduction band (CB) to the VB, thus hindering recombination. The variation in halogen atomic radii due to solid-solution formation alters the interlayer spacing, subsequently affecting photogenerated carriers' transfer pathways. Ren et al.98 suggested that introducing fluorine, with its smaller atomic radius, into the halogen layer decreases the interlayer spacing and shortens the carrier transfer pathway. The formation of ternary solid solutions also enhances the strength of the built-in electric field, promoting the separation and transfer of charge carriers.
Other studies have demonstrated the significant influence of solid solution formation in modulating the carrier behavior. Solid solution engineering facilitates the spatial segregation of carriers through bandgap expansion, establishment of electron capture traps, and alteration of photogenerated hole mobility, thereby effectively suppressing carrier recombination. This phenomenon is advantageous for enhancing the photocatalytic performance of the catalysts.89 Further, the intrinsic properties of solid solutions can be improved through various techniques. These include morphological control, optimization of optical properties, and facet modification. Additionally, controlling oxygen vacancies (crystal vacancy defects) and synergizing with other modification techniques, such as cation doping, establishing heterojunctions, and inducing plasma resonance effects. These methods are described as follows:
4.2.3. Morphological control.
Solid solution materials of BiOY and BixOyYz are crucial for photocatalysis, particularly for the utilization of visible light. However, the photocatalytic performance of BiOY and BixOyYz solid solutions can still be further improved. Nanoscale morphological engineering through a synthesis process has been conducted to endow solid solutions with unique characteristics for enhanced performance.99 This section provides an overview of the morphological control of BiOY and BixOyYz solid-solution materials employed as photocatalysts in recent studies. This summary encompasses one-dimensional (1D) nanorods, nanowires; two-dimensional (2D) nanosheets, nanoplates; three-dimensional (3D) nanospheres, and the creation of hierarchical and hollow structures. A schematic representation of light undergoing multiple reflections within various structures is illustrated in Fig. 9, including nanospheres formed by the assembly of nanosheets and nanoparticles, isolated nanosheets, hollow nanospheres with interior and exterior views, and nanorods.6
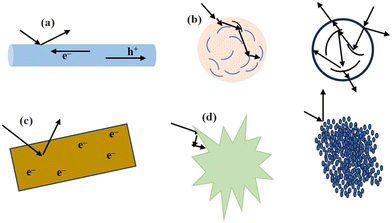 |
| Fig. 9 A schematic illustration depicting multiple reflections within different structures: a) nanorod, b) nanosheets, c) hollow nanoparticles, and d) nanospheres assembled by nanosheets. | |
4.2.3.1. One-dimensional morphology.
One-dimensional nanostructures, including nanorods, nanowires, nanofibers, and nanotubes, are characterized by a high length-to-diameter ratio and offer notable benefits. These advantages include substantial surface area, distinct longitudinal movement pathways for charge carriers, extensive exposure to active sites, and improved light absorption and scattering properties. Zhang et al. (2020) demonstrated that 1D Bi12O17ClxBr2−x (Bi12O17ClBr) nanotube solid solution with rich oxygen vacancies could achieve 93% tetrachlorobiphenyl A (TCBPA) degradation. The tubular structure of the nanotubes, shown in Fig. 10, allows for efficient light absorption and redox capabilities, enhancing their photocatalytic activity. Additionally, the presence of oxygen vacancies in nanotubes contributes to the generation of superoxide and hydroxyl radicals, which play essential roles in the photocatalytic degradation of the target pollutant.100
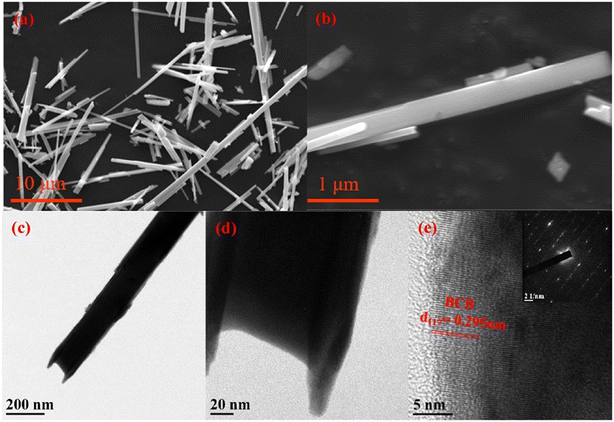 |
| Fig. 10 a and b) SEM, c and d) TEM, and e) high-resolution TEM images of 1D Bi12O17ClBr nanotubes. Reprinted with permission from ref. 100. Copyright (2020) Elsevier. | |
4.2.3.2. Two-dimensional morphology.
There has recently been increasing attention toward low-dimensional materials, mainly 2D nanosheets, and nanoplates, for energy conversion and environmental remediation.101 2D nanostructures offer expanded surface areas, providing ample reactive sites for redox reactions, with manageable adjustments in the exposed facets.102,103 Thi et al.104 synthesized high-quality BiOBr, BiOCl, and BiOCl1−xBrx solid solutions in nanoplate form (Fig. 11a), with BiOCl0.5Br0.5 solid solutions showing unique morphologies because of the increased Cl− content. The preparation of Bi5O7I/BiOBrxI1−x heterostructures via hydrobromic acid etching yielded rod-like morphologies (Fig. 11b), which were controlled by pH variations.105 Additionally, solvothermal methods produce BiOCl0.5Br0.5 solid solution nanosheets and BiOCl1−xBrx@AgBr heterostructures (Fig. 11c), enhancing photocatalytic activity by increasing the surface area and charge separation.106,107 Varying the Br/I ratio resulted in ultrathin BiOBrxI1−x 2D nanosheets with enhanced degradation abilities, indicating the potential for improved pollutant removal.108,109 Furthermore, BiOCl0.5Br0.5 nanoplates (Fig. 11f) with exposed {001} facets display enhanced photocatalytic performance, suggesting their suitability for environmental applications.110 Morphological studies of the BiOCl0.25Br0.75 and Au-BiOCl0.25Br0.75 (Fig. 11g) composites revealed intact heterojunctions, indicating potential enhancements in the degradation efficiency through effective charge transfer mechanisms.111 These observations underscore the significance of morphological characteristics in enhancing the efficiency of bismuth-based photocatalysts.
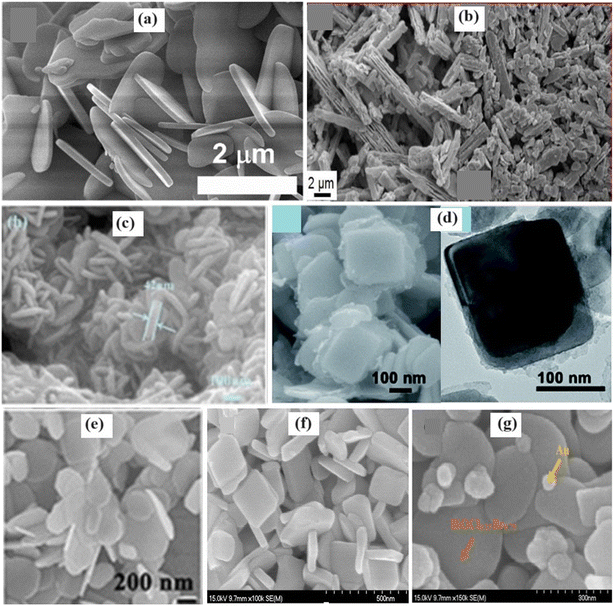 |
| Fig. 11 FESEM of a) BiOCl0.5Br0.5 nanoplate. Reprinted with permission from ref. 104. Copyright (2023) Elsevier; b) Bi5O7I/BiOBrxI1−x heterostructures. Reprinted with permission from ref. 105. Copyright (2023) Elsevier; c) 3CQDs−BiOCl0.5Br0.5 nanosheet structures. Reprinted with permission from ref. 106. Copyright 2022 American Chemical Society; d) BiOCl1−xBrx@AgBr heterostructures;107 e) 2D BiOCl0.5Br0.5 nanoplates. Reprinted with permission from ref. 110. Copyright (2015) John Wiley and Sons; f) BiOCl0.25Br0.75 and g) 1.3% Au-BiOCl0.25Br0.75 composite. Reprinted with permission from ref. 111. Copyright (2017) Elsevier. | |
4.2.3.3. 3D morphology.
Increasingly, there is a growing recognition of the role hierarchical nanostructures play in enhancing the photocatalytic performances of BiOY and BixOyYz solid solutions. These structures, typically composed of nanosheets, offer increased specific surface areas and multiple light reflections, enhancing photon absorption. Different synthetic techniques have been explored to control the morphology of these materials.112–114 For example, BiOCl1−xBrx samples prepared via precipitation at ambient temperature exhibit uniform flower-like architectures (Fig. 12a) with porous structures.115 Whereas chemically synthesized C-BiOBr0.5I0.5 composites display irregular nanoplates, leaf-extract-mediated G-BiOBrxI1−x composites form 3D hierarchical nanospheres (Fig. 12b).116 Additionally, the morphologies of the BiOClxIy solid solutions varied with the Cl-to-I molar ratio, influencing the formation of flower-like microspheres or clustered nanoplates (Fig. 12c).115,116 Incorporating additives such as BiPO4 did not alter the hierarchical microflower morphology of BiOCl0.75Br0.25, indicating successful heterostructure formation.117 Furthermore, the addition of KI during synthesis influenced the morphology of the BiOClxBryIz samples, with varying ratios resulting in different structures, from interleaved wafer-like particles to more extensive and more compact hierarchical spheres (Fig. 12d).118,119
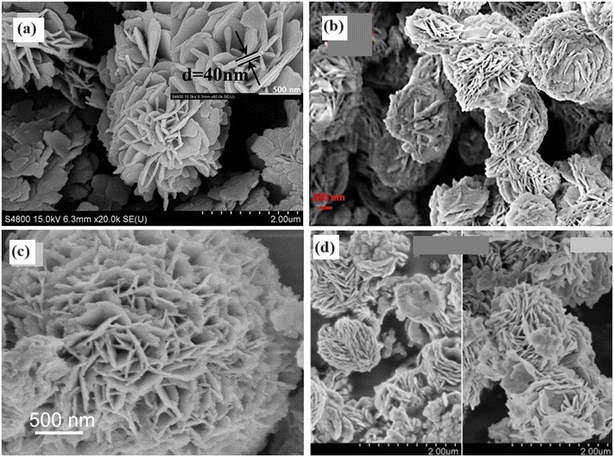 |
| Fig. 12 The morphologies of the 3D structures of a) BiOCl1−xBrx. Reprinted with permission from ref. 115. Copyright (2016) Elsevier; b) G-BiOBrxI1−x composites. Reprinted with permission from ref. 116. Copyright (2019) Elsevier; c) BiOCl3I. Reprinted with permission from ref. 120. Copyright (2016) Elsevier; and d) BiOClxBryIz. Reprinted with permission from ref. 117. Copyright (2015) Elsevier. | |
4.2.4. Crystal vacancy defect.
Oxygen vacancies (OVs) serve as pivotal defects in semiconductor photocatalysts, such as BiOCl, profoundly influencing their photocatalytic performance by expanding light absorption and enhancing photoreactivity. The formation of OVs is particularly notable in ultrathin structures such as nanosheets, which expose a more significant number of surface atoms, facilitating vacancy creation.6,54,121 Alansi et al.122 demonstrated the remarkable photochromic response of 3D-BiOCl0.8Br0.2 nanoflowers, transitioning from white to black under sunlight owing to OV formation (Fig. 13a–c). Zhang et al.123 showed the controllable introduction of OVs by adjusting the halogen composition in BiOY solid solutions, highlighting the significantly higher OV concentration in Br-solid solution-based BiOY compared to pure BiOY. Additionally, the prepared BiOClxI1−x solid solution with variable Cl to I ratios exhibited OV formation.124 Ji et al.125 revealed the significant impact of the OV content on the carrier recombination rates in a BiOCl0.5I0.5 solid solution, where a higher OV content led to enhanced carrier migration and reduced recombination rates (Fig. 13d). Ren et al.98 synthesized F/Br/I ternary halogen solid solution-based BiOY with varying OV contents and observed that a higher OV content effectively suppressed photogenerated carrier complexes, thereby improving the carrier lifetime.
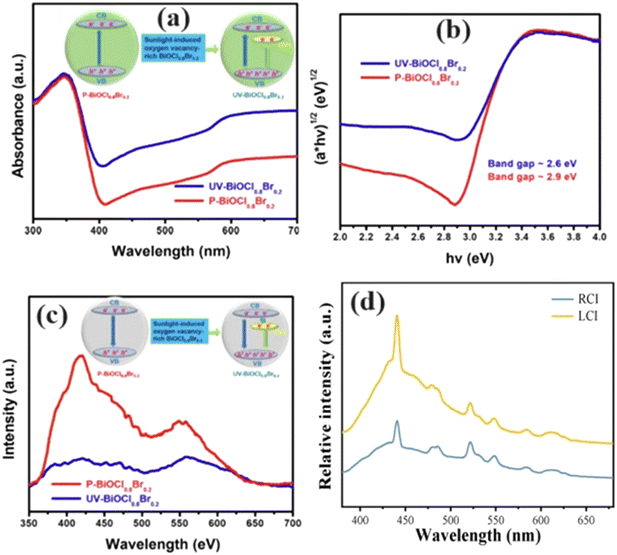 |
| Fig. 13 a) UV-vis diffuse reflectance spectra, b) band gap energies from Tauc plots, c) photoluminescence emission spectra of the P-BiOCl0.8Br0.2 and UV- BiOCl0.8Br0.2. Reprinted with permission from ref. 122. Copyright (2022) Elsevier; d) PL spectra of BiOCl0.5I0.5 rich in OV (RCI) and BiOCl0.5I0.5 less rich in OV (LCI). Reprinted with permission from ref. 125. Copyright (2022) Elsevier. | |
Xu et al.126 also employed a molten salt approach to produce BiOCl1−3xI3x with an increased OV concentration compared to traditional hydrothermal methods. They underscored the critical role of OVs in enhancing photocatalytic performance and offered insights into strategies for controlling the OV content in semiconductor photocatalysts. Other studies reported that the improved carrier separation efficiency was attributed to introducing OVs into the solid solution. These vacancies, formed by the absence of oxygen atoms, act as traps for capturing photoexcited electrons, impeding carrier recombination and promoting the adsorption and activation of small molecules during interfacial reactions, thereby enhancing the reaction efficiency.79,127 Moreover, Guo et al. reported that the presence of various oxygen species, including lattice oxygen (OL), weakly bound or chemisorbed oxygen (OC), and OV/OT (OT = OV + OL + OC), provides insights into the relationship between the OV concentration and solid solution ratio.128
4.2.5. Other integrated intrinsic properties enhancement strategies.
The synergistic augmentation effect achieved through the integration of diverse modification techniques is widely recognized for its positive impact on the photocatalytic efficacy of the catalyst. Incorporating solid solutions with additional modification approaches significantly expands the scope of design possibilities and potential applications of BiOY and BixOyYz materials. Researchers have merged solid solutions with an array of modification techniques to refine the intrinsic characteristics and improve the photocatalytic performance of BiOY and BixOyYz materials.
