DOI:
10.1039/D4DT00694A
(Paper)
Dalton Trans., 2024,
53, 9763-9776
Radical and diradical states of bis(molybdenocene dithiolene) complexes†
Received
7th March 2024
, Accepted 16th May 2024
First published on 21st May 2024
Abstract
The synthesis and characterization of two bis(dithiolene) proligands involving heteroatomic linkers such as 1,4-dithiine and dihydro-1,4-disiline between the two protected dithiolene moieties are described. Two bimetallic complexes involving these heteroatomic bridges between two redox active bis(cyclopentadienyl)molybdenum dithiolene moieties have been synthesized and characterized by electrochemistry, spectroelectrochemistry, and their properties rationalized with (TD-)DFT. Cyclic voltammetry experiments show sequential oxidation of the two redox centers with ΔE values between successive one-electron transfers varying according to the nature of the bridge. Depending on the nature of the heteroatomic bridge, the bis-oxidized complexes exhibit either a diradical character with both radicals essentially localized on the metallacycles, or a closed-shell dicationic state.
Introduction
Dithiolene ligands are non-innocent bidentate ligands which have been known for more than sixty years1 for their use as precursors of various conducting metal bis(dithiolene) complexes of interest for materials science.2 The synthesis of ditopic dithiolene ligands, i.e. two covalently linked dithiolene ligands, is also currently of interest for the elaboration of conducting polymeric materials. For this purpose, the two chelating sites can be connected through a conjugated organic bridge of various lengths as in tetrathiooxalate (tto),3–5 1,2,4,5-benzenetetrathiolate (btt),6–8 or tetrathiafulvalentetrathiolate (TTFS4) (Chart 1a).9,10 It is worth mentioning that these three ditopic dithiolene ligands present a common parameter, the two dithiolene units are fused to the organic spacer leading to an almost planar overall structure. This conjugated organic bridge might allow for electronic communication between the two coordinated metal ions, an important parameter to enable charge transport within polymeric materials.11 In order to evidence the electronic communication between two coordinated metal ions, various electroactive bimetallic complexes have been investigated.12 For instance, with the btt ligand, electronic interplay has been evidenced in several bimetallic complexes by electrochemistry through the occurrence of multi redox systems and by spectroelectrochemistry through the appearance of an intervalence charge transfer (IVCT) band in the near-IR region.13–17 For example, when the btt ligand is bridging two bis(cyclopentadienyl) molybdenum(IV) moieties, Mo2B in Chart 1b, a sequential oxidation of each electrophore, Cp2Mo(dithiolene) units, was observed together with the appearance of an IVCT band in the near IR for the mono-oxidized state.17 Among the ditopic dithiolene ligands used as precursor of bis molybdenocene complexes, the btt ligand led to the strongest interactions between the two electrophores.17,18 Herein, in order to keep the two metallacycles in close vicinity, we focused on the use of other six membered heterocycles that could bring different degree of delocalization relative to the btt situation. Thus, we decided to investigate the corresponding 1,4-dithiine and dihydro-1,4-disiline bis(dithiolene) derivatives, S2tt and Si2tt respectively, Chart 1b. These ligands have never been prepared even though theoretical calculations have been performed on coordination polymers involving the S2tt ligand.19 Heteroatom linkers, such as dithiine or dihydro-1,4-disiline, have been previously used as bridges between electroactive organic molecules such as tetrathiafulvalene (TTF) and they both have shown their efficiency to allow for electronic interplay within the two connected TTFs.20,21 Therefore we decided to prepare the corresponding ditopic ligands, S2tt and Si2tt (Chart 1b) and to study their propensity to allow for electronic communication within bimetallic complexes, Mo2S2 and Mo2Si2 (Chart 1c). Indeed, Cp2Mo(dithiolene) complexes are redox active moieties and the heterocyclic linker between the two metallacycles could modulate the nature and the strength of electronic interactions. In this paper we will present a straightforward synthesis of the two novel proligands and the corresponding bimetallic complexes involving two Cp2Mo(dithiolene) units. Electrochemical and spectrochemical studies have been carried out on the two bimetallic complexes and highlight different behaviors, depending on the nature of the heterocycle. DFT calculations shed light on the comprehension of the possible electronic interactions between the two electrophores depending on the bridge nature.
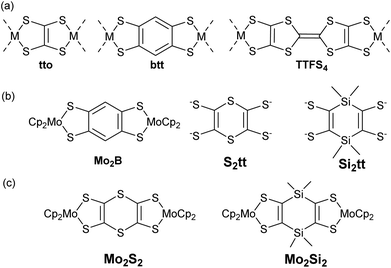 |
| Chart 1 (a) Tetrathiolate ligands involved in linear coordination polymers. (b) Mo2B, 1,4-dithiinetetrathiolate (S2tt) and dihydro-1,4-disilinetetrathiolate (Si2tt) ligands. (c) Target bis molydenocene complexes Mo2S2 and Mo2Si2. | |
Results and discussion
Synthesis of the proligands
During the course of our investigations on Cp2Mo(dithiolene) complexes we found that dithiol-2-one22 was an excellent dithiolene proligand for the syntheses of such complexes. Therefore, we focused our attention on the preparation of bis(dithiol-2-one) derivatives where the two heterocycles are connected either with a dithiine or a dihydro-1,4-disiline bridging linker. For the dithiine proligand 2, we did not follow the literature procedure as the overall yield starting from [Bu4N][Zn(dmit)2] led to the corresponding dithiine in 2.6% yield only.23 Instead we developed a novel approach based on the copper catalyzed coupling between aryl iodides and zinc–thiolate complex.24 Thus, by coupling the 4,5-diiodo-1,3-dithiole-2-thione25 and the [Bu4N][Zn(dmit)2] in the presence of Cu2O we isolated the sulfur-bridged bis(dithiole-2-thione) 1 in 89% yield (Scheme 1a). This compound is poorly soluble in common organic solvents. Nevertheless, the transchalcogenation of 1 into the bis(dithiol-2-one) 2 was successfully realized by using mercuric acetate in chloroform solution. According to this approach, we isolated the desired proligand 2 in 52% overall yield starting from [Bu4N][Zn(dmit)2].
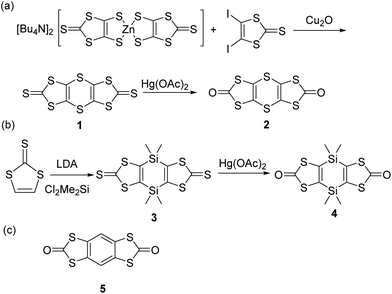 |
| Scheme 1 Synthesis of the proligands 2 and 4, and molecular structure of 5. | |
Concerning the synthesis of the dihydro-1,4-disiline bridged bis(dithiolene) proligand 4 we used a similar strategy than the one described in the literature on the tetrathiafulvalene that we adapted for the 1,3-dithiole-2-thione as outlined in Scheme 1b.21 The bis(metalation) of the 1,3-dithiole-2-thione was realized by adding an excess of LDA and the dilithiated species is further reacted with dichlorodimethylsilane, Me2SiCl2. The bis(dithiole-2-thione) 3 was converted into the corresponding dihydro-1,4-disiline bridged bis(dithiole-2-one) 4 by using the procedure described above for the dithiine analogue. It is worthmentioning that the proligand 4 is highly soluble in common organic solvents while the dithiine proligand 2 is less soluble.
Crystals were obtained by recrystallization of 2 in CHCl3 and by slow evaporation of a concentrated dichloromethane solution of the derivatives 3 and 4. For comparison we also recrystallized the known benzo fused bis(dithiol-2-one) 526 into chlorobenzene (Scheme 1c). The molecular structures of the three proligands 2, 4 and 5 obtained from single X-ray diffraction are given in Fig. 1, the structure of precursor 3 is given in Fig. S1† and bond lengths and selected angles are collected in Table S1 in ESI.† As can be seen in Fig. 1, the dithiine proligand 2 is not planar with a strong folding angle along the S⋯S hinge of 130°. This value is in the range observed for dithiine subunits which are known to adopt a non-planar conformation (S⋯S hinge of 127 to 137°)27,28 and to exhibit clamp-like hinge motions by folding along the S⋯S axis with an inversion barrier depending on the fused cycles on either side of the 1,4-dithiine cycle from 3 to 7 kcal mol−1.27–29 Contrariwise, the dihydro-1,4-disiline proligand 4 and the benzo-fused proligand 5 are fully planar.
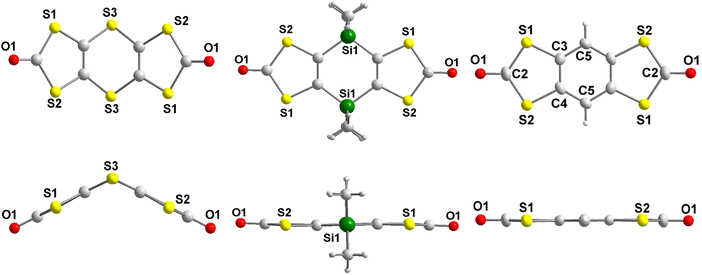 |
| Fig. 1 Top and side views of molecular structures of proligand 2 (left), 4 (middle) and 5 (right). | |
Syntheses of the complexes
Having in hands these two new proligands 2 and 4, where two dithiol-2-one are fused to a six-membered ring, we attempted the formation of the bimetallic complexes through the successive addition of MeONa and bis(cyclopentadienyl) molybdenum dichloride (Cp2MoCl2) in the medium (Scheme 2). All these reactions were performed using Schlenck techniques as the expected complexes are presumably easy to oxidize, as observed for the benzo analogue Mo2B.18b In all the cases, the colourless medium of the proligands turned darkly coloured after the addition of base followed by the one of Cp2MoCl2. With the dithiine fused bis(dithiolene), we isolated the bis-substituted complex, Mo2S2, a poorly soluble complex in common organic solvents, together with more soluble byproducts which were identified by X-ray diffraction studies as molybdenocene disulfide Cp2MoS2
30 and Cp2MoS2CO (Fig. S2 and Table S2 in ESI†). On the other hand, in the case of the dihydro-1,4-disiline proligand 4, the addition of the two reagents led to the destruction of the heterocyclic ring presumably due to the nucleophilic attack of methanolate on the silicium atoms leading to an opening of the ring. Nevertheless, this approach allowed us to isolate the simplest molybdenocene(dithiolene) complex, Modt. This complex has been previously reported but with a different synthetic approach and neither crystal structure or electrochemical properties have been investigated so far for this complex.31 To reach the desired bimetallic dihydro-1,4-disiline complex, Mo2Si2, instead of using MeONa we favoured the use of nBuLi to deprotect the dithiolene ligands. Accordingly, Mo2Si2 was isolated as a soluble bimetallic complex in 47% yield, and the monometallic complex MoSi2 was isolated as a side product in 6% yield (Scheme 2).