4.2.5.1. Solid solution combined with metal or nonmetal.
Metal doping has long been a viable method for fine-tuning BiOY and BixOyYz band structures, enhancing the carrier separation efficacy. Theoretical modeling has demonstrated that the combined impact of metal doping and solid solution reduces the catalyst's band gap and improves its oxidative capabilities, offering significant advantages in the photooxidation of contaminants.129 Qi et al.111 documented that incorporating Au into BiOCl1−xBrx enhanced the photocatalytic activity of the composite materials. The interplay between Au NPs and BiOCl1−xBrx led to elevated Schottky barriers. Au NPs acted as electron reservoirs, fostering the disjunction of e−/h+ pairs and advancing interfacial electron transfer. Furthermore, these nanoparticles demonstrate robust absorption of visible light owing to surface plasmon resonance, augmenting optical absorption and photocatalytic efficacy when exposed to visible light irradiation.130
Similarly, Han et al.131 engineered Fe3+-doped BiOClxI1−x (Fe–BiOClxI1−x@AP) supported on amidoxime-functionalized fibers. The Fe–O–Bi coordination site enhanced Fe–BiOClxI1−x light absorption via electron transfer. The fiber support, Fe ions, and Cl/I alloy synergistically promoted electron transfer. This mechanism involved the transfer of electrons from O2− to the Bi3+ 6p orbital in BiOClxI1−x, with the addition of Fe3+ enhancing this transfer through electron repulsion interactions. Moreover, the amidoxime moieties on the fiber substrate contributed lone pair electrons to Fe3+, thereby promoting electron transfer from O2− to Bi3+. Consequently, Fe–BiOClxI1−x@AP exhibited a superior tetracycline photodegradation performance.
4.2.5.2. Solid solutions with heterojunction.
Solid solution construction narrows the energy band gap, intensifies light absorption, and increases carrier recombination. Conversely, the electric fields of heterojunctions segregate carriers, mitigating the drawbacks of solid solutions. The stratified BiOY and BixOyYz solid solutions enhanced the interfacial contact, improving charge transfer during heterojunction formation. Combining solid solutions and heterostructures reduced the bandgap, enhanced light absorption, and stabilized the photocatalytic systems by mitigating carrier recombination through heterojunction electric fields.132
The heterojunction between BiOBr0.75I0.25 and BiOIO3 was prepared via a simple deposition–precipitation technique, which showed the distinct features of BiOBr0.75I0.25 and BiOIO3. BiOBr0.75I0.25 has a narrow energy band gap (2.02 eV) responsive to visible light, whereas BiOIO3 has a wider band gap (2.87 eV) and is less responsive to visible light. Heterojunction formation allows for an advantageous combination of these materials, improving the photocatalytic performance of the composite. The interface between BiOBr0.75I0.25 and BiOIO3 facilitated the separation and transfer of photoexcited charge carriers, significantly promoting the degradation of pollutants under visible light irradiation.133
The formation of BiOY/Ag3PO4, BiOY/Fe3O4@SiO2, and BiOY/TiO2 heterostructures can facilitate efficient electron transfer and separation of charge carriers, resulting in improved photocatalytic activity under visible-light irradiation. These heterostructures exhibited improved degradation of phenol, PPCPs, benzophenone-3, ibuprofen, and RhB dye compared with BiOY solid solution. These heterojunctions exploit the unique properties of each material to broaden the range of light absorption (Fig. 14a) and enhance the overall efficiency of photocatalysis.131–133 Another strategy involves exploring 2D–2D interface heterojunctions (Fig. 14b), such as Ag3PO4-BiOCl1−xBrx,
65 BiOClxBr1−x/GO66 and Ti3C2/BiOClxBr1−x,134 which provided enhanced gas adsorption and electron–hole pair separation capabilities. These structures promote synergistic effects between the components, resulting in an enhanced photocatalytic activity for the degradation of various pollutants.
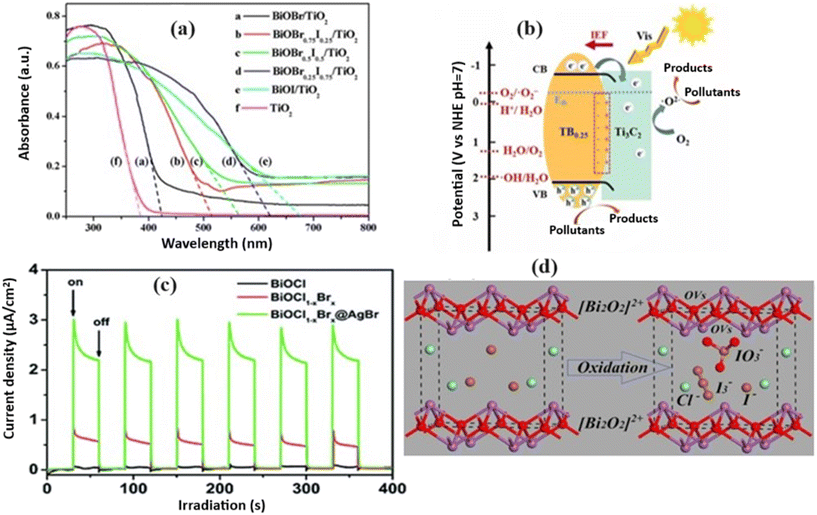 |
| Fig. 14 a) UV-vis diffuse reflectance spectra of TiO2–BiOBrxI1−x heterojunctions and TiO2 NRs. Reprinted with permission from ref. 135. Copyright (2020) Elsevier; b) 2D/2D interfaces of Ti3C2/BiOClxBr1−x. Reprinted with permission from ref. 134. Copyright (2023) Elsevier; c) transient photocurrent responses of BiOCl, BiOCl1−xBrx, and BiOCl1−xBrx@AgBr. Reprinted with permission from ref. 107. Copyright (2023) Elsevier; d) schematic representation illustrating the mechanism of IO3− and I3− ions formation for BiOCl0.9I0.1. Reprinted with permission from ref. 136. Copyright (2019) Elsevier. | |
Moreover, several studies have emphasized the significance of heterojunction interfaces in promoting efficient charge transfer and separation. Heterojunctions with well-developed interfaces, such as Bi5O7I/BiOBrxI1−x
67 or BiOCl1−xBrx@AgBr,107 exhibit superior photocatalytic performance owing to the enhanced separation of electron–hole pairs and extended carrier lifetime (Fig. 14c). The reported study introduces a direct redox-mediator-free Z-scheme heterojunction between BiPO4 and BiOCl0.9I0.1, prepared through a water bath reaction at 90 °C. This synthesis method ensured a close interfacial contact between the two materials, forming a heterostructure with multiple vacancies and valences. These defects, including Bi5+, Bi3+, Bi(3−x)+, I−, I3−, and IO3−, promoted efficient charge separation and transfer during photocatalysis (Fig. 14d).136
4.2.5.3. Solid solutions with carbon-based compounds.
Further improvement of the intrinsic properties of BiOY and BixOyYz was achieved by investigating the influence of carbon-based compounds such as graphitic carbon nitride (g-C3N4), graphene, and carbon quantum dots (CQD) in improving the photocatalytic capacity of BiOY and BixOyYz solid solutions.89 These carbon-based compounds have been explored due to their synergistic properties and high surface areas.137 g-C3N4 emerged as a promising material despite its limited carrier separation efficiency. Combining g-C3N4 with BiOY and BixOyYz led to heterostructures with enhanced photocatalytic efficacies.89
A type II g-C3N4/BiOCl0.2Br0.8 heterojunction was fabricated using a straightforward immersion-precipitation technique.138 Remarkably, g-C3N4/BiOCl0.2Br0.8 exhibited an extended photoluminescence lifetime compared to pristine g-C3N4, indicating enhanced carrier separation efficiency. This augmentation enhanced RhB degradation activity for g-C3N4/BiOCl0.2Br0.8 compared to the individual g-C3N4 and BiOClxBr1−x counterparts.138 To augment the efficacy of organic pollutant elimination, a type-II g-C3N4/BiOClxI1−x heterojunction (BCI-CN-P) rich in OV was synthesized via a precipitation technique aided by PVP.139 The mechanism of carrier transfer (Fig. 15a) revealed preferential photogenerated electron migration towards OV-induced defect sites before transitioning to the CB at a lower energy level. The photocurrent characteristics (Fig. 15b) highlighted the synergistic effect of the solid solution, heterojunction formation, and OV in inhibiting photogenerated electron–hole recombination. Consequently, BCI-CN-P demonstrated remarkable efficiency in complete BPA degradation within 40 min.139 To enhance the semiconductor's redox potential and enhance the efficiency of RhB degradation, a Z-scheme graphene-like g-C3N4)/BiOClxI1−x heterojunction was fabricated through in situ deposition. A systematic investigation was conducted to determine the optimal composition of the catalyst for RhB degradation, and the photoelectric properties of the catalysts were evaluated. Notably, the 10-CN/BiOCl0.8I0.2 composite showed reduced photoluminescence intensity, increased photocurrent, and lowered resistance, indicating the practical separation of charge carriers by the catalyst.140
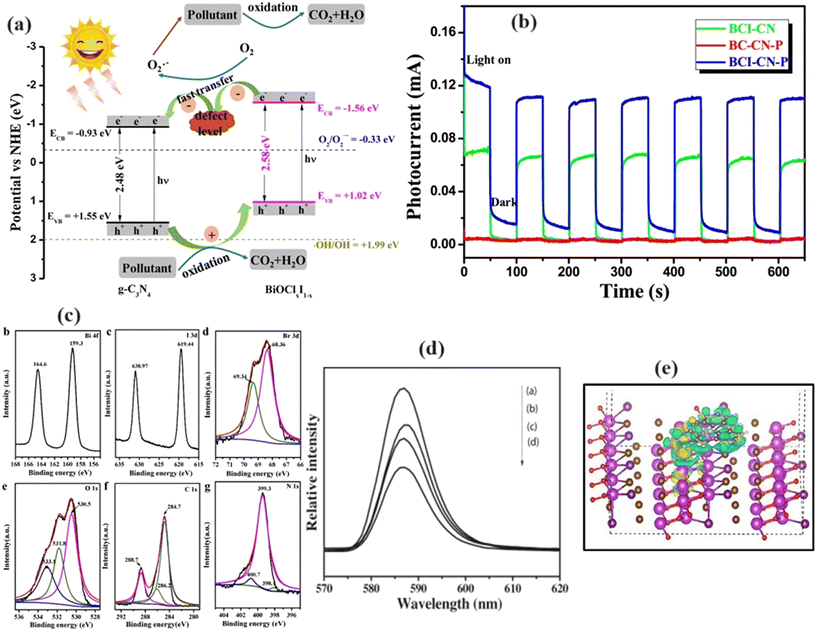 |
| Fig. 15 a) Energy band structures and electron–hole pairs separation over BCI-CN-P nanosheets, b) transient photocurrent responses. Reprinted with permission from ref. 139. Copyright (2019) Elsevier; c) X-ray photoelectron spectroscopy of g-C3N4 and BiOBr0.2I0.8. Reprinted with permission from ref. 141. Copyright (2018) Elsevier; d) PL spectra of the as-synthesized samples. Reprinted with permission from ref. 142. Copyright (2013) Elsevier; e) 3D charge density differences of N-CQD/BiOI0.25Br0.75: yellow and blue isosurfaces indicate charge accumulation and depletion, respectively, compared to isolated N-CQD and BiOI0.25Br0.75. Reprinted with permission from ref. 143. Copyright (2020) Elsevier. | |
The preparation of g-C3N4-BiOClxBr1−x hybrids via a solvothermal method was aimed at exploring the synergistic effects of combining these materials in a direct Z-scheme heterojunction. The resulting heterojunction exhibited improved photocatalytic performance, attributed to effective charge separation, surpassing that of pristine g-C3N4 and BiOCl0.5Br0.5.144 Engineered band structure improvements bolstered the light absorption, redox capabilities, and transfer of charge carriers. The direct Z-scheme mechanism retains a strong redox potential, further enhancing the photocatalytic performance.144 A 3D hybrid photocatalyst integrating g-C3N4 and BiOBr0.2I0.8via hydrothermal synthesis showed a tailored band structure.141 Tailoring the energy-band alignments facilitates efficient charge migration at the heterojunction interface. The formed heterojunction significantly enhanced the charge separation, providing a conducive environment for separating electron–hole pairs and improving the quantum efficiency. X-ray photoelectron spectroscopy analysis (Fig. 15c) revealed a distinct channel for photogenerated charge transmission within the heterojunction. Compared with bare g-C3N4 and BiOBr0.2I0.8, the 3D hybrid photocatalyst demonstrated a notable improved photocatalytic performance, primarily ascribed to effective separation of charge carriers.141
Using a solvothermal technique, graphene sheets were grafted onto three-dimensional microspheres of BiOBr0.2I0.8, comprising nanoplates uniformly dispersed across the graphene surface. This amalgamation facilitated augmented separation and transfer of charges, as confirmed by photoluminescence findings (Fig. 15d). The composite showed diminished electron–hole recombination rates, credited to electron migration from the VB to the CB and subsequently to the graphene sheets, thereby impeding direct recombination.142 The composite of BiOBr0.2I0.8/graphene exhibited remarkable photocatalytic efficacy in the degradation of rhodamine B (RhB) and phenol under visible-light illumination.142 N-doped CQDs (N-CQDs) were incorporated into a BiOIxBr1−x solid solution forming N-CQDs/BiOI0.25Br0.75 heterojunction through in situ co-precipitation. The binding affinity between BiOIxBr1−x and N-CQDs was facilitated by the interaction of amino and carboxyl groups on N-CQDs' surface with Bi atoms in the precursor compounds derived from bismuth. This interaction led to enhanced charge transfer (Fig. 15e) and improved the intrinsic properties of the photocatalyst. The hierarchically layered microspheres of BiOIxBr1−x, composed of nanosheets, facilitated multiple reflections of light, thereby enhancing light harvesting. The combined effect of light scattering and the internal electric field from N-CQDs to BiOI0.25Br0.75 contributed to enhanced photocatalytic activity.143
4.3. Decomposition of pollutants in water and in air
Different water contaminants such as organic dyes,145,146 metal ions,147,148 phenolic compounds,149,150p-hydroxyphenylacetic acid,151 pharmaceuticals,146 and personal care products152 have been widely reported to have negative impacts on the global ecosystem and human health. The oxidative-reductive interactions between BiOY, BixOyYz and aquatic pollutants rely on the reactive species generated by electrons or holes. It is feasible to fabricate BiOY and BixOyYz materials with tailored light absorption capabilities through deliberate control of the structural configuration during synthesis. This enables customization of the photocatalytic characteristics to suit specific applications in this domain.19