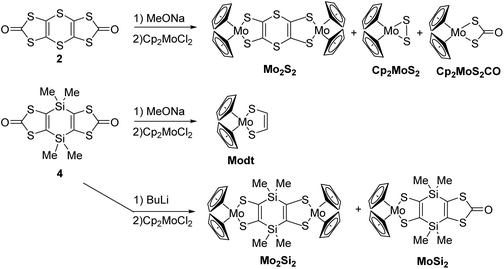 |
| Scheme 2 Synthesis of the metallocene complexes Mo2S2, Modt, MoSi2 and Mo2Si2. | |
Crystals of the mononuclear complexes, Modt and MoSi2, were obtained by slow concentration of a dichloromethane solution and the molecular structures, elucidated by X-ray diffraction, are given in Fig. 2 and significant bond lengths and angles are collected in Table 1. These complexes crystallize in the monoclinic system, space group P21/n for Modt and P21/c for MoSi2. The molecular structure of Modt is characterized by a quasi-planar metallacycle (3° along the S⋯S hinge). Similarly, MoSi2 exhibits a quasi-planar structure with the S⋯S hinge of the metallacycle reaching 6.3° while within the dithiol-2-one ring the S⋯S folding angle amounts to 5°.
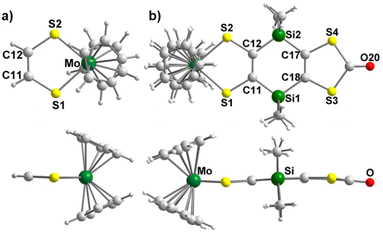 |
| Fig. 2 Molecular structures of Modt (a), MoSi2 (b). | |
Table 1 Significant bond lengths (Å) and angles (°) of Modt and MoSi2

|
Modt
|
MoSi2
|
C C |
1.327(4) |
1.345(5) |
C–S |
1.749(2) |
1.773(3) |
1.753(2) |
1.768(3) |
S–Mo |
2.4513(6) |
2.4345(10) |
2.4483(5) |
2.4360(8) |
S–Mo–S |
82.4(1) |
82.12(3) |
With now the two desired bimetallic complexes, Mo2S2 and Mo2Si2 at hand, together with monometallic reference complexes such as Modt and MoSi2, we are able to investigate their electronic and optical properties, by comparison with our preliminary results reported with the benzene-centered bimetallic and monometallic complexes, Mo2B and MoB respectively.
Electrochemical studies
Cyclic voltammetry measurements were performed on Modt, MoSi2, Mo2Si2 and Mo2S2 complexes in order to determine their redox properties. The mononuclear complexes, Modt and MoSi2, as well as the bimetallic one Mo2Si2 were investigated in CH2Cl2 and in DMSO for comparison purposes. Concerning Mo2S2, it should be mentioned that even in DMSO, this complex is poorly soluble. All the solutions contain 0.1 M of [Bu4N][PF6] as supporting electrolyte. The redox potentials are collected in Table 2 together with those previously determined for the benzo analogues MoB and Mo2B,17 in order to analyze the influence of the heteroatomic bridge on the redox properties.
Table 2 Redox potentialsa and ΔEp
b of the various investigated complexes, E in V vs. SCE using-[Bu4N][PF6]
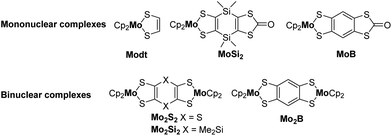
|
Complexes |
|
E
1/2
1 (ΔEp) |
E
1/2
2 (ΔEp) |
E
1/2
3 (ΔEp) |
E
1/2
4 (ΔEp) |
Oxidation processes, taken as the average of the anodic and the cathodic peak potentials.
ΔEp (in mV): peak-to-peak separation.
Irreversible process.
Using CH2Cl2–[Bu4N][B(C6H4(CF3)2)4].
|
Mononuclear (CH2Cl2) |
Modt
|
0.09 (70) |
0.92 (110) |
|
|
MoSi2
|
0.18 (70) |
0.97 (80) |
|
|
MoB
|
0.42 (70) |
1.08 (60) |
|
|
(DMSO) |
Modt
|
0.13 (70) |
0.75* |
|
|
MoSi2
|
0.24 (70) |
0.79* |
|
|
MoB
|
0.43 (60) |
1.01c |
|
|
Binuclear (CH2Cl2) |
Mo2Si2
|
−0.02 (50) |
0.21 (60) |
0.91 |
1.08/0.95 |
Mo2B
|
−0.13 (60) |
0.37 (60) |
1.09 (130) |
|
Mo2Si2
|
−0.01 (60) |
0.28 (70) |
1.38 (90) |
— |
(DMSO) |
Mo2Si2
|
0.06(60) |
0.23 (60) |
0.79* |
|
Mo2S2
|
−0.25 (60) |
0.13 (60) |
|
|
Mo2B
|
−0.03 (60) |
0.38 (70) |
|
|
(CH3CN) |
Mo2S22+
|
−0.26 (60) |
0.11 (60) |
1.00 (60) |
|
Mo2B2+
|
−0.09(60) |
0.34 (60) |
1.05 (60) |
+1.57 |
The cyclic voltammogram (CV) of the mononuclear complexes, Modt and MoSi2, investigated in CH2Cl2, exhibits two reversible oxidation waves corresponding to the reversible oxidation of the neutral complex into the cation radical species and then to the dication one (Fig. 3). A common feature observed on the CV of the mononuclear complexes studied in DMSO, is that the second oxidation process is not reversible anymore and the oxidation potentials are slightly shifted to higher potentials for the first redox process and to lower oxidation potentials for the second irreversible process. These modifications of the shape of the CV, depending on the solvent used, DMSO vs. CH2Cl2, are reminiscent to what was previously observed for other mononuclear such as MoB. There is a significant influence of the substituent on the metallacycle on the overall donating properties. Indeed, the easiest complex to oxidize is the non-substituted one, Modt (+0.09 V vs. SCE in CH2Cl2), while the most difficult to oxidize is the MoB complex with a fused benzo/dithiol-2-one core (+0.42 V vs. SCE in CH2Cl2) demonstrating the electron withdrawing effect of the fused dithiole-2-one ring in MoB.
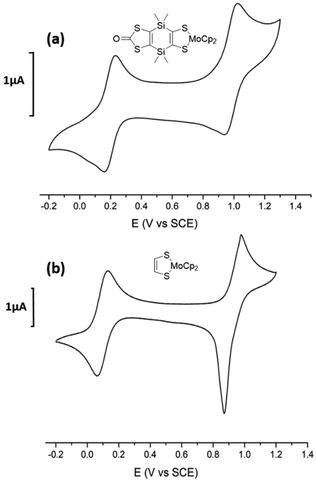 |
| Fig. 3 Cyclic voltammograms of (a) MoSi2 and (b) Modt, in CH2Cl2, [Bu4N][PF6] 0.1 M (100 mV s−1), E in V vs. SCE. | |
The electrochemical investigation carried out on the bimetallic complex Mo2S2 was only performed in DMSO due to solubility issues in CH2Cl2. The CV, reported in Fig. 4a, exhibits only two reversible oxidation processes as observed for the bimetallic complex with a benzo linker, Mo2B, analyzed in the same conditions. Interestingly the presence of the dithiine linker induces a strong cathodic shift of the first redox potential as it occurs at −0.25 V for Mo2S2 compared to −0.03 V for Mo2B (E in V vs. SCE in DMSO)17 as well as on the second redox potential (0.13 V for Mo2S2 and 0.38 V vs. SCE for Mo2B). It should be noted that due to the narrow potential window of DMSO, no redox processes associated with the dithiin oxidation moiety were observed.28a As observed for Mo2B, and taking to account what was previously observed in the dimeric TTF series, the presence of only two redox processes could be due the small potential window of DMSO. The potential difference between these two redox processes amounts to 380 mV, close to the value noticed for Mo2B (410 mV).
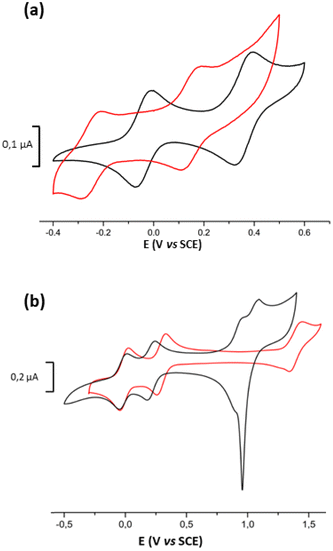 |
| Fig. 4 Cyclic voltammograms of (a) Mo2S2 (red line) and Mo2B (black line) in DMSO, [Bu4N][PF6] 0.1 M, (b) Mo2Si2 in CH2Cl2 [Bu4N][PF6] 0.1 M (black line) and in CH2Cl2 [Bu4N][B(C6H4(CF3)2)4] 0.02 M (red line), E in V vs. SCE, 100 mV s−1. | |
Concerning the bimetallic complex with the dihydro-1,4-disiline linker, Mo2Si2, in dichloromethane, the CV of the Mo2Si2 complex displays four oxidation waves (Fig. 4b). The first two waves are reversible and correspond to the oxidation from the neutral state to the radical cation Mo2Si2˙+ and to the dication Mo2Si22+. The two other oxidation processes at higher potentials are no more reversible probably due to adsorption of the oxidized species at the working electrode. These two oxidation waves are assigned to the successive oxidation of Mo2Si22+ into the tricationic state Mo2Si23+ and then to the tetra one Mo2Si24+. The first redox potential at −0.02 V vs. SCE is significantly shifted, by 110 mV, towards higher oxidation potentials compared to the one of Mo2B in the same conditions (−0.13 V vs. SCE). Another difference with Mo2B, is the potential difference between the two first redox processes (ΔE1/2 = E1/22 − E1/21), which amounts to 230 mV for Mo2Si2 while it amounts to 500 mV for Mo2B in CH2Cl2. Based on the comparison of CVs obtained in CH2Cl2 between Mo2Si2 and Mo2B, the nature of the bridging spacer connecting the two Cp2Mo(dithiolene) moieties influences significantly the redox activity and the interactions between these two electrophores. Based on the splitting of the two first systems (ΔE), the strength of the interactions between the two electrophores is decreasing from the benzo (500 mV in CH2Cl2) to the dihydro-1,4-disiline bridge (230 mV in CH2Cl2).