4.3.1. Removal of dyes.
Inspired by the discovery of the photocatalytic efficacy of BiOY and BixOyYz photocatalysts, a range of engineered BiOY and BixOyYz materials with layered structures, has been employed for the degradation of different organic dyes, including RhB,85,145 MO,84 MB,4 Reactive Blue KN-R,107 MG,153 and acid orange 7.154Table 1 shows the literature on the photocatalytic degradation of different dyes over BiOY and BixOyYz solid solutions. Some researchers have reported that solid solutions of BiOY and BixOyYz, in comparison to their pure forms, demonstrated a remarkable capability to achieve complete removal of RhB, MO, and MG dyes, thereby underscoring their enhanced efficiency in dye-removal processes.84,87,153–156
Table 1 Summary of the reported literature on the photocatalytic degradation of various organic dyes using BiOY and BixOyYz solid solution
Solid solution material |
Synthesis method |
Contaminant & dosage |
Irradiation source |
Photocatalytic activity & active species |
Ref. |
BiOCl1−xBrx; BiOCl0.5Br0.5 |
Hydrothermal |
20 mg L−1 RhB, 1 g L−1 |
Visible light |
97% in 60 min higher than BiOCl (48%) & BiOBr (45%) |
80
|
Bi(NO3)3·5H2O, water |
BiOClxBr1−x; BiOCl0.5Br0.5 |
Glycol-assisted hydrothermal |
20 mg L−1 MO, 1 g L−1 |
Visible light |
98.27% in 60 min ∼10.9, 2.7, & 29.8 times higher than pure BiOCl, BiOBr & TiO2, respectively. ˙O2−, h+, & ˙OH |
84
|
Bi(NO3)3·5H2O, polyacrylamide & cetyltrimethylammonium bromide |
Bi4O5BrxI2−x; Bi4O5BrI, |
Solvothermal |
10 mg L−1 RhB, 0.1 g L−1 |
Simulated solar light |
99% in 30 min h+, ˙O2−, & e− |
85
|
Bi(NO3)3·5H2O, polyethylene glycol |
Bi24O31ClxBr10−x; Bi24O31Cl5Br5 |
Solvothermal |
15 mg L−1 RhB, 0.5 g L−1 |
Visible light |
98.9% in 30 min ∼1.6 & 1.9 times higher than Bi24O31Br10 & Bi24O31Cl10, respectively. ˙O2− & h+ |
88
|
Bi(NO3)3·5H2O, ethylene glycol |
BiOCl1−xBrx (0 ≤ x ≤ 1); BiOCl0.6Br0.4 |
Etching process |
10 wt% RhB |
Visible light |
93.6% in 40 min |
145
|
Bismuth glass, H2SO4 |
BiOBrxI1−x; BiOBr0.5I0.5 |
Precipitation |
20 mg L−1 MG, 0.4 g L−1 |
Visible light |
∼100% in 120 min h+ |
153
|
Bi(NO3)3·5H2O, ethylene glycol |
CFC/BiOBrxI1−x; CFC/BiOBr0.8I0.2 |
Solvothermal |
20 mg L−1 acid orange 7 |
Visible light |
94.1% in 120 min |
154
|
Bi(NO3)3·5H2O, DMF, ethylene glycol |
BiOBrxI1−x (x = 0.0–1.0); BiOBr0.25I0.75 |
Solvothermal |
10 mg L−1 RhB, 0.2 g L−1 |
Visible light |
100% in 20 min ˙O2− |
155
|
Bi(NO3)3·5H2O, ethylene glycol |
BiOClxI1−x; BiOCl0.75I0.25 |
Alcoholysis |
20 mg L−1 RhB, 1 g L−1 |
Visible light |
∼100% in 150 min BiOCl (81.2%) and BiOI (31.5%) in 240 min |
156
|
Bi(NO3)3·5H2O, ethyl alcohol |
BiOBrxI1−x; BiOBr0.75I0.25 |
Ultrasonication synthesis. Bi(NO3)3·5H2O, acetic acid |
20 mg L−1 RhB, 1 g L−1 |
Visible light |
h+ |
157
|
BiOBrxI1−x (0 ≤ x ≤ 1)@PAN; BiOBr0.5I0.5@PAN |
Electrospinning |
10 mg L−1 MO, 0.4 g L−1 |
Visible light |
94.4% in 180 ∼2.5 and 3.2 times higher than BiOBr@PAN and BiOI@PAN, respectively. ˙O2− |
158
|
Bi(NO3)3·5H2O, Polyvinylpyrrolidone |
BiOBrxI1−x; BiOBr0.3I0.7 |
Ultrasonication |
10 mg L−1 MO, 0.67 g L−1 |
Visible light |
92% in 40 min. Higher than pure BiOCl, BiOBr. ˙O2− & h+ |
159
|
Bi(NO3)3·5H2O, water |
BiOClxI1−x (x = 0.75) |
Precipitation |
20 mg L−1 RhB, 1 g L−1 |
Visible light |
99.2% in 80 min |
160
|
Bi(NO3)3·5H2O, nitric acid, polyvinylpyrrolidone, citric acid |
BiOClxBr1−x; BiOClBr |
Precipitation |
10−5 RhB, 0.5 g L−1 |
Visible light |
99% in 30 min ∼2.0 & 6.9 times higher than BiOBr & BiOI, respectively. 3.5, 14.9 |
161
|
Bi(NO3)3·5H2O, nitric acid, water |
BiOCl0.808Br0.192/alumina |
Hydrolysis & precipitation |
5 mg L−1 RhB |
Visible light |
>99.3% in 60 min ˙O2− & ˙OH |
162
|
Aluminium tri-sec-butoxide, water Bi(NO3)3·5H2O, acetic acid |
BiOClxBr1−x; BiOCl0.9Br0.1 |
Solvothermal |
15 mg L−1 RhB, 0.2 g L−1 |
UV light |
44.83% in 100 min ∼51.6 & 2.6 times higher than BiOBr & BiOCl, respectively. ˙O2−, ˙OH & h+ |
163
|
Bi(NO3)3·5H2O, ethylene glycol |
BiOBrxI1−x; BiOBr0.8I0.2 |
Precipitation |
20 mg L−1 RhB, ∼0.33 g L−1 |
Visible light |
99% in 90 min 1.7 & 5.4 times higher than BiOBr & BiOI, respectively. h+ |
164
|
Bi(NO3)3·5H2O, ethylene glycol |
BiO(ClxBr1−x); BiO(Cl0.5Br0.5) |
Hydrothermal |
15 mg L−1 RhB, 0.5 g L−1 |
Visible light |
74.2% in 60 min h+ |
165
|
Bi(NO3)3·5H2O, water |
BiOBrxI1−x; BiOBr0.75I0.25 |
Solvothermal |
10 mg L−1 RhB, 0.4 g L−1 |
Visible light |
17.9 & 3.8 times higher than BiOBr & BiOI, respectively |
166
|
Bi(NO3)3·5H2O, polyvinylpyrrolidone, ethylene glycol |
Solid solutions such as BiOBr0.5I0.5,153 BiOBr0.25I0.75,155 and BiOCl0.75I0.25 (ref. 156) exhibit complete degradation of dyes under visible light, attributed to their oxidation ability, valence band potential, and high specific surface area. Additionally, BiOCl1−xBrx solid solutions, particularly at x = 0.5, showed enhanced activity in dye degradation owing to efficient electron–hole pair separation and stability.80,84 Bismuth-rich Bi4O5BrxI2−x solid solutions synthesized via solvothermal methods displayed optimal performance at a Br/I ratio of 1
:
1, inhibiting the recombination of photoinduced e−/h+ pairs and improving the photocatalytic efficiency.85 Bi24O31ClxBr10−x solid solutions demonstrate enhanced photocatalytic performance relative to individual compositions, with Bi24O31Cl5Br5 demonstrating the highest efficiency ascribed to its favorable band gap configuration and effective charge transfer mechanisms.88
Nanosheet-shaped BiOCl1−xBrx solid solutions, particularly BiOCl0.6Br0.4, showed excellent performance in dye degradation under visible light due to their energy band control and efficient catalysis.145In situ growth of BiOBrxI1−x solid solutions on carbon fiber cloth and their incorporation into nanofibers enhanced their photocatalytic activity and stability, demonstrating their potential for environmental applications.154 Optimized solid solutions of BiOMxR1−x exhibited notable enhancements in dye removal efficiency and stability.160 BiOClxBr1−x sheets with a Cl
:
Br ratio of 1
:
1 demonstrated high dye degradation activity, ascribed to the synergistic effect of photosensitization and photocatalysis.161 Incorporating surfactants during synthesis improves the photocatalytic activity and reusability, making them advantageous for industrial applications.159 Visible-light-responsive BiOBrxI1−x solid solutions exhibit tunable band gaps and demonstrate high photocatalytic efficiency for dye degradation.164 BiO(ClxBr1−x) solid solutions displayed efficient dye degradation under visible light, emphasizing the interplay between light absorption capacity and photooxidation reactions.165 BiOBr0.75I0.25 showed superior performance over P25 TiO2 in degrading organic contaminants under visible light, which was ascribed to its distinctive band structure and charge separation efficiency. These studies underscore the significance of advanced photocatalytic materials in environmental remediation, offering pathways toward cleaner ecosystems and human well-being.166
4.3.2. Pharmaceuticals.
There are a limited number of studies on the degradation of pharmaceuticals using BiOY and BixOyYz based solid solutions photocatalysis processes. Thus, there is a pressing need for further research to develop efficient techniques for treating refractory pharmaceuticals in wastewater treatment plants. Table 2 illustrates the degradation of typical pharmaceuticals using BiOY and BixOyYz-based solid solutions photocatalysts. The target pharmaceuticals studied in BiOY and BixOyYz solid-solution solutions photocatalysis include ciprofloxacin (CIP),154,167 ibuprofen, amoxicillin (AMX), tetracycline (TC),153,154 chlortetracycline hydrochloride (CT-HCl), acetophenone (AP), ofloxacin, tetracycline hydrochloride (TC-HCl), sulfamethazine (SMT),168 levofloxacin (LVFX),154,165,169 and hydroxyphenylacetic acid (p-HPA).96,151 Remarkably, tetracycline has emerged as the predominant pharmaceutical target in BiOY and BixOyYz solid-solution-driven photocatalysis systems. The application of pure BiOY and BixOyYz materials in the degradation of pharmaceuticals has been hindered by their high photocorrosion and high recombination of e−/h+ pairs.
Table 2 Summary of the reported literature on the photocatalytic degradation of various pharmaceuticals on BiOY and BixOyYz solid solution
Solid solution material |
Synthesis method |
Contaminant & dosage |
Irradiation source |
Photocatalytic activity & active species |
Ref. |
BiOClxIy; BiOCl0.75I0.25 |
Precipitation |
p-HPA & ACTP, 0.6 g L−1, 1 g L−1 |
Solar light |
∼100 & 80% in 180 min ˙O2− |
96
|
Bi(NO3)3·5H2O, ethylene glycol, water |
BiOCl0.75I0.25 |
Precipitation |
22.8 mg L−1p-HPA, 0.6 g L−1 |
Simulated solar |
∼100% in 120 min |
151
|
Bi(NO3)3·5H2O, ethylene glycol, water |
BiOBrxI1−x; BiOBr0.95I0.05 |
Precipitation |
20 mg L−1 TC, 0.4 g L−1 |
Visible light |
85% TC in 30 min ∼3.5 & 14.9 times higher than BiOBr & BiOI, respectively. ˙O2− & h+ |
153
|
Bi(NO3)3·5H2O, ethylene glycol |
CFC/BiOBrxI1−x; CFC/BiOBr0.8I0.2 |
Solvothermal |
10 mg L−1 LVFX, CIP, & TC |
Visible light |
Levofloxacin (94.1%), CIP (94.5%), & TC (98.9%) in 120 min e−, ˙O2− & h+ |
154
|
Bi(NO3)3·5H2O, DMF, ethylene glycol |
BiO(ClxBr1−x); BiO(Cl0.5Br0.5) |
Hydrothermal |
15 mg L−1 acetophenone, 0.5 g L−1 |
Visible light |
56.05% in 240 min h+ |
165
|
Bi(NO3)3·5H2O, water |
BiOX (Cl : Br = 1 : 3)–U |
Sono-solvothermal |
50 mg L−1 CIP |
Simulated solar light |
100% in 120 min Greater than pristine BiOBr–U (74.9%) & BiOCl–U (45.9%). ˙OH & h+ |
167
|
Bi(NO3)3·5H2O, ethylene glycol |
F-BiOCl0.4Br0.3I0.3 (Bi : F = 3 : 2) |
Solvothermal |
10 mg L−1 SMT, 0.5 g L−1 |
Visible light |
99.6% in 60 min 2.6 times higher than BiOCl0.4Br0.3I0.3. ˙O2− & h+ |
168
|
Bi(NO3)3·5H2O, NaF, ethylene glycol |
(BiOBr)x(Bi7O9I3)1−x; (BiOBr)0.75(Bi7O9I3)0.25–U |
Sono-solvothermal |
50 mg L−1 levofloxacin, 1 g L−1 |
Solar light |
95.4% in 120 min |
169
|
Bi(NO3)3·5H2O, Bi7O9I3, ethylene glycol |
Several solid solutions, including BiOCl0.75I0.25 and BiOBr0.95I0.05, exhibit high degradation efficiencies for various pollutants, including p-HPA, AMX, and TC.96,151,153In situ growth of BiOBrxI1−x solid solutions on carbon fiber cloth resulted in tunable bandgaps and enhanced photocatalytic activity. Notably, CFC/BiOBr0.8I0.2 showed exceptional efficiency in degrading antibiotics under visible light.154 Additionally, (Cl
:
Br = 1
:
3)–U solid solution demonstrated outstanding CIP removal efficiency, attributed to its unique morphology and effective electron–hole pair separation.167 F-doped BiOCl0.4Br0.3I0.3 displayed remarkable activity in sulfamethazine degradation due to its narrow band gap and unique morphology.168 Ultrasonically fabricated (BiOBr)0.75(Bi7O9I3)0.25-U exhibited high degradation efficiency for LVFX, attributed to its optimal band gap, enhanced charge transportation, and large specific surface area.169
4.3.3. Phenolic compounds.
Phenolic compounds are extensively utilized in industrial and everyday activities and have been identified as prevalent contaminants in water sources. Owing to their inherent stability and carcinogenic properties, these compounds pose a significant risk to human health and aquatic ecosystems, causing harm and posing threats to both.170Table 3 illustrates the degradation of phenolic compounds by BiOY and BixOyYz-based solid solutions photocatalysts. Complete degradation of phenolic compounds such as hydroquinone, 4-chlorophenol, phenol, and resorcinol over (Bi4O5BrxI2−x; Bi4O5BrI,),85 (Bi4O5BrxI2−xCy; Bi2.8C1.2O5Br0.5I1.5),171 and (BiOBrxI1−x; BiOBr0.5I0.5),172 and BPA over (g-C3N4/BiOClxI1−x)139 and (BiOClxI1−x-Ovs; BiOCl0.5I0.5-Ovs)173 have been reported. The complete degradation was attributed to the efficient segregation of photoinduced electron–hole pairs. The synergistic interaction between the solid solution and OV and a strong internal electric field facilitated this phenomenon. The unique architectural features, combining a 2D structure and dominant {0 0 1} crystal facets, also contributed to this process.
Table 3 Summary of the reported literature on the photocatalytic degradation of phenolic compounds on BiOY and BixOyYz solid solution
Solid solution material |
Synthesis method |
Contaminant & dosage |
Irradiation source |
Photocatalytic activity & active species |
Ref. |
Bi4O5BrxI2−x; Bi4O5BrI |
Solvothermal |
10 mg L−1 phenol, 0.5 g L−1 |
Simulated solar light |
∼100 in 40 min ∼ 6.4 & 8.5 times higher than that of pure Bi4O5Br2 and Bi4O5I2 h+, ˙O2−, & e− |
85
|
Bi(NO3)3·5H2O, polyethylene glycol |
Bi24O31ClxBr10−x; Bi24O31Cl5Br5 |
Solvothermal |
15 mg L−1 phenol, 0.5 g L−1 |
Visible light |
81.0% in 120 min |
88
|
Bi(NO3)3·5H2O, ethylene glycol |
g-C3N4/BiOClxI1−x; |
Precipitation |
10 mg L−1, BPA, 0.6 g L−1 |
Visible light |
∼100% in 40 min h+ & ˙O2− |
139
|
Bi(NO3)3·5H2O, glycerol, PVP |
BiO(OH)xI1−x; BiO(OH)0.45I0.55 |
Chemical precipitation |
10 mg L−1 2-CP, ∼1.6 g L−1 |
Visible light |
88% in 40 min 10 times higher than BiOI. e− & h+ |
149
|
Bi(NO3)3·5H2O, acetic acid, water |
B-IxBr1−x; B–I0.3Br0.7 |
Microwave heating |
10 mg L−1 4NP, 3NP & BPA, 1 g L−1 |
Visible light |
97.5%, 72.8, & 27.5 for BPA, 4NP, & 3NP, respectively in 180 min h+, ˙O2−, & ˙OH |
150
|
Bi(NO3)3·5H2O, ethylene glycol |
BiOBrxI1−x; BiOBr0.5I0.5 |
Precipitation |
10 mg BPA, 0.4 g L−1 |
Visible light |
— |
153
|
Bi(NO3)3·5H2O, ethylene glycol |
BiOBrxI1−x (0 ≤ x ≤ 1)@PAN; BiOBr0.5I0.5@PAN |
Electrospinning |
10 mg L−1 BPA, 0.4 g L−1 |
Visible light |
94.4% in 180 ∼1.9 times higher than BiOI@PAN. ˙O2− and h+ |
157
|
Bi(NO3)3·5H2O, Polyvinylpyrrolidone |
BiOBrxI1−x; BiOBr0.75I0.25 |
Solvothermal |
10 mg L−1 BPA, 0.4 g L−1 |
Visible light |
17.3 & 2.3 times higher than BiOBr & BiOI, respectively |
166
|
Bi(NO3)3·5H2O, polyvinylpyrrolidone, ethylene glycol |
Bi4O5BrxI2−xCy; Bi2.8C1.2O5Br0.5I1.5 |
Solvothermal |
10 mg L−1 phenol, 1 g L−1 |
Visible light |
100% in 60 min h+ & ˙O2− |
171
|
Bi(NO3)3·5H2O, ethylene glycol |
BiOBrxI1−x; BiOBr0.5I0.5 |
Solvothermal |
20 mg L−1 phenol, 2 g L−1 |
Visible light |
100% in 80 min ˙O2− |
172
|
Bi(NO3)3·5H2O, ethylene glycol |
BiOClxI1−x-OVs; BiOCl0.5I0.5-OVs |
Solvothermal |
5 mg L−1 BPA, 0.5 g L−1 |
Visible light |
∼100% in 45 min 22.4, 3.1 and 4.5 times higher than BiOCl, BiOCl-OVs and BiOCl0.5I0.5, respectively. h+ & ˙O2− |
173
|
Bi(NO3)3·5H2O, ethylene glycol, polyvinylpyrrolidone |
Ag–AgCl0.5Br0.5/BiOCl0.5Br0.5 |
Solvothermal |
10 mg L−1 BPA, 0.5 g L−1 |
Visible light |
84.52% in 300 min ˙O2−, & h+ |
174
|
Bi(NO3)3·5H2O, AgNO3, ethylene glycol |
Ag–AgI/AgCl/Bi3O4Cl0.5Br0.5 |
Photo-reduction |
10 mg L−1 phenol, 0.5 g L−1 |
Visible light |
57.7% in 300 min 4 & 1.5 times higher than Bi3O4Cl0.5Br0.5 & Ag–AgI/AgCl, respectively |
175
|
Bi(NO3)3·5H2O, AgNO3, Bi2O3, absolute ethanol |
BiOCl1−xBrx; BiOCl0.3Br0.7 |
Solvothermal |
10 mg L−1 PNP & TBBPA, 1 g L−1 |
Visible light |
98 & in 60 min & 97% in 30 min. h+ & ˙O2− |
176
|
Bi(NO3)3·5H2O, 2-methoxyethanol |
BiOBrnI1−n; BiOBr0.75I0.25 |
Solvothermal |
20 mg L−1p-cresol, 1 g L−1 |
Visible light |
>99% in 90 min 31 & 1.7 times higher than pure BiOBr & BiOI, respectively. ˙OH, h+, & ˙O2− |
177
|
Bi(NO3)3·5H2O, dimethylformamide, ethylene glycol |
Several other studies have explored the synthesis and performance of bismuth-based solid-solution photocatalysts for phenol degradation. Liu et al.88 reported enhanced phenol degradation using Bi-based solid solutions, outperforming pure Bi24O31Cl10 and Bi24O31Br10 owing to the improved band structures. Ji et al.149 showed ten times higher degradation of 2-chlorophenol compared to BiOI, which was attributed to efficient interfacial reactions and electron migration in BiO(OH)xI1−x solid solutions. Chachvalvutikul et al.150 demonstrated superior activity in phenolic compound degradation using Bi7O9I3–Bi4O5Br2 solid-solution photocatalysts with B-I0.3Br0.7, showing enhanced degradation rates and improved charge carrier separation. Liu et al.158 found that BiOBrxI1−x@PAN nanofibers exhibited 1.9 times higher degradation of BPA than BiOI@PAN, with BiOBr0.75I0.25 (ref. 166) showing significant improvement over pure BiOBr and BiOI. Additionally, Ag–AgCl0.5Br0.5/BiOCl0.5Br0.5 (ref. 174) and a polycomplex of Ag–AgI/AgCl/Bi3O4Cl0.5Br0.5 (ref. 175) demonstrated remarkable photocatalytic activities, attributed to synergistic effects that facilitate charge separation. Hierarchical microspheres of BiOCl1−xBrx and BiOBrnI1−n exhibit adjustable band gaps and high degradation efficiencies for alkyl phenols and EDCs, with BiOBr0.75I0.25 showing superior performance and sustained activity over multiple cycles.176,177
4.3.4. Metal ions.
The global issue of heavy metal water contamination significantly threatens human health and the natural environment. Despite its known carcinogenic and mutagenic properties, hexavalent chromium (Cr(VI)) remains a key ingredient in various industrial processes, such as electroplating, leather tanning, and the production of anti-corrosion steel. Cr(III) ions are considered safe with relatively low toxicity compared to other chromium ions. Therefore, photocatalytic conversion of Cr(IV) to Cr(III) is imperative. Table 4 lists the preparation methods, contaminant dosage, percentage removal of metal ions, and reactive species involved in the reported solid solutions.
Table 4 Summary of the reported literature on the photocatalytic reduction of various metal ions on BiOY and BixOyYz solid solution
Solid solution material |
Synthesis method |
Contaminant & dosage |
Irradiation source |
Photocatalytic activity & active species |
Ref. |
BiOClxI1−x; BiOCl0.7I0.3 |
Solvothermal |
10 mg L−1 Cr(VI), 1 g L−1 |
Visible light |
∼100% in less than 30m min |
147
|
BiOBrxI1−x; BiOBr0.7I0.3 |
Bi(NO3)3·5H2O, ethylene glycol |
∼100% in less than 30m min |
BiOClxBr1−x; BiOCl0.3Br0.7 |
97.7% in 60 min |
Bi4O5BrxI2−x; Bi4O5BrI |
Hydrolytic |
60 mg L−1 Cr(VI), 0.4 g L−1 |
Visible light |
88% in 60 min higher than Bi4O5Br2 (47%) & Bi4O5I2 (53%) |
148
|
Bi(NO3)3·5H2O, glycerol |
BiOBrxI1−x; BiOBr0.75I0.25 |
Solvothermal |
10 mg L−1 Cr6+, 0.4 g L−1 |
Visible light |
12.2 & 5.1 times higher than BiOBr & BiOI, respectively |
166
|
Bi(NO3)3·5H2O, polyvinylpyrrolidone, ethylene glycol |
BiOClxBr1−x; BiOCl0.8Br0.2 |
Precipitation |
8 mg L−1 Cr(VI), 0.5 g L−1 |
Visible light |
BiOCl0.8Br0.2 showed higher photocatalytic activity than pure BiOCl or BiOBr |
178
|
Bi(NO3)3·5H2O, ethylene glycol, water |
BiOCl1−nIn; BiOCl0.6I0.4 |
Solvothermal |
35 mg L−1, Cr(VI) |
UV-vis |
87.7 in 35 min |
179
|
Bi(NO3)3·5H2O, glycerol, water |
Bai et al.148 highlighted the enhanced photocatalytic reduction of Cr(VI) by BiOBr0.75I0.25, attributed to band structure modification through iodine substitution for bromine, inducing defect states that facilitate electron transfer and reduce recombination.166 Hussain et al.178 demonstrated the superior photocatalytic activity of BiOCl0.8Br0.2 compared to that of pure BiOCl or BiOBr, owing to its larger surface area, microporous volume, and morphology, which provide additional active sites for Cr(VI) adsorption and charge carrier transport. Wang et al.179 introduced halogen vacancies into BiOCl1−xIx solid solutions, resulting in broadened band structures and the creation of active sites that significantly improved the reduction of Cr(VI) ions, supported by the extended optical absorption range and effective charge carrier separation within the solid solution.
4.3.5. Air purification.
Revolutionizing air purification through cutting-edge photocatalytic processes can enhance environmental sustainability and health. The breakdown of gaseous pollutants through photocatalysis primarily involves three pivotal stages: (i) separation and migration of charges, (ii) generation of reactive oxygen species (h+, ˙OH, and/or ˙O2−), and (iii) catalytic reduction reactions.180 BiOY and BixOyYz solid solution photocatalysts have recently garnered significant attention in the photoreduction of NOx, CO2, and other gases. Table 5 lists the preparation methods, contaminant dosage, percentage removal, and reactive species involved in the reported solid solutions.
Table 5 Summary of the reported literature on the photocatalytic degradation of various gaseous compounds on BiOY and BixOyYz solid solution
Solid solution material |
Synthesis method |
Contaminant & dosage |
Irradiation source |
Photocatalytic activity & active species |
Ref. |
Bi4O5BrxI2−x; Bi4O5BrI |
Hydrolytic |
CO2 |
Visible light |
0.372% in 240 min 3.65 & 2.72 time higher than Bi4O5Br2 (0.102%) and Bi4O5I2 (0.137%), respectively |
148
|
Bi(NO3)3·5H2O, glycerol |
BiOI0.25Cl0.75 |
Template free |
450 ppb NO |
Simulated solar light |
54.6% in 30 min 21 & 4 times higher than BiOCl & BiOI, respectively |
181
|
Bi(NO3)3·5H2O, water |
BiOClxBr1−x; BiOCl0.5Br0.5 |
Template free |
100 ppm NO |
Visible light |
57.3% in 40 min 3 & 19 times higher than BiOBr & BiOCl, respectively. ˙OH & ˙O2− |
182
|
Bi(NO3)3·5H2O, water |
Bi3O4ClxBr1−x; Bi3O4Cl0.5Br0.5 |
Solid state reaction |
600 ppb NO |
Simulated visible light |
60% in 10 min. Greater than Bi3O4Cl (25%) and Bi3O4Br (35%). h+ |
183
|
Bi(NO3)3·5H2O, Bi2O3, ethylene glycol |
(BiO)2(CO3)x(I2)1−x; (BiO)2(CO3)0.75(I2)0.25 |
Precipitation |
600 ppb NO |
Visible light |
50.8% in 30 min. 50 & 8 times higher than pure (BiO)2CO3 & BiOI, respectively |
184
|
Bi(NO3)3·5H2O, (BiO)2CO3, nitric acid |
BiOBrxCl1−x; BiOBr0.6Cl0.4 |
Precipitation |
CO2 reduction to CO and O2 |
Simulated solar light |
7.5 and 10.2 times higher than pure BiOCl & BiOBr, respectively |
185
|
Bi(NO3)3·5H2O, nitric acid |
Bai et al.148 emphasized the influence of conduction band position on the photocatalytic activity of bismuth-rich Bi4O5BrxI2−x solid solutions in achieving enhanced CO2 conversion rates. Dong et al.181 demonstrated efficient removal of NO gas using BiOI/BiOCl solid solutions, with BiOI0.25Cl0.75 exhibiting superior performance owing to its 3D flower-like morphology and high surface area. Wu et al.182 recorded significant oxidation of NO gas by a BiOCl0.5Br0.5 solid solution, which was attributed to its hierarchical nanostructures and modified band structures. Similarly, Bai et al.183 reported enhanced NO degradation by a Bi3O4Cl0.5Br0.5 solid solution owing to efficient charge separation. Ou et al.184 highlighted the remarkable NO degradation efficiency of a (BiO)2(CO3)0.75(I2)0.25 solid solution, attributed to its tailored band structure and hierarchical morphology. Gao et al.185 synthesized Persian buttercup-like BiOBr0.6Cl0.4, which exhibited notable CO2 photoreduction rates and outstanding stability over multiple cycles.
4.3.6. Other pollutants.
Contemporary environmental pollution from pollutants including formaldehyde, sodium pentachlorophenate, bacteria, acrylamide, naphthalene, and trace organic pollutants (TrOPs) presents significant challenges to both the environment and human health. Understanding their degradation mechanisms and assessing the efficacy of photocatalytic treatment is crucial for devising effective remediation strategies. Numerous studies have reported the degradation of these pollutants using photocatalysts based on BiOY and BixOyYz solid solutions.186 Despite employing different methodologies and materials, these studies aim to enhance the photocatalytic activity for environmental remediation. The removal of these other pollutants is summarized in Table 6.
Table 6 Summary of the reported literature on the photocatalytic degradation of various other pollutants on BiOY and BixOyYz solid solution
Solid solution material |
Synthesis method |
Contaminant & dosage |
Irradiation source |
Photocatalytic activity & active species |
Ref. |
BiOCl0.808Br0.192/alumina |
Hydrolysis & precipitation. Aluminium tri-sec-butoxide, water Bi(NO3)3·5H2O, acetic acid |
25 mg L−1 naphthalene |
Visible light |
∼100% in 60 min |
159
|
Ag–AgI/AgCl/Bi3O4Cl0.5Br0.5 |
Photo-reduction |
10 mg L−1 acrylamide, 0.5 g L−1 |
UV light |
41.0% in 300 min 3.1 & 1.6 times higher than Bi3O4Cl0.5Br0.5 & Ag–AgI/AgCl, respectively |
175
|
Bi(NO3)3·5H2O, AgNO3, Bi2O3, absolute ethanol |
Ag–AgCl0.5Br0.5/BiOCl0.5Br0.5 |
Solvothermal |
10 mg L−1 acrylimide, 0.5 g L−1 |
Visible light |
75.63% in 300 min ˙O2−, & h+ |
174
|
Bi(NO3)3·5H2O, AgNO3, ethylene glycol |
BiOBrxI1−x; BiOBr0.75I0.25 |
Precipitation |
109 CFU mL−1Escherichia coli, 15 mg L−1 |
Visible light |
∼100% in 10 min |
186
|
Bi(NO3)3·5H2O, acetic acid |
106 CFU mL−1Bacillus subtilis, 10 mg L−1 |
∼100% in 120 min |
3% Bi(0)-doped BiOCl0.875Br0.125 |
Precipitation |
100 μg L−1 TrOPs (CBZ, VLX, & BZF) |
Solar simulator |
Degradation followed the order BZF > VLX > CBZ |
187
|
Bi(NO3)3·5H2O, acetic acid, water, CTAB |
BiOCl0.95Br0.05 |
Hydrothermal |
15 mg L−1 PCPNa, 1 g L−1 |
UV light |
|
188
|
Bi(NO3)3·5H2O, water |
BiOClxBr1−x; 3% Bi-doped BiOCl0.875Br0.125 |
Precipitation |
Chlorobenzene, benzene, toluene, xylene, 0.75 g L−1 |
Visible light |
Fully decomposed to CO2 & H2O |
189
|
Bi(NO3)3·5H2O, acetic acid, sodium borohydride |
Highly stable composite films (BiOCl0.808Br0.192/alumina) achieved complete naphthalene degradation within 60 min.159 The development of BiOY nanostructures (Ag–AgCl0.5Br0.5/BiOCl0.5Br0.5 and Ag–AgI/AgCl/Bi3O4Cl0.5Br0.5) has expanded applications to acrylamide and bisphenol A degradation, with elemental bismuth doping enhancing organic contaminant mineralization.174,175 Dandapat et al.187 engineered Bi(0)-doped BiOY solid solution films, notably 3% Bi(0)-doped BiOCl0.875Br0.125, exhibiting enhanced TrOPs degradation due to ion-exchange adsorption mechanisms. Similarly, UV light-induced OVs on BiOCl0.95Br0.05-010-OVs enhanced PCPNa degradation and remained stable over time for long-term applications.188 Controlled doping of BiOClxBr1–x solid solution with elemental bismuth particles enhances the mineralization of various pollutants, fully decomposing toluene, chlorobenzene, xylene, and benzene into CO2 and water.189 The BiOBr0.75I0.25 nanostructures displayed high bactericidal activity, suggesting potential beyond photocatalysis for environmental challenges.186
5. Challenges and recommendations
Although BiOY and BixOyYz photocatalysts have been extensively studied, there remain some inherent challenges that should be addressed for the full exploitation of these materials in various fields.