In order to compare the three bimetallic complexes, Mo2B, Mo2S2, and Mo2Si2, we also studied the redox behavior of Mo2Si2 in DMSO. At variance with the CV obtained in CH2Cl2, the CV of Mo2Si2 realized in DMSO shows clearly the two first redox processes at 0.06 and 0.23 V vs. SCE (ΔE = 170 mV) but the third oxidation process can only be distinguished at 0.79 V, at the beginning of the oxidation of the DMSO itself (Fig. S3 in ESI†). Thus, based on the ΔE between the two first redox potentials which amounts to 410 mV for Mo2B, 380 mV for Mo2S2 and 170 mV for Mo2Si2, the strength of the interactions between the electrophores is decreasing from the benzo to the dithiine and then the weakest one being the dihydro-1,4-disiline bridge.
As previously mentioned,18 different factors are known to affect the magnitude of the ΔE value between successive one-electron transfers, including electrostatic effects and electronic couplings. Thus, in order to have a more precise idea on the electrostatic contribution in the ΔE values, we investigated the redox behavior of the more soluble Mo2Si2 complex using a weakly coordinating electrolyte CH2Cl2–[Bu4N][B(C6H4(CF3)2)4] (or [Bu4N][BArF]).32 The choice of [Bu4N][BArF] instead of [Na][BarF] for this study is based on the higher solubility of [Bu4N][BArF] in CH2Cl2 compared to [Na][BArF]. As the [BArF] anion exhibits a very low ion-pairing strength with positively charged species in low polarity solvents such as dichloromethane while solvation dominates in high-polarity solvents such as DMSO, we performed this study only on the Mo2Si2 complex because it is the only well soluble bimetallic complex in dichloromethane.
In the weakly coordinating electrolyte CH2Cl2–[Bu4N][BArF], the CV of Mo2Si2 displays three well-resolved reversible processes with similar peak current intensity (Fig. 4b, red line). In these conditions, the potential difference between the first two oxidation waves is slightly affected by the nature of the supporting electrolyte, with a ΔE of 290 mV while it amounts to 230 mV with [Bu4N][PF6]. The small increase of the ΔE (E2 − E1) for Mo2Si2 (60 mV) indicates a limited electrostatic contribution in the ΔE value. It can be noticed also that the third redox process is significantly anodically shifted as the potential difference between the third and the second redox systems, ΔE = E3 − E2, reaches 1.1 V with [Bu4N][BArF] and only 700 mV with [Bu4N][PF6]. The increase of this shift indicates that the electrostatic tuning window of the potential differences (ΔE) is larger in the case of the oxidation of already charged species due to the very low ion pairing properties of [BArF]− in dichloromethane. Using the fluorinated weakly ion pairing anion [BArF]− as supporting electrolyte, no adsorption at the electrode is observed due to the increased solubility of the polycationic species. Moreover, due to the large anodic shift, the fourth redox system is now located outside the limit of the CH2Cl2–[Bu4N][B(C6H4(CF3)2)4] potential window.
Ground state DFT calculations
The optimized geometries of the different oxidation states of Mo2S2 and Mo2Si2, are reported in Fig. 5. The structure of the neutral Mo2S2 is found to be non-planar with a bending angle of 30° (angle taken from mid-points between the three pairs of S atoms from left to right). However, this angle decreases to about 15° to 18° (depending on the structure) for the mono-cation Mo2S2˙+ and is close to zero for the dication Mo2S22+. For all oxidation states, several conformers were located within a range of energy of a few kJ mol−1, distinguished by different orientations of the cyclopentadienyl groups. The cation radical, Mo2S2˙+, has a spin density symmetrically delocalized on both sides of the system as shown on the spin density plot in Fig. 6a. Additionally, this spin density has large contributions on the dithiine linkers. For the di-cationic species, Mo2S22+, as for the benzene linker, Mo2B2+,17 the electronic ground state is calculated to be a singlet where the open shell singlet (obtained from a decontaminated broken symmetry solution) is found to be only 0.7 kJ mol−1 lower in energy than the closed shell singlet, pointing at a possible coexistence of the two electronic configurations.