1. Optimizing the synthesis methods for practical scalability is crucial. The current laboratory-scale synthesis methods for BiOY and BixOyYz photocatalysts involve multistep reactions with low yields and long reaction times. It is essential to develop simpler, more efficient, and scalable methods suitable for commercial applications.
2. Expanding the applications for energy conversion is a priority. BiOY and BixOyYz photocatalysts are primarily used for organic contaminant degradation and heavy metal removal, with limited research on energy conversion processes, such as CO2 reduction and water splitting. Enhancing the photocatalytic efficiency, stability, and bandgap adjustment can broaden their applicability in energy-related fields.
3. Enhancing recyclability and understanding environmental impacts are key considerations. Recyclability is essential for the practical application of BiOY and BixOyYz, ensuring greenness, safety, and economic feasibility. Assessing the environmental impact of the synthesis and modification processes is necessary, including the evaluation of potential ecological risks and ecotoxicological effects.
4. Clarifying the reaction mechanisms and exploring theoretical simulations are critical. The exact reaction mechanisms of BiOY and BixOyYz and their nanocomposites remain unclear, particularly in multi-step reactions and electron transfer processes. Utilizing in situ spectroscopy and theoretical simulations, such as DFT can deepen our understanding of structure–activity relationships and reaction pathways.
5. A notable challenge identified in the reviewed studies is the need for reported degradation products. Despite the extensive research on environmental pollutants, many studies need to provide comprehensive information regarding the degradation by-products, hindering a thorough understanding of the pollutant's fate and potential environmental impact.
6. Conclusions and perspectives
The distinctive layered structure and adjustable electronic properties of BiOX and BixOyYz make them promising candidates for environmental remediation. Pure BiOX and BixOyYz face inherent limitations, such as inadequate light absorption, insufficient surface-active sites, and an inclination for fast recombination of e−/h+ pairs. Many optimized structural strategies have emerged to mitigate these environmental challenges, ushering in a new realm of possibilities for enhancing photocatalytic performance. The improvement of photocatalytic efficiency in pure BiOY and BixOyYz is primarily achieved through solid solution formation, which involves adjusting the energy band structure and influencing carrier dynamics. The photocatalytic efficacy of BiOY and BixOyYz solid solutions depends on their specific compositions. Fine-tuning of the energy band structure was accomplished by modifying the halogen composition. With an increase in the proportion of halogens possessing more significant atomic numbers, the VB position progressively increases, constricting the bandgap width of the catalyst and substantially augmenting light absorption. This results in a more substantial generation of photogenerated carriers. Conversely, the elevated VB position diminishes the redox capability of the charge carriers.
Moreover, solid solution-based materials derived from BiOY and BixOyYz have found applications in the removal of dyes, phenolic compounds, metal ions, pharmaceuticals, and air (NO2 and CO2) reduction, showing commendable photocatalytic efficacy and operational stability. Enhanced characterization methodologies hold the promise of offering novel insights into structural evolution and gaining a comprehensive understanding of photocatalytic processes. Currently, characterization techniques predominantly rely on ex situ methods.
BiOY and BixOyYz stand out as exceptional layered materials characterized by outstanding photochemical attributes with immense promise for significant advancements in diverse environmental applications. However, evaluating BiOY and BixOyYz-based photodegradation processes predominantly focuses on aqueous solutions, leaving a notable absence of studies concerning gaseous pollutants, particularly volatile organic compounds. Further, there is a pressing need to develop robust methodologies for monitoring the toxicity of photocatalytic end products during the treatment of complex organic contaminants to mitigate the risk of secondary pollution. Lastly, this review has shed light on improving the intrinsic properties of BiOY and BixOyYz-based solid solutions. Such insights are expected to propel and diversify the applications of bismuth-based photocatalysts.
Data availability
This review paper synthesizes data from previously published studies. No new data were generated or analyzed in this study. All data supporting the findings of this review are available from the corresponding publications cited in the reference section. The datasets analyzed during the review are publicly accessible and can be found in the respective repositories as mentioned in the original studies.
Author contributions
Robert O. Gembo: conceptualization, methodology, data collection, data analysis, data curation, writing – original draft; Rudzani Ratshiedana: writing – review and editing; Alex T. Kuvarega: writing – review and editing; Lawrence M. Madikizela: supervision, writing – review and editing; I. Kamika: supervision, writing – review and editing; Cecil K. King'ondu: writing – review and editing; and Titus A. M. Msagati: conceptualization, resources, funding, supervision, writing – review and editing.
Conflicts of interest
The authors declare that they have no known competing financial interests or personal relationships that could have influenced the work reported in this study.
Acknowledgements
The authors would like to express their sincere gratitude to Dr Orlette Mkhari for his invaluable contributions to the manuscript. Additionally, they acknowledge the Institute for Nanotechnology and Water Sustainability and University of South Africa (UNISA) for administrative support.
References
- K. A. Adegoke, O. R. Adegoke, R. A. Adigun, N. W. Maxakato and O. S. Bello, Two-dimensional metal-organic frameworks: From synthesis to biomedical, environmental, and energy conversion applications, Coord. Chem. Rev., 2022, 473, 214817 CrossRef CAS.
- J. Z. Hassan, A. Raza, U. Qumar and G. Li, Recent advances in engineering strategies of Bi-based photocatalysts for environmental remediation, Sustainable Mater. Technol., 2022, 33, e00478 CrossRef CAS.
- A. Kumar, G. Sharma, Mu. Naushadd, A. H. Al-Muhtaseb, A. García-Peñas, G. T. Molaf, C. Si and F. J. Stadler, Bio-inspired and biomaterials-based hybrid photocatalysts for environmental detoxification: A review, Chem. Eng. J., 2020, 382, 122937 CrossRef CAS.
- R. O. Gembo, O. Aoyi, S. Majoni, A. Etale, S. Odisitse and C. K. King'ondu, Synthesis of bismuth oxyhalide (BiOBrzI(1-z)) solid solutions for photodegradation of methylene blue dye, AAS Open Res., 2022, 4, 43 Search PubMed.
- J. Sharma, P. Dhiman, R. A. Alshgari, Z. A. ALOthman, A. Kumar, G. Sharma and G. Rana, Advances in photocatalytic environmental and clean energy applications of bismuth-rich oxy halides-based heterojunctions: a review, Mater. Today Sustain., 2023, 21, 100327 Search PubMed.
- N. Tian, C. Hu, J. Wang, Y. Zhang, T. Ma and H. Huang, Layered bismuth-based photocatalysts, Coord. Chem. Rev., 2022, 463, 214515 CrossRef CAS.
- A. Fujishima and K. Honda, Electrochemical Photolysis of Water at a Semiconductor Electrode, Nature, 1972, 238(5358), 37–38 CrossRef CAS.
- R. Asahi, T. Morikawa, H. Irie and T. Ohwaki, Nitrogen-doped titanium dioxide as visible-light-sensitive photocatalyst: Designs, developments, and prospects, Chem. Rev., 2014, 114(19), 9824–9852 CrossRef CAS.
- A. Kudo and Y. Miseki, Heterogeneous photocatalyst materials for water splitting, Chem. Soc. Rev., 2009, 38(1), 253–278 RSC.
- P. Zhang, J. Zhang and J. Gong, Tantalum-based semiconductors for solar water splitting, Chem. Soc. Rev., 2014, 43(13), 4395–4422 RSC.
- G. Liu, P. Niu and H. M. Cheng, Visible-light-active elemental photocatalysts, ChemPhysChem, 2013, 14(5), 885–892 CrossRef CAS.
- N. Zhang, R. Ciriminna, M. Pagliaro and Y. J. Xu, Nanochemistry-derived Bi2WO6 nanostructures: Towards production of sustainable chemicals and fuels induced by visible light, Chem. Soc. Rev., 2014, 43(15), 5276–5287 RSC.
- L. Zhang and Y. Zhu, A review of controllable synthesis and enhancement of performances of bismuth tungstate visible-light-driven photocatalysts, Catal. Sci. Technol., 2012, 2(4), 694–706 RSC.
- J. Yang, Z. Huang, J. Li, Y. Yao, Y. Meng, B. Xie, Z. Ni and S. Xia, Photocatalytic reduction of nitrogen to ammonia by bismuth oxyhalides containing oxygen vacancies, Colloids Surf., A, 2023, 662(11), 130995 CrossRef CAS.
- Y. Sun, Y. Ahmadi, K. H. Kim and J. Lee, The use of bismuth-based photocatalysts for the production of ammonia through photocatalytic nitrogen fixation, Renewable Sustainable Energy Rev., 2022, 170(6), 112967 CrossRef CAS.
- M. Arumugam, T. S. Natarajan, T. Saelee, S. Praserthdam, M. Ashokkumar and P. Praserthdam, Recent developments on bismuth oxyhalides (BiOX; X = Cl, Br, I) based ternary nanocomposite photocatalysts for environmental applications, Chemosphere, 2021, 282(6), 131054 CrossRef CAS.
- Z. Mengting, T. A. Kurniawan, L. Duan, Y. Song, S. W. Hermanowicz and M. H. D. Othman, Advances in BiOX-based ternary photocatalysts for water technology and energy storage applications: Research trends, challenges, solutions, and ways forward, Rev. Environ. Sci. Biotechnol., 2022, 21, 331–370 CrossRef.
- S. Liu, J. Sun, G. Ren and X. Meng, Vacancy-engineered bismuth-based semiconductor with enhanced photocatalytic activity: A review, Mater. Sci. Semicond. Process., 2022, 137, 106230 CrossRef CAS.
- Z. Wang, M. Chen, D. Huang, G. Zeng, P. Xu, C. Zhou, H. Lai, M. Wang and W. W. Cheng, Multiply structural optimized strategies for bismuth oxyhalide photocatalysis and their environmental application, Chem. Eng. J., 2019, 374(6), 1025–1045 CrossRef CAS.
- I. Ahmad, S. Shukrullah, M. Yasin Naz, S. Ullah and A. M. Ali, Designing and modification of bismuth oxyhalides BiOX (X = Cl, Br and I) photocatalysts for improved photocatalytic performance, J. Ind. Eng. Chem., 2022, 105(9), 1–33 CAS.
- J. Xiong, P. Song, J. Di and H. Li, Bismuth-rich bismuth oxyhalides: A new opportunity to trigger high-efficiency photocatalysis, J. Mater. Chem. A, 2020, 8(41), 21434–21454 RSC.
- L. Wang, L. Wang, Y. Du, X. Xu and S. X. Dou, Progress and perspectives of bismuth oxyhalides in catalytic applications, Mater. Today Phys., 2021, 16, 100294 CrossRef CAS.
- A. Malathi, J. Madhavan, M. Ashokkumar and P. Arunachalam, A review on BiVO4 photocatalyst: Activity enhancement methods for solar photocatalytic applications, Appl. Catal., A, 2018, 555, 47–74 CrossRef CAS.
- G. A. Kallawar, D. P. Barai and B. A. Bhanvase, Bismuth titanate based photocatalysts for degradation of persistent organic compounds in wastewater: A comprehensive review on synthesis methods, performance as photocatalyst and challenges, J. Cleaner Prod., 2021, 318, 128563 CrossRef CAS.
- A. Elaouni, M. El Ouardi, A. BaQais, M. Arab, M. Saadi and H. Ait Ahsaine, Bismuth tungstate Bi2WO6: a review on structural, photophysical and photocatalytic properties, RSC Adv., 2023, 13(26), 17476–17494 RSC.
- M. Shetty, K. Manickavasakam, N. Kulal, P. D. Shivaramu, M. N. Rani, M. Shastri, M. Murthy, S. J. Babu, G. V. Shanbhag, M. Sathish and D. Rangapp, Bismuth oxycarbonate Nanoplates@α-Ni(OH)2 nanosheets 2D plate-on-sheet heterostructure as electrode for high-performance supercapacitor, J. Alloys Compd., 2021, 860, 158495 CrossRef CAS.
- H. R. Mahmoud, Bismuth silicate (Bi4Si3O12 and Bi2SiO5) prepared by ultrasonic-assisted hydrothermal method as novel catalysts for biodiesel production via oleic acid esterification with methanol, Fuel, 2019, 256, 115979 CrossRef CAS.
- N. Wang, X. Luo, L. Han, Z. Zhang, R. Zhang, H. Olin and Y. Yang, Structure, Performance, and Application of BiFeO3 Nanomaterials, Nano-Micro Lett., 2020, 12(1), 81 CrossRef CAS.
- G. Yang, Y. Liang, K. Li, J. Yang, K. Wang, R. Xu and X. Xie, Engineering the dimension and crystal structure of bismuth molybdate photocatalysts via a molten salt-assisted assembly approach, J. Alloys Compd., 2020, 844, 156231 CrossRef CAS.
- Y. Liu, Z. Zhu, Y. Liu, J. Wu, Y. Ling, Z. Xiang, S. Qin, Y. Ye and M. Bai, First principles insight on enhanced photocatalytic performance of sulfur-doped bismuth oxide iodate, Mater. Sci. Semicond. Process., 2023, 165, 107672 CrossRef CAS.
- M. Kuznetsova, S. A. A. Oliveira, B. S. Rodrigues and J. S. Souza, Microwave-Assisted Synthesis of Bismuth Niobate/Tungsten Oxide Photoanodes for Water Splitting, Top. Catal., 2021, 64(13–16), 748–757 CrossRef CAS.
- R. Kumar, P. Raizada, A. A. P. Khan, V. H. Nguyen, Q. Van Le, S. Ghotekar, R. Selvasembian, V. Gandhi, A. Singh and P. Singh, Recent progress in emerging BiPO4-based photocatalysts: Synthesis, properties, modification strategies, and photocatalytic applications, J. Mater. Sci. Technol., 2022, 108, 208–225 CrossRef CAS.
- R. He, D. Xu, B. Cheng, J. Yu and W. Ho, Review on nanoscale Bi-based photocatalysts, Nanoscale Horiz., 2018, 3(5), 464–504 RSC.
- J. Di, C. Zhu, M. Ji, M. Duan, R. Long, C. Yan, K. Gu, J. Xiong, Y. She, J. Xia, H. Li and Z. Liu, Defect-Rich Bi12O17Cl2 Nanotubes Self-Accelerating Charge Separation for Boosting Photocatalytic CO2 Reduction, Angew. Chem., Int. Ed., 2018, 57(45), 14847–14851 CrossRef CAS.
- P. Gao, Y. Yang, Z. Yin, F. Kang, W. Fan, J. Sheng, L. Feng, Y. Liu, Z. Du and L. Zhang, A critical review on bismuth oxyhalide based photocatalysis for pharmaceutical active compounds degradation: Modifications, reactive sites, and challenges, J. Hazard. Mater., 2021, 412, 125186 CrossRef CAS.
- X. Wei, M. U. Akbar, A. Raza and G. Li, A review on bismuth oxyhalide based materials for photocatalysis, Nanoscale Adv., 2021, 3(12), 3353–3372 RSC.
- J. Zhao, Z. Miao, Y. Zhang, G. Wen, L. Liu, X. Wang, X. Cao and B. Wang, Oxygen vacancy-rich hierarchical BiOBr hollow microspheres with dramatic CO2 photoreduction activity, J. Colloid Interface Sci., 2021, 593, 231–243 CrossRef CAS.