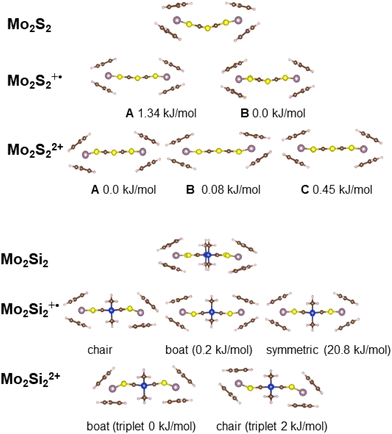 |
| Fig. 5 Representation of the different calculated, low energy conformations of Mo2Si2 and Mo2S2 and their oxidized species. | |
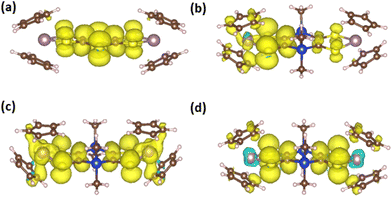 |
| Fig. 6 Spin density plots of (a) Mo2S2˙+, (b) Mo2Si2˙+ and (c) Mo2Si22(˙+) (triplet) both in their boat conformation, and (d) symmetric Mo2Si2˙+ (positive values in yellow and negative values in blue, with an isosurface of 0.001). | |
Contrary to Mo2S2, the structure of the neutral Mo2Si2 is found to be planar as can be seen in Fig. 5. The optimized geometries of the oxidized forms, Mo2Si2˙+ and Mo2Si22+ show the appearance of quasi-degenerate “boat” and “chair” conformations, reminiscent to what was observed for Mo2B˙+ and Mo2B2+.17 These structural similarities with Mo2B originate from the planar nature of the central ring in both systems. However, the benzene ring induces a conjugated structure between the two dithiolene moieties in Mo2B while the absence of π conjugation with the dihydro-1,4-disiline ring in Mo2Si2 isolates the two metallocentres. This aspect leads to non-trivial electronic structure in the case of the cation radical species Mo2Si2˙+ (Fig. 5). Indeed, the geometries of both conformers are computed to be symmetrical (or quasi-symmetrical in the chair conformer) but the radical electron is largely localized on one side of the molecule as can be seen in the spin density plots in Fig. 6b. Upon forcing the system to remain symmetric during the optimization process we did obtain a solution with a spin density delocalized on both side of the molecule (see Fig. 6c). However, this symmetric structure is calculated to be about 20 kJ mol−1 higher in energy than the other two previously mentioned. We believe this situation to be the illustration of the limitation of the mono-determinantal nature of the DFT. Another effect of the dihydro-1,4-disiline bridge compared to the benzene ring is that for the dicationic system, Mo2Si22+, the triplet ground state is calculated to be about 40 kJ mol−1 lower in energy than the closed shell singlet. For Mo2B2+, the triplet was located 18 kJ mol−1 higher than the closed shell singlet. Calculations on the broken symmetry solution of Mo2B2+ at both conformations (boat and chair) showed that an open-shell singlet state was very close in energy to the closed-shell singlet indicating the coexistence of the two electronic configurations. Here, in Mo2Si22+, the decontaminated broken symmetry solution, i.e. the open shell singlet solution, is found only 2 kJ mol−1 lower in energy than the triplet state. Thus, this theoretical description shows that the electronic ground state of Mo2Si22+ is open shell but it remains difficult to conclude whether it is a triplet or an open shell singlet.
Spectroelectrochemical investigations
In order to determine the UV–vis–NIR spectral signatures of the different redox states, spectroelectrochemical investigations were carried out on the two mononuclear complexes, Modt and MoSi2 as well as on the two binuclear complexes, Mo2S2 and Mo2Si2. The investigations were carried out on a dichloromethane solution of the most soluble complexes, Modt, MoSi2 and Mo2Si2, and in DMSO for both binuclear complexes, Mo2S2 and Mo2Si2, with 0.2 M [Bu4N][PF6] as the supporting electrolyte in all cases.
In dichloromethane the three neutral complexes, Modt, MoSi2 and Mo2Si2, exhibit absorption bands only in the UV-vis range with the lowest energy absorption band centered in the range 400 to 600 nm, close to that observed for monometallic33,34 and bimetallic complexes.18 This energy band is classically assigned to a ligand–metal charge transfer transition (LMCT) between the dithiolene and the molybdenum. Upon gradual oxidation of the mononuclear complex, Modt, besides the modifications in the UV region, the absorption bands initially centered at 518 nm gradually decrease and new absorption bands in the vis-NIR region centered at 660 and 1126 nm grow (Fig. 7a). This evolution upon oxidation is the one typically observed in all the mononuclear Cp2Mo(dithiolene) complexes with the lowest energy band in the range 1040–1150 nm.33–35 Then upon oxidation to the dicationic state, Modt2+, a novel absorption band grows centered at 750 nm (Fig. S4†). Similar evolution of the spectra is observed for the MoSi2 complex, with the signature of the radical cation species in the NIR region at 1150 nm (Fig. 7b).