- X. Ren, M. Gao, Y. Zhang, Z. Zhang, X. Cao, B. Wang and X. Wang, Photocatalytic reduction of CO2 on BiOX: Effect of halogen element type and surface oxygen vacancy mediated mechanism, Appl. Catal., B, 2020, 274, 119063 CrossRef CAS.
- J. Di, C. Chen, C. Zhu, P. Song, J. Xiong, M. Ji, J. Zhou, Q. Fu, M. Xu, W. Hao, J. Xia, S. Li, H. Li and Z. Liu, Bismuth Vacancy-Tuned Bismuth Oxybromide Ultrathin Nanosheets toward Photocatalytic CO2 Reduction, ACS Appl. Mater. Interfaces, 2019, 11(34), 30786–30792 CrossRef CAS.
- H. Yu and Q. Han, Effect of reaction mediums on photocatalytic performance of BiOX (X = Cl, Br, I), Opt. Mater., 2021, 119, 111399 CrossRef CAS.
- Y. Huang, H. Li, M. S. Balogun, W. Liu, Y. Tong, X. Lu and H. Ji, Oxygen vacancy induced bismuth oxyiodide with remarkably increased visible-light absorption and superior photocatalytic performance, ACS Appl. Mater. Interfaces, 2014, 6(24), 22920–22927 CrossRef CAS.
- S. Weng, Z. Fang, Z. Wang, Z. Zheng, W. Feng and P. Liu, Construction of teethlike homojunction BiOCl (001) nanosheets by selective etching and its high photocatalytic activity, ACS Appl. Mater. Interfaces, 2014, 6(21), 18423–18428 CrossRef CAS.
- H. Huang, X. Han, X. Li, S. Wang, P. K. Chu and Y. Zhang, Fabrication of multiple heterojunctions with tunable visible-light-active photocatalytic reactivity in BiOBr-BiOI full-range composites based on microstructure modulation and band structures, ACS Appl. Mater. Interfaces, 2015, 7(1), 482–492 CrossRef CAS.
- Z. K. Tang, W. J. Yin, L. Zhang, B. Wen, D. Y. Zhang, L. M. Liu and W. M. Lau, Spatial separation of photo-generated electron-hole pairs in BiOBr/BiOI bilayer to facilitate water splitting, Sci. Rep., 2016, 6, 1–9 CrossRef.
- C. Lai, M. Zhang, B. Li, D. Huang, G. Zeng, L. Qin, X. Liu, H. Yi, M. Cheng, L. Li, Z. Chen and L. Chen, Fabrication of CuS/BiVO4 (0 4 0) binary heterojunction photocatalysts with enhanced photocatalytic activity for Ciprofloxacin degradation and mechanism insight, Chem. Eng. J., 2019, 358, 891–902 CrossRef CAS.
- R. Dong, Y. Hu, Y. Wu, W. Gao, B. Ren, Q. Wang and Y. Cai, Visible-light-driven BiOI-based janus micromotor in pure water, J. Am. Chem. Soc., 2017, 139(5), 1722–1725 CrossRef CAS.
- D. Kandi, S. Martha, A. Thirumurugan and K. M. Parida, Modification of BiOI Microplates with CdS QDs for Enhancing Stability, Optical Property, Electronic Behavior toward Rhodamine B Decolorization, and Photocatalytic Hydrogen Evolution, J. Phys. Chem. C, 2017, 121(9), 4834–4849 CrossRef CAS.
- J. Hu, W. Fan, W. Ye, C. Huang and X. Qiu, Insights into the photosensitivity activity of BiOCl under visible light irradiation, Appl. Catal., B, 2014, 158–159, 182–189 CrossRef CAS.
- S. O. Ganiyu, C. A. Martínez-Huitle and M. A. Rodrigo, Renewable energies driven electrochemical wastewater/soil decontamination technologies: A critical review of fundamental concepts and applications, Appl. Catal., B, 2020, 270, 118857 CrossRef CAS.
- A. Sudhaik, P. Raizada, P. Shandilya, D. Y. Jeong, J. H. Lim and P. Singh, Review on fabrication of graphitic carbon nitride based efficient nanocomposites for photodegradation of aqueous phase organic pollutants, J. Ind. Eng. Chem., 2018, 67, 28–51 CrossRef CAS.
- K. L. Zhang, C. M. Liu, F. Q. Huang, C. Zheng and W. D. Wang, Study of the electronic structure and photocatalytic activity of the BiOCl photocatalyst, Appl. Catal., B, 2006, 68, 125–129 CrossRef CAS.
- J. Bai, J. Sun, X. Zhu, J. Liu, H. Zhang, X. B. Yin and L. Liu, Enhancement of Solar-Driven Photocatalytic Activity of BiOI Nanosheets through Predominant Exposed High Energy Facets and Vacancy Engineering, Small, 2020, 16(5), 1904783 CrossRef CAS.
- Z. Luo, X. Ye, S. Zhang, S. Xue, C. Yang, Y. Hou, W. Xing, R. Yu, J. Sun, Z. Yu and X. Wang, Unveiling the charge transfer dynamics steered by built-in electric fields in BiOBr photocatalysts, Nat. Commun., 2022, 13(1), 1–10 Search PubMed.
- M. Guan, C. Xiao, J. Zhang, S. Fan, R. An, Q. Cheng, J. Xie, M. Zhou, B. Ye and V. Xie, Vacancy associates promoting solar-driven photocatalytic activity of ultrathin bismuth oxychloride nanosheets, J. Am. Chem. Soc., 2013, 135(28), 10411–10417 CrossRef CAS.
- L. Sun, L. Xiang, X. Zhao, C. J. Jia, J. Yang, Z. Jin, X. Cheng and W. Fan, Enhanced visible-light photocatalytic activity of BiOI/BiOCl heterojunctions: Key role of crystal facet combination, ACS Catal., 2015, 5(6), 3540–3551 CrossRef CAS.
- X. J. Guo, M. Zhen, H. Liu and L. Liu, BiOBr-BiOI microsphere assembled with atom-thick ultrathin nanosheets and its high photocatalytic activity, RSC Adv., 2015, 5(31), 24777–24782 RSC.
- J. Jiang, K. Zhao, X. Xiao and L. Zhang, Synthesis and facet-dependent photoreactivity of BiOCl single-crystalline nanosheets, J. Am. Chem. Soc., 2012, 134(10), 4473–4476 CrossRef CAS.
- Z. Fan, Y. Zhao, W. Zhai, L. Qiu, H. Li and M. R. Hoffmann, Facet-dependent performance of BiOBr for photocatalytic reduction of Cr(VI), RSC Adv., 2016, 6(3), 2028–2031 RSC.
- L. Ye, X. Jin, C. Liu, C. Ding, H. Xie, K. H. Chu and P. K. Wong, Thickness-ultrathin and bismuth-rich strategies for BiOBr to enhance photoreduction of CO2 into solar fuels, Appl. Catal., B, 2016, 187, 281–290 CrossRef CAS.
- L. Wang, J. Shang, W. Hao, S. Jiang, S. Huang, T. Wang, Z. Sun, Y. Du, S. Dou, T. Xie, D. Wang and J. Wang, A dye-sensitized visible light photocatalyst-Bi24O31Cl10, Sci. Rep., 2014, 4, 7384 CrossRef.
- J. Li, H. Li, G. Zhan and L. Zhang, Solar water splitting and nitrogen fixation with layered bismuth oxyhalides, Acc. Chem. Res., 2017, 50(1), 112–121 CrossRef CAS.
- X. Lin, H. Tao, H. Fuqiang, W. Wendeng and S. Jianlin, Photocatalytic activity of a Bi-based oxychloride Bi3O4Cl, J. Phys. Chem. B, 2006, 110(48), 24629–24634 CrossRef CAS.
- X. Jin, L. Ye, H. Xie and G. Chen, Bismuth-rich bismuth oxyhalides for environmental and energy photocatalysis, Coord. Chem. Rev., 2017, 349(9), 84–101 CrossRef CAS.
- A. Hussain, J. Hou, M. Tahir, S. S. Ali, Z. U. Rehman, M. Bilal, T. Zhang, Q. Dou and X. Wang, Recent advances in BiOX-based photocatalysts to enhanced efficiency for energy and environment applications, Catal. Rev.: Sci. Eng., 2024, 66(1), 119–173 CrossRef CAS.
- Y. L. Qi, G. Han and X. C. Song, Enhanced photocatalytic degradation of phenol over Ag3PO4-BiOCl1−xBrx composites, Mater. Res. Bull., 2018, 102, 16–23 CrossRef CAS.
- J. Wang, H. Li, X. Yan, C. Qian, Y. Xing, S. Yang, Z. Kang, J. Han, W. Gu, H. Yang and F. Xiao, Synergistic enhancement of the visible-light photocatalytic activity of hierarchical 3D BiOClxBr1-x/graphene oxide heterojunctions for formaldehyde degradation at room temperature, J. Alloys Compd., 2019, 795, 120–133 CrossRef CAS.
- H. Huang, C. Zeng, K. Xiao and Y. Zhang, Coupling of solid-solution and heterojunction in a 2D-1D core-shell-like BiOCl0.5I0.5/Bi5O7I hierarchy for promoting full-spectrum photocatalysis and molecular oxygen activation, J. Colloid Interface Sci., 2017, 504, 257–267 CrossRef CAS.
- Y. Wang, J. Meng, S. Jing, K. Wang, C. Ban, Y. Feng, Y. Duan, J. Ma, L. Gan and V. Zhou, Origin of Bismuth-Rich Strategy in Bismuth Oxyhalide Photocatalysts, Energy Environ. Mater., 2023, 6(5), 2–9 Search PubMed.
- C. Y. Wang, X. Zhang and H. Q. Yu, Bismuth oxyhalide photocatalysts for water purification: Progress and challenges, Coord. Chem. Rev., 2023, 493(7), 215339 CrossRef CAS.
- V. Charles, I. P. Ebuka and N. A. Gaya, Solid-Solutions as Supports and Robust Photocatalysts and Electrocatalysts: A Review, Catal. Sustainable Energy, 2020, 7, 8–28 CAS.
-
R. Li and C. Li, Photocatalytic Water Splitting on Semiconductor-Based Photocatalysts, Advances in Catalysis, Elsevier Inc., 1st edn, 2017, vol. 60, pp. 1–57 Search PubMed.
- H. Shi, W. Ming and M. H. Du, Bismuth chalcohalides and oxyhalides as optoelectronic materials, Phys. Rev. B, 2016, 93(10), 104108 CrossRef.
- D. Gogoi, A. K. Shah, M. Qureshi, A. K. Golder and N. R. Peela, Silver grafted graphitic-carbon nitride ternary hetero-junction Ag/gC3N4(Urea)-gC3N4(Thiourea) with efficient charge transfer for enhanced visible-light photocatalytic green H2 production, Appl. Surf. Sci., 2021, 558(2), 149900 CrossRef CAS.
- J. Di, J. Xia, H. Li, S. Guo and S. Dai, Bismuth oxyhalide layered materials for energy and environmental applications, Nano Energy, 2017, 41, 172–192 CrossRef CAS.
- R. Marschall, Semiconductor composites: Strategies for enhancing charge carrier separation to improve photocatalytic activity, Adv. Funct. Mater., 2014, 24(17), 2421–2440 CrossRef CAS.
- R. Pode, Organic light emitting diode devices: An energy efficient solid state lighting for applications, Renewable Sustainable Energy Rev., 2020, 133, 110043 CrossRef.
- D. Zhang, G. Li, H. Li and Y. Lu, The development of better photocatalysts through composition- and structure-engineering, Chem. – Asian J., 2013, 8, 26–40 CrossRef CAS.
- H. Y. Xu, X. Han, Q. Tan, X. L. He and S. Y. Qi, Structure-dependent photocatalytic performance of BiOBrxI1-x nanoplate solid solutions, Catalysts, 2017, 7(5), 153 CrossRef.
- H. Zhang, L. Liu and Z. Zhou, Towards better photocatalysts: First-principles studies of the alloying effects on the photocatalytic activities of bismuth oxyhalides under visible light, Phys. Chem. Chem. Phys., 2012, 14(3), 1286–1292 RSC.
- Y. Liu, W. J. Son, J. Lu, B. Huang, Y. Dai and M. H. Whangbo, Composition dependence of the photocatalytic activities of BiOCl1-xBrx solid solutions under visible light, Chem. – Eur. J., 2011, 17(34), 9342–9349 CrossRef CAS.
- S. Ouyang and J. Ye, β-AgAl1-xGaxO2 solid-solution photocatalysts: Continuous modulation of electronic structure toward high-performance visible-light photoactivity, J. Am. Chem. Soc., 2011, 133(20), 7757–7763 CrossRef CAS.
- Q. Wang, Z. Liu, D. Liu, G. Liu, M. Yang, F. Cui and W. Wang, Ultrathin two-dimensional BiOBrxI1-x solid solution with rich oxygen vacancies for enhanced visible-light-driven photoactivity in environmental remediation, Appl. Catal., B, 2018, 236, 222–232 CrossRef CAS.
- H. Gnayem and Y. Sasson, Hierarchical nanostructured 3D flowerlike BiOClxBr1-x semiconductors with exceptional visible light photocatalytic activity, ACS Catal., 2013, 3(2), 186–191 CrossRef CAS.
- J. Yang, Y. Liang, K. Li, Y. Zhu, S. Liu, R. Xu and W. Zhous, Design of 3D flowerlike BiOClxBr1-x nanostructure with high surface area for visible light photocatalytic activities, J. Alloys Compd., 2017, 725, 1144–1157 CrossRef CAS.
- S. Lu, J. Li, F. Duan, L. Duan, M. Du and M. Chen, One-step preparation of Bi4O5BrxI2−x solid solution with superior photocatalytic performance for organic pollutants degradation under visible light, Appl. Surf. Sci., 2019, 475, 577–586 CrossRef CAS.
- S. Meng, Y. Bi, T. Yan, Y. Zhang, T. Wu, Y. Shao, D. Wei and B. Du, Room-temperature fabrication of bismuth oxybromide/oxyiodide photocatalyst and efficient degradation of phenolic pollutants under visible light, J. Hazard. Mater., 2018, 358, 20–32 CrossRef CAS.
- Y. Bai, X. Shi, P. Wang, L. Wang, H. Xie, Z. Li, L. Qu and L. Ye, Synthesis of one-dimensional Bi5O7Br0.5I0.5 solid solution for effective real oilfield wastewater treatment via exciton photocatalytic process, J. Taiwan Inst. Chem. Eng., 2018, 91, 358–368 CrossRef CAS.
- Z. Liu, J. Niu, P. Feng and Y. Zhu, Solvothermal synthesis of Bi24O31ClxBr10-x solid solutions with enhanced visible light photocatalytic property, Ceram. Int., 2015, 41(3), 4608–4615 CrossRef CAS.
- Y. D. Sun, C. Zeng, X. Zhang, Z. Q. Zhang, B. Yang and S. Q. Guo, Tendencies of alloyed engineering in BiOX-based photocatalysts: a state-of-the-art review, Rare Met., 2024, 43, 1488–1512 CrossRef CAS.
- J. Xie, Y. Cao, D. Jia and Y. Li, Dahlia-shaped BiOClxI1−x structures prepared by a facile solid-state method:
Evidence and mechanism of improved photocatalytic degradation of rhodamine B dye, J. Colloid Interface Sci., 2017, 503(5), 115–123 CrossRef CAS.
- A. Al-Keisy, R. Mahdi, D. Ahmed, K. Al-Attafi and W. H. Wan, Enhanced Photoreduction Activity in BiOI1-xFx Nanosheet for Efficient Removal of Pollutants from Aqueous Solution, ChemistrySelect, 2020, 5, 9758–9764 CrossRef CAS.
- L. Zhang, F. Liu, X. Xiao, X. Zuo and J. Nan, Microwave synthesis of iodine-doped bismuth oxychloride microspheres for the visible light photocatalytic removal of toxic hydroxyl-contained intermediates of parabens: catalyst synthesis, characterization, and mechanism insight, Environ. Sci. Pollut. Res., 2019, 26(28), 28871–28883 CrossRef CAS.
- H. Guo, S. Wang, L. Li, Y. Zhang, W. Wang and Q. Sun, Facile fabrication of BiOBrxCl1-x hierarchical microspheres photocatalysts for efficient degradation of diverse pollutants under visible light, J. Mol. Struct., 2022, 1265, 133359 CrossRef CAS.
- H. Y. Xu, X. Han, Q. Tan, K. J. Wu and S. Y. Qi, Crystal-chemistry insight into the photocatalytic activity of BiOClxBr1−x nanoplate solid solutions, Front. Mater. Sci., 2017, 11(2), 120–129 CrossRef.
- Z. Jia, F. Wang, F. Xin and B. Zhang, Simple solvothermal routes to synthesize 3D BiOBrxI1-x microspheres and their visible-light-induced photocatalytic properties, Ind. Eng. Chem. Res., 2011, 50(11), 6688–6694 CrossRef CAS.
- X. Wang, W. Bi, P. Zhai, X. Wang, H. Li, G. Mailhot and W. Dong, Adsorption and photocatalytic degradation of pharmaceuticals by BiOClxIy nanospheres in aqueous solution, Appl. Surf. Sci., 2016, 360, 240–251 CrossRef CAS.
- H. Huang, X. Li, X. Han, N. Tian, Y. Zhang and T. Zhang, Moderate band-gap-broadening induced high separation of electron–hole pairs in Br substituted BiOI: a combined experimental and theoretical investigation, Phys. Chem. Chem. Phys., 2015, 17, 3673–3679 RSC.
- X. Ren, J. Li, X. Cao, B. Wang, Y. Zhang and Y. Wei, Synergistic effect of internal electric field and oxygen vacancy on the photocatalytic activity of BiOBrxI1−x with isomorphous fluorine substitution, J. Colloid Interface Sci., 2019, 554, 500–511 CrossRef CAS.
- B. Yin and C. Liu, Convenient Synthesis and Enhanced Photocatalytic Activity of BiOI/BiOBr Nanostructures with Different Morphologies, J. Nanosci. Nanotechnol., 2017, 18(7), 4771–4779 CrossRef.
- Z. Zhang, Y. Zhao, J. Shen, Z. Pan, Y. Guo, P. K. Wong and H. Yu, Synthesis of 1D Bi12O17ClxBr2−x nanotube solid solutions with rich oxygen vacancies for highly efficient removal of organic pollutants under visible light, Appl. Catal., B, 2020, 269, 118774 CrossRef CAS.
- B. Luo, G. Liu and L. Wang, Recent advances in 2D materials for photocatalysis, Nanoscale, 2016, 8, 6904–6920 RSC.
- B. Zhang, J. Zhang, R. Duan, Q. Wan and X. Tan, Nano Energy BiOCl nanosheets with periodic nanochannels for high-efficiency photooxidation, Nano Energy, 2020, 78, 105340 CrossRef CAS.
- J. Fortner, L. Yang and P. Banerjee, Highly Conducting, n-Type Bi12O15Cl6 Nanosheets with Superlattice- like Structure, Chem. Mater., 2015, 27, 7710–7718 CrossRef.
- L. N. Thi, H. Tran Huu, T. N. Ngoc, N. S. M. Viswanath, H. T. T. Le, T. T. T. Phan, L. T. Nguyen, Q. T. H. Ta, H. L. Han, L. N. Tan and V. Vo, BiOCl1−xBrx solid solution – Novel synthesis, highly-efficient visible-light-driven photocatalyst, and DFT study, J. Alloys Compd., 2023, 960, 170503 CrossRef CAS.
- X. Li, T. Chen, H. Lin, J. Cao, H. Huang and S. Chen, Intensive photocatalytic activity enhancement of Bi5O7I via coupling with band structure and content adjustable BiOBrxI1−x, Sci. Bull., 2018, 63(4), 219–227 CrossRef CAS.
- X. Qiu, S. Lin, J. Li and L. Guo, One-Step Coprecipitation Synthesis of BiOClxBr1-x Photocatalysts Decorated with CQDs at Room Temperature with Enhanced Visible-Light Response, Inorg. Chem., 2022, 61(28), 10999–11010 CrossRef CAS.
- C. Hua, X. Dong, Y. Wang, N. Zheng, H. Ma and X. Zhang, Synthesis of a BiOCl1-xBrx@AgBr heterostructure with enhanced photocatalytic activity under visible light, RSC Adv., 2018, 8(30), 16513–16520 RSC.
- B. Zhang, S. Fu, D. Wang, S. Jiao, Z. Zeng, X. Zhang, Z. Xu, Y. Liu, C. Zhao, J. Pan, D. Liu and J. Wang, Synthesis and enhanced light photocatalytic activity of modulating band BiOBrxI1−x nanosheets, Nanomaterials, 2021, 11(11), 2940 CrossRef CAS.
- J. Wan, W. Yang, J. Liu, K. Sun, L. Liu and F. Fu, Enhancing an internal electric field by a solid solution strategy for steering bulk-charge flow and boosting photocatalytic activity of Bi24O31ClxBr10–x, Chin. J. Catal., 2022, 43(2), 485–496 CrossRef CAS.
- X. Zhang, L. W. Wang, C. Y. Wang, W. K. Wang, Y. L. Chen, Y. X. Huang, W. W. Li, Y. J. Feng and H. Q. Yu, Synthesis of BiOClxBr1-x Nanoplate Solid Solutions as a Robust Photocatalyst with Tunable Band Structure, Chem. – Eur. J., 2015, 21(33), 11872–11877 CrossRef CAS.
- Y. L. Qi, Y. F. Zheng and X. C. Song, Au-BiOCl1−xBrx composites with highly enhanced photocatalytic activity for phenol decomposition, J. Alloys Compd., 2017, 726, 1147–1154 CrossRef CAS.
- J. Han, G. Zhu, M. Hojamberdiev, J. Peng and P. Liu, Temperature effect on phase transition and morphological transformation of BiOI microspheres to Bi5O7I microstructures, Mater. Lett., 2016, 169, 122–125 CrossRef CAS.
- L. Zhang, J. Yang, X. Zhao, X. Xiao, F. Sun, X. Zuo and J. Nan, Small-molecule surface-modified bismuth-based semiconductors as a new class of visible-light-driven photocatalytic materials: Structure-dependent photocatalytic properties and photosensitization mechanism, Chem. Eng. J., 2020, 380, 122546 CrossRef CAS.
- L. Chen, S. F. Yin, R. Huang, Y. Zhou, S. L. Luo and C. T. Au, Facile synthesis of BiOCl nano-flowers of narrow band gap and their visible-light-induced photocatalytic property, Catal. Commun., 2012, 23, 54–57 CrossRef CAS.
- J. Zhang, Q. Han, J. Zhu and X. Wang, A facile and rapid room-temperature route to hierarchical bismuth oxyhalide solid solutions with composition-dependent photocatalytic activity, J. Colloid Interface Sci., 2016, 477, 25–33 CrossRef CAS.
- M. Yadav, S. Garg, A. Chandra and K. Hernadi, Fabrication of leaf extract mediated bismuth oxybromide/oxyiodide (BiOBrxI1−x) photocatalysts with tunable band gap and enhanced optical absorption for degradation of organic pollutants, J. Colloid Interface Sci., 2019, 555, 304–314 CrossRef CAS.
- Y. L. Qi, Y. F. Zheng and X. C. Song, Synthetic adjustable energy band structure of BiPO4-BiOClxBr1−x p–n heterojunctions with excellent photocatalytic activity, J. Taiwan Inst. Chem. Eng., 2017, 77, 216–226 CrossRef CAS.
- L. Qi, Y. Yang, P. Zhang, Y. Le, C. Wang and T. Wu, Hierarchical flower-like BiOIxBr(1−x) solid solution spheres with enhanced visible-light photocatalytic activity, Appl. Surf. Sci., 2019, 467–468, 792–801 CrossRef CAS.
- X. Sun, Y. Zhang, C. Li, Z. Zhang, Z. Peng, H. Si and L. Y. Zhang, BiOClxBryIz (x + y + z = 1) solid solutions with controllable band gap and highly enhanced visible light photocatalytic performances, J. Alloys Compd., 2015, 638, 254–260 CrossRef CAS.
- Y. Zhang, X. Sun, G. Yang, Y. Zhu, H. Si, J. Zhang and Y. Li, Preparation and characterization of bifunctional BiOClxIy solid solutions with excellent adsorption and photocatalytic abilities for removal of organic dyes, Mater. Sci. Semicond. Process., 2015, 41, 193–199 CrossRef.
- H. Wang, B. Zhang, F. Zhao and B. Zeng, One-Pot Synthesis of N-Graphene Quantum Dot-Functionalized I-BiOCl Z-Scheme Cathodic Materials for “signal-Off” Photoelectrochemical Sensing of Chlorpyrifos, ACS Appl. Mater. Interfaces, 2018, 10(41), 35281–35288 CrossRef CAS.
- A. M. Alansi, T. F. Qahtan, N. Al Abass, M. Al-Qunaibit and T. A. Saleh, In-situ sunlight-driven tuning of photo-induced electron-hole generation and separation rates in bismuth oxychlorobromide for highly efficient water decontamination under visible light irradiation, J. Colloid Interface Sci., 2022, 614, 58–65 CrossRef CAS.
- Q. Zhang, W. Nie, T. Hou, H. Shen, Q. Li, C. Guan, L. Duan and X. Zhao, Optical and Photocatalytic Properties of Br-Doped BiOCl Nanosheets with Rich Oxygen Vacancies and Dominating {001} Facets, Nanomaterials, 2022, 12(14), 2423 CrossRef CAS.
- G. Zhang, L. Cai, Y. Zhang and Y. Wei, Bi5+, Bi(3−x)+, and Oxygen Vacancy Induced BiOClxI1−x Solid Solution toward Promoting Visible-Light Driven Photocatalytic Activity, Chem. – Eur. J., 2018, 24(29), 7434–7444 CrossRef CAS.
- M. Ji, Y. Shao, E. Nkudede, Z. Liu, X. Sun, J. Zhao, Z. Chen, S. Yin, H. Li and J. Xia, Oxygen vacancy triggering the broad-spectrum photocatalysis of bismuth oxyhalide solid solution for ciprofloxacin removal, J. Colloid Interface Sci., 2022, 626, 221–230 CrossRef CAS.
- K. Xu, D. Xu, Z. Li, S. Zhang, L. Tong, J. Peng, S. Zhang, J. Shen and X. Chen, Enhanced visible-light photocatalytic degradation of ciprofloxacin hydrochloride by bulk iodine doped BiOCl with rich oxygen vacancy, Appl. Surf. Sci., 2022, 578, 152083 CrossRef CAS.
- H. Wang, B. Zhang, J. Xi, F. Zhao and B. Zeng, Z-scheme I-BiOCl/CdS with abundant oxygen vacancies as highly effective cathodic material for photocathodic immunoassay, Biosens. Bioelectron., 2019, 141(6), 111443 CrossRef CAS.
- J. Guo, X. Li, J. Liang, X. Yuan, L. Jiang, H. Yu, H. Sun, Z. Zhu, S. Ye, N. Tang and J. Zhang, Fabrication and regulation of vacancy-mediated bismuth oxyhalide towards photocatalytic application: Development status and tendency, Coord. Chem. Rev., 2021, 443, 214033 CrossRef.
- C. Wang, H. Qiu, T. Inoue and Q. Yao, Electronic structure calculations of I and Mn doped BiOCl with modified Becke – Johnson potential, Comput. Mater. Sci., 2014, 85, 138–141 CrossRef CAS.
- H. Liu, Y. Su, Z. Chen, Z. Jin and Y. Wang, Novel 3D flowerlike Au/BiOBr0.2I0.8 composites with highly enhanced visible-light photocatalytic performances, Sep. Purif. Technol., 2014, 133, 343–350 CrossRef CAS.
- Z. Han, M. Lv, X. Shi, G. Li, J. Zhao and X. Zhao, Regulating the Electronic Structure of Fe3+-Doped BiOClxI1–x Solid Solution by an Amidoxime-Functionalized Fibrous Support for Efficient Photocatalysis, Adv. Fiber Mater., 2023, 5(1), 266–281 CrossRef CAS.
- S. S. Cui, X. Liu, Y. B. Shi, M. Y. Ding and X. F. Yang, Construction of atomic-level charge transfer channel in Bi12O17Cl2/MXene heterojunctions for improved visible-light photocatalytic performance, Rare Met., 2022, 41(7), 2405–2416 CrossRef CAS.
- C. Zeng, Y. Hu and H. Huang, BiOBr0.75I0.25/BiOIO3 as a Novel Heterojunctional Photocatalyst with Superior Visible-Light-Driven Photocatalytic Activity in Removing Diverse Industrial Pollutants, ACS Sustainable Chem. Eng., 2017, 5(5), 3897–3905 CrossRef CAS.
- D. Wu, L. Zeng, Y. Liu, C. Yuan, X. Xue and X. Zhang, Design of 2D/2D heterojunction of Ti3C2/BiOClxBr1−x for enhancing photocatalytic performance, Colloids Surf., A, 2023, 663, 131010 CrossRef CAS.
- J. Han, C. Lu, Y. Wang, S. Zhang, L. Zhang and Q. Yin, Fabrication of TiO2-BiOBrxI1-x heterojunctions with adjustable band structure for enhanced visible light photocatalytic activity, J. Alloys Compd., 2020, 825, 152047 CrossRef CAS.
- P. Yue, G. Zhang, X. Cao, B. Wang, Y. Zhang and Y. Wei, In situ synthesis of Z-scheme BiPO4/BiOCl0.9I0.1 heterostructure with multiple vacancies and valence for efficient photocatalytic degradation of organic pollutant, Sep. Purif. Technol., 2019, 213, 34–44 CrossRef CAS.
- L. Zhang, Y. Li, Q. Li, J. Fan, S. A. C. Carabineiro and K. Lv, Recent advances on Bismuth-based Photocatalysts: Strategies and mechanisms, Chem. Eng. J., 2021, 419, 129484 CrossRef CAS.
- S. Shi, M. A. Gondal, S. G. Rashid, Q. Qi, A. A. Al-saadi, Z. H. Yamani, Y. Sui, Q. Xu and K. Shen, Synthesis of g-C3N4/BiOClxBr1−x hybrid photocatalysts and the photoactivity enhancement driven by visible light, Colloids Surf., A, 2014, 461, 202–211 CrossRef CAS.
- X. Hu, Y. Zhang, B. Wang, H. Li and W. Dong, Novel g-C3N4/BiOClxI1-x nanosheets with rich oxygen vacancies for enhanced photocatalytic degradation of organic contaminants under visible and simulated solar light, Appl. Catal., B, 2019, 256, 117789 CrossRef CAS.
- X. Sun, Y. Du, Z. Li, S. Chen, Y. Feng, N. Jiang, Y. Liu, J. Wang and H. Li, Facile synthesis of g-C3N4/BiOClxI1-x hybrids with efficient charge separation for visible-light photocatalysis, Ceram. Int., 2020, 46, 10843–10850 CrossRef CAS.
- Y. Feng, Y. Du, M. Du, Z. Li, Z. He, K. Yang, X. Lv, N. Jiang and Y. Liu, Facile constructing novel 3D porous g-C3N4/BiOBr0.2I0.8 hybrids: Efficient charge separation for visible-light photocatalysis, J. Alloys Compd., 2018, 767, 241–252 CrossRef CAS.