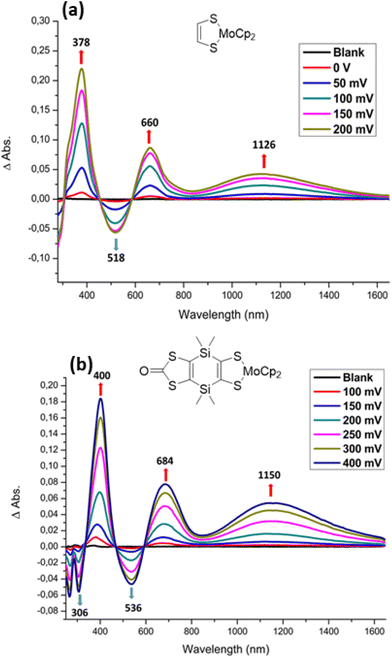 |
| Fig. 7 Differential UV-vis-NIR absorption spectra of the complexes Modt (a) and MoSi2 (b) monitored from neutral state to the monocation radical state upon electrochemical oxidation in CH2Cl2–[Bu4N][PF6]. | |
As the bimetallic complex involving the dithiine linker between the two metallacycles, Mo2S2, is easily oxidized in solution, we first reduced the solution to the neutral state through an electrolysis of the solution at −0.4 V vs. SCE during 15 min before performing the spectroelectrochemical investigations. Upon gradual oxidation to the monocationic state, Mo2S2˙+, besides the progressive disappearance of the band localized at 328 nm, new absorption bands in the UV-vis region at 420, 480 nm and in the NIR range at 800, 1154, 1442 nm are growing (Fig. 8a). Then upon oxidation to the second oxidation step, a novel band centered at 855 nm increases and the absorption band in the NIR region decreases (Fig. 8b).
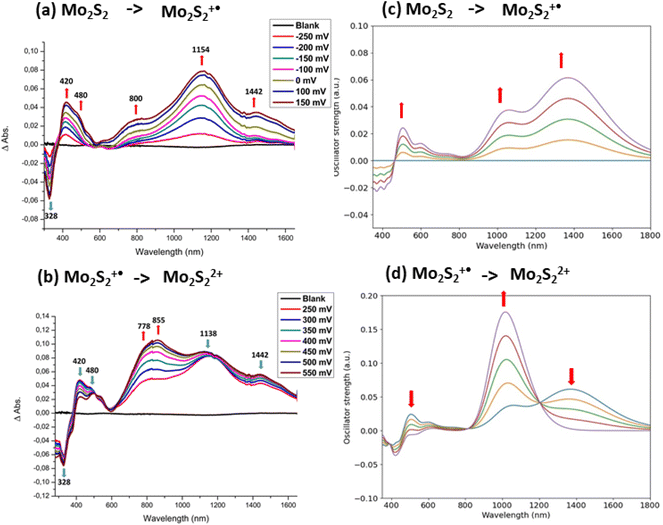 |
| Fig. 8 Experimental (a and b) and simulated (c and d) differential UV-vis-NIR absorption spectra of Mo2S2, monitored from neutral to radical cation state (a and c), from radical cation to dication state (b and d), calculated using 50 snapshots of a molecular dynamic simulation (see computational details). | |
The UV-vis-NIR absorption spectra of Mo2S2, Mo2S2˙+, and Mo2S22+ were computed and are shown in Fig. S5 in the ESI.† For the mono-cationic species, despite a broad absorption band in the NIR, several features are missing compared to the experimental differential UV-vis-NIR spectrum (Fig. 8a). As seen before, in the case of Mo2B˙+, such differences can be explained by the inclusion of several conformers.17 However, this is not the case here as the two conformers A and B of Mo2S2˙+ give very similar spectral signatures (Fig. S5†). Here, the non-planar structure induced by the dithiine linker can thermally evolve from one orientation to the other. Thus, in this case, instead of using only stable conformers, the dynamical evolution of the structure should be considered to improve the description of the absorption spectra. Molecular dynamics on the three charge states of Mo2S2 were performed and a sample of 50 snapshots of each dynamic simulation was selected to generate the averaged UV-vis-NIR spectra (see computational details). The resulting differential UV-vis-NIR spectra are presented in Fig. 8c and d. The calculated differential UV-vis-NIR from neutral Mo2S2 to monocation Mo2S2˙+ now reproduce most of the experimental features with a small red shift, namely a large absorption band at about 1400 nm and a smaller one at around 1050 nm. It appears that the absorption band at about 1050 correspond to structures with the spin localized on one side of the molecule (see spin density in Fig. 9) while the band at about 1400 nm has a spin density delocalised on both sides similar to that of the ground state. The nature of the low energy transitions differs and is identified for the band at 1050 nm as a complete charge transfer between the two sides of the molecule due to the breaking of the symmetry in this conformation, while the latter at 1400 nm exhibits a charge transfer in a symmetric fashion from the S atoms bound to the metal toward the central bridging S atoms (see representations in Fig. 9). Finally, the calculated differential UV-vis-NIR spectra from the monocation Mo2S2˙+ to the dication Mo2S22+ reproduce well the growth of the experimental band at about 800 nm, albeit from a red shifted to ca. 1000 nm, and correspond mostly to a charge transfer from the lateral S atoms to the bridging S atoms (see Fig. S5†).