- H. Liu, Y. Su, Z. Chen, Z. Jin and Y. Wang, Graphene sheets grafted three-dimensional BiOBr0.2I0.8 microspheres with excellent photocatalytic activity under visible light, J. Hazard. Mater., 2014, 266, 75–83 CrossRef CAS.
- K. Gao, X. Gao, W. Zhu, C. Wang, T. Yan, F. Fu, J. Liu, C. Liang and Q. Li, The hierarchical layered microsphere of BiOIxBr1-x solid solution decorated with N-doped CQDs with enhanced visible light photocatalytic oxidation pollutants, Chem. Eng. J., 2021, 406, 127155 CrossRef CAS.
- J. Han, S. Zhang, C. Lu, L. Zhang, K. Cao, N. Li and Q. Yin, Band engineering design of g-C3N4-BiOClxBr1−x direct Z-scheme heterojunctions with remarkable enhancement of photocatalytic performance, Appl. Surf. Sci., 2021, 546, 148887 CrossRef CAS.
- W. Dong, H. Ren, T. Xie and H. Lin, A novel route to synthesize BiOCl1-xBrx solid solution with excellent photocatalytic performance in visible range by etching bismuth glass, Mater. Lett., 2022, 317, 132043 CrossRef CAS.
- M. Yadav, S. Garg, A. Chandra and K. Hernadi, Fabrication of leaf extract mediated bismuth oxybromide/oxyiodide (BiOBrxI1−x) photocatalysts with tunable band gap and enhanced optical absorption for degradation of organic pollutants, J. Colloid Interface Sci., 2019, 555, 304–314 CrossRef CAS.
- F. Deng, Y. Luo, H. Li, B. Xia, X. Luo, S. Luo and D. D. Dionysiou, Efficient toxicity elimination of aqueous Cr(VI) by positively-charged BiOClxI1-x, BiOBrxI1-x and BiOClxBr1-x solid solution with internal hole-scavenging capacity via the synergy of adsorption and photocatalytic reduction, J. Hazard. Mater., 2020, 383, 121127 CrossRef CAS.
- Y. Bai, L. Ye, T. Chen, P. Wang, L. Wang, X. Shi and P. K. Wong, Synthesis of hierarchical bismuth-rich Bi4O5BrxI2-x solid solutions for enhanced photocatalytic activities of CO2 conversion and Cr(VI) reduction under visible light, Appl. Catal., B, 2017, 203, 633–640 CrossRef CAS.
- H. Ji, C. Hu, S. Zhang, L. Zhang and X. Yang, BiO(OH)xI1-x solid solution with rich oxygen vacancies: interlayer guest hydroxyl for improved photocatalytic properties, J. Colloid Interface Sci., 2022, 605, 1–12 CrossRef CAS.
- A. Chachvalvutikul, T. Luangwanta, B. Inceesungvorn and S. Kaowphong, Bismuth-rich oxyhalide (Bi7O9I3–Bi4O5Br2) solid-solution photocatalysts for the degradation of phenolic compounds under visible light, J. Colloid Interface Sci., 2023, 641, 595–609 CrossRef CAS.
- X. Wang, Z. Zhang, Y. Xue, M. Nie, H. Li and W. Dong, Low crystallized BiOCl0.75I0.25 synthesized in mixed solvent and its photocatalytic properties under simulated solar irradiation, Mater. Lett., 2014, 136(3), 30–33 CrossRef CAS.
- C. S. L. Fung, M. Khan, A. Kumar and I. M. C. Lo, Visible-light-driven photocatalytic removal of PPCPs using magnetically separable bismuth oxybromo-iodide solid solutions: Mechanisms, pathways, and reusability in real sewage, Sep. Purif. Technol., 2019, 216, 102–114 CrossRef CAS.
- R. Lu, A. H. Zahid and Q. Han, Insight into the photocatalytic mechanism of the optimal x value in the BiOBrxI1−x, BiOClxI1−x and BiOClxBr1−x series varying with pollutant type, Nanoscale, 2022, 14(37), 13711–13721 RSC.
- X. Li, J. Cai, M. Li, L. Zhang and Z. Chen, Growth of BiOBrxI1-x solid-solutions with tunable band-gaps on carbon fiber cloth as flexible membrane-shaped photocatalyst for purifying antibiotic wastewater, J. Cleaner Prod., 2023, 414, 137725 CrossRef CAS.
- T. Kaewmanee, S. Wannapop, A. Phuruangrat, T. Thongtem and S. Thongtem, Solvothermal synthesis of BiOBrxI1-x (x = 0.0–1.0) solid solutions used for adsorption and photodegradation of cationic and anionic dyes, Inorg. Chem. Commun., 2021, 134, 109054 CrossRef CAS.
- H. Y. Xu, D. Lu, Q. Tan, X. L. He and S. Y. Qi, Visible-light-driven photocatalytic degradation of rhodamine B in water by BiOClxI1-x solid solutions, Water Sci. Technol., 2020, 81(5), 1080–1089 CrossRef CAS.
- L. Kong, J. Guo, J. W. Makepeace, T. Xiao, H. F. Greer, W. Zhou, Z. Jiang and P. P. Edwards, Rapid synthesis of BiOBrxI1-x photocatalysts: Insights to the visible-light photocatalytic activity and strong deviation from Vegard's law, Catal. Today, 2019, 335, 477–484 CrossRef CAS.
- S. Liu, P. Liang, J. Liu, J. Xin, X. Li, C. Shao, X. Li and Y. Liu, Anchoring bismuth oxybromo-iodide solid solutions on flexible electrospun polyacrylonitrile nanofiber mats for floating photocatalysis, J. Colloid Interface Sci., 2022, 608, 3178–3191 CrossRef CAS.
- J. Lu, Q. Meng, H. Lv, L. Shui, M. Jin, Z. Zhang, Z. Chen, M. Yuan, X. Wang, J. M. Liu and G. Zhou, Synthesis of visible-light-driven BiOBrxI1-x solid solution nanoplates by ultrasound-assisted hydrolysis method with tunable bandgap and superior photocatalytic activity, J. Alloys Compd., 2018, 732, 167–177 CrossRef CAS.
- K. Ren, J. Liu, J. Liang, K. Zhang, X. Zheng, H. Luo, Y. Huang, P. Liu and X. Yu, Synthesis of the bismuth oxyhalide solid solutions with tunable band gap and photocatalytic activities, Dalton Trans., 2013, 42(26), 9706–9712 RSC.
- D. Du, W. Li, S. Chen, T. Yan, J. You and D. Kong, Synergistic degradation of rhodamine B on BiOClxBr1-x sheets by combined photosensitization and photocatalysis under visible light irradiation, New J. Chem., 2015, 39(4), 3129–3136 RSC.
- H. Gnayem, A. Dandapat and Y. Sasson, Development of Hybrid BiOClxBr1-x-Embedded Alumina Films and Their Application as Highly Efficient Visible-Light-Driven Photocatalytic Reactors, Chem. – Eur. J., 2016, 22(1), 370–375 CrossRef CAS.
- Y. y. Gu, Y. q. Xiong, X. x. Zhang, L. Zhao, S. c. Zhang and J. Yan, Facile synthesis of hierarchical BiOClxBr1–x solid solution with enhanced photocatalytic activity, J. Cent. South Univ., 2018, 25(7), 1619–1627 CrossRef CAS.
- X. Zhang, C. Y. Wang, L. W. Wang, G. X. Huang, W. K. Wang and H. Q. Yu, Fabrication of BiOBrxI1-x photocatalysts with tunable visible light catalytic activity by modulating band structures, Sci. Rep., 2016, 6, 22800 CrossRef CAS.
- S. Shenawi-Khalil, V. Uvarov, Y. Kritsman, E. Menes, I. Popov and Y. Sasson, A new family of BiO(ClxBr1-x) visible light sensitive photocatalysts, Catal. Commun., 2011, 12(12), 1136–1141 CrossRef CAS.
- G. Zhang, L. Zhang, Y. Liu, L. Liu, C. P. Huang, H. Liu and J. Li, Substitution Boosts Charge Separation for High Solar-Driven Photocatalytic Performance, ACS Appl. Mater. Interfaces, 2016, 8(40), 26783–26793 CrossRef CAS.
- N. Mohseni, M. Haghighi and M. Shabani, Sunlight-activated 3D-mesoporous-flowerlike Cl–Br bismuth oxides nanosheet solid solution: In situ EG-thermal-sonication synthesis with excellent photodecomposition of ciprofloxacin, Environ. Res., 2020, 188, 109810 CrossRef CAS.
- Y. Li, J. Lai, X. Zheng, S. Lv, J. Yang and S. Cui, Fabrication of wool ball-like F-doped BiOCl0.4Br0.3I0.3 composite for effective sulfamethazine photocatalytic degradation, Mater. Res. Bull., 2020, 130, 110937 CrossRef CAS.
- S. Gholizadeh and M. Haghighi, Environmental Facile one-pot ultrasound-assisted solvothermal fabrication of ball- flowerlike nanostructured (BiOBr)x(Bi7O9I3)1-x solid-solution for high active photodegradation of antibiotic levofloxacin under sun-light, Appl. Catal., B, 2019, 248, 320–331 CrossRef.
- R. Ameta, S. Benjamin, A. Ameta and S. C. Ameta, Photocatalytic degradation of organic pollutants: A review, Mater. Sci. Forum, 2013, 734, 247–272 Search PubMed.
- X. Gao, K. Gao, W. Zhu, C. Liang, Q. Li, F. Fu and Y. Zhu, Accurate guided alternating atomic layer enhance internal electric field to steering photogenerated charge separation for enhance photocatalytic activity, Appl. Catal., B, 2021, 298, 120536 CrossRef CAS.
- Q. Y. Liu, G. Han, Y. F. Zheng and X. C. Song, Synthesis of BiOBrxI1−x solid solutions with dominant exposed {0 0 1} and {1 1 0} facets and their visible-light-induced photocatalytic properties, Sep. Purif. Technol., 2018, 203, 75–83 CrossRef CAS.
- H. He, C. Liu, M. Li, Y. Liu and R. Zhu, Synergistic photocatalytic degradation mechanism of BiOClxI1-x-OVs based on oxygen vacancies and internal electric field-mediated
solid solution, Chemosphere, 2023, 337, 139281 CrossRef CAS.
- X. Zhang, P. Yang, B. Yang, Y. Bai, W. Liu and K. Zhang, Synthesis of the Ag-AgCl0.5Br0.5/BiOCl0.5Br0.5 composite for enhanced photocatalytic activity of degradation of oil field pollutant through synergistic effect of ag-bi based solid solution, J. Photochem. Photobiol., A, 2019, 384, 112047 CrossRef CAS.
- X. Zhang, P. Yang, Y. Bai, B. Yang and W. Liu, Synthesis of efficient composite photocatalysts from solid solution Bi3O4Cl0.5Br0.5 and Ag-AgI/AgCl for decomposition the oil field pollutants of phenol and acrylamide, Adv. Powder Technol., 2020, 31(3), 973–985 CrossRef CAS.
- Q. Qin, Y. Guo, D. Zhou, Y. Yang and Y. Guo, Facile growth and composition-dependent photocatalytic activity of flowerlike BiOCl1−xBrx hierarchical microspheres, Appl. Surf. Sci., 2016, 390, 765–777 CrossRef CAS.
- H. Zhang, J. C. L. Tee, S. Jaenicke, M. A. Gondal, M. A. Dastageer, C. Basheer and G. K. Chuah, BiOBrnI1-n solid solutions as versatile photooxidation catalysts for phenolics and endocrine disrupting chemicals, Catal. Today, 2021, 375, 547–557 CrossRef CAS.
- M. B. Hussain, M. S. Khan, H. M. Loussala and M. S. Bashir, The synthesis of a BiOClxBr1-x nanostructure photocatalyst with high surface area for the enhanced visible-light photocatalytic reduction of Cr(VI), RSC Adv., 2020, 10(8), 4763–4771 RSC.
- J. Wang, H. Mei, D. Jin, Q. Lin, R. Zhang and X. Wang, Indirect Substitution Constructing Halogen-Vacancy BiOCl1–xIn Solid Solution with a Suitable Surface Structure for Enhanced Photoredox Performance, Inorg. Chem., 2022, 61, 8540–8549 CrossRef CAS.
- X. Wu, K. Zhang, G. Zhang and S. Yin, Facile preparation of BiOX (X = Cl, Br, I) nanoparticles and up-conversion phosphors/BiOBr composites for efficient degradation of NO gas: Oxygen vacancy effect and near infrared light responsive mechanism, Chem. Eng. J., 2017, 325, 59–70 CrossRef CAS.
- F. Dong, Y. Sun, M. Fu, Z. Wu and S. C. Lee, Room temperature synthesis and highly enhanced visible light photocatalytic activity of porous BiOI/BiOCl composites nanoplates microflowers, J. Hazard. Mater., 2012, 219–220, 26–34 CrossRef CAS.
- T. Wu, X. Li, D. Zhang, F. Dong and S. Chen, Efficient visible light photocatalytic oxidation of NO with hierarchical nanostructured 3D flower-like BiOClxBr1-x solid solutions, J. Alloys Compd., 2016, 671, 318–327 CrossRef CAS.
- Y. Bai, P. Yang, P. Wang, Z. Fan, H. Xie, P. K. Wong and L. Ye, Solid phase fabrication of Bismuth-rich Bi3O4ClxBr1−x solid solution for enhanced photocatalytic NO removal under visible light, J. Taiwan Inst. Chem. Eng., 2018, 82, 273–280 CrossRef CAS.
- M. Ou, F. Dong, W. Zhang and Z. Wu, Efficient visible light photocatalytic oxidation of NO in air with band-gap tailored (BiO)2CO3-BiOI solid solutions, Chem. Eng. J., 2014, 255, 650–658 CrossRef CAS.
- M. Gao, J. Yang, T. Sun, Z. Zhang, D. Zhang, H. Huang, H. Lin, Y. Fang and X. Wang, Persian buttercup-like BiOBrxCl1-x solid solution for photocatalytic overall CO2 reduction to CO and O2, Appl. Catal., B, 2019, 243, 734–740 CrossRef CAS.
- D. Pancholi, N. S. Bisht, V. Pande and A. Dandapat, Development of novel BiOBr0.75I0.25 nanostructures with remarkably High dark phase bactericidal activities, Colloids Surf., B, 2021, 199, 111558 CrossRef CAS.
- A. Dandapat, I. Horovitz, H. Gnayem, Y. Sasson, D. Avisar, T. Luxbacher and H. Mamane, Solar Photocatalytic Degradation of Trace Organic Pollutants in Water by Bi(0)-Doped Bismuth Oxyhalide Thin Films, ACS Omega, 2018, 3(9), 10858–10865 CrossRef CAS.
- S. Ge, D. Li, Y. Gao, J. Hou, J. Fu, W. Li, P. Li, C. Zhu, S. Guo and Z. Zheng, Dual control of surface oxygen vacancies and exposed facets onto BiOCl0.95Br0.05 sheets for enhancing photocatalytic degradation of sodium pentachlorophenate, J. Alloys Compd., 2021, 871, 159568 CrossRef CAS.
- H. Gnayem and Y. Sasson, Nanostructured 3D Sunflower-like Bismuth Doped BiOClxBr1-x Solid Solutions with Enhanced Visible Light Photocatalytic Activity as a Remarkably Efficient Technology for Water Purification, J. Phys. Chem. C, 2015, 119(33), 19201–19209 CrossRef CAS.
|
This journal is © The Royal Society of Chemistry 2024 |
Click here to see how this site uses Cookies. View our privacy policy here.