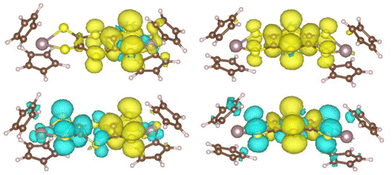 |
| Fig. 9 Characteristic spin densities (top) and charge density difference plots of the first excited state (bottom) of Mo2S2˙+ for snapshots absorbing around 1050 nm (left) and 1400 nm (right) (positive values in yellow and negative values in blue, with an isosurface of 0.001). | |
Upon gradual oxidation of the bimetallic complex Mo2Si2 from the neutral to the monocationic state (Fig. 10a), several modifications occur on the spectra in the UV-vis region such as the decrease of the LMCT band at 570 nm and the growth of two new absorption bands at 402 and 724 nm. Moreover, a broad absorption band in the NIR region at 1450 nm is growing (Fig. 10a). The main difference with the mononuclear complex MoSi2˙+ (Fig. 7b) is that this absorption is located at lower energy for Mo2Si2˙+ (1450 nm) compared to the one at 1150 nm for MoSi2˙+. Gradual oxidation of the mono-oxidized complex Mo2Si2˙+, to the bis-oxidized one, Mo2Si22+, induces the growing of a new absorption band at higher energy centered at 1190 nm (Fig. 10b). Interestingly, this spectral signature, especially the wavelength of this absorption band is now observed at an energy similar to the one observed for all the mononuclear complexes under the cation radical state (Fig. 7),31–33 suggesting that the dicationic state could be ascribed as two, essentially non interacting, radical cation sites.
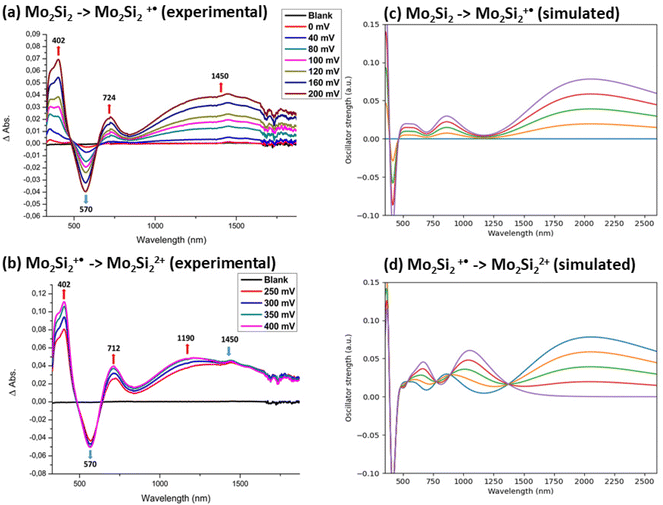 |
| Fig. 10 Experimental (a and b) and simulated (c and d) differential UV–vis–NIR absorption spectra Mo2Si2 monitored from neutral to radical cation state (a and c), from radical cation to dication state (b and d). | |
The theoretical differential UV-vis-NIR absorption spectra between Mo2Si2 and Mo2Si2˙+ (Fig. 10c) and Mo2Si2˙+ and Mo2Si22+ (Fig. 10d) were computed by artificially increasing the contributions of the oxidized species by 25 percent for each curve. In addition, the separated absorption spectra of the three states are presented in Fig. S6.† The calculated spectrum of Mo2Si2˙+ shows the contribution of three conformers, i.e. the two low energy conformers (boat and chair), which are responsible for the absorption band at about 900 nm, together with a broad band centered at 2100 which is due to the symmetric conformer (Fig. S6†). We believe that this symmetric electronic structure is essential to explain the experimental spectrum but it is not well described by DFT and leads to an absorption band largely red shifted. These two types of ground states, localized and symmetric, can be expected to coexist depending on the geometry (dynamical behaviour) and correspond to the broad band starting at 900 nm in the experimental spectrum. The analysis of the transitions reveals a MLCT character for the localized ground state, while for the symmetric ground state the transition corresponds mostly to a charge transfer from Si and S atoms to the bridging carbons and the two metals (see charge density difference plots in Fig. 11). On the other hand, for Mo2Si22+, the two main bands at ca. 700 nm and 1100 nm are well reproduced at this level of theory. The nature of the low energy bands corresponds to a MLCT as can be seen from the charge density difference plot in Fig. 11c. Note that dynamical protocol was also tested for Mo2Si2 but did not lead to the low energy band corresponding to the symmetric conformer. Nonetheless, in every case, we underline that the complexity of the experimental spectra can only be understood when considering several conformers with characteristic spin localisation.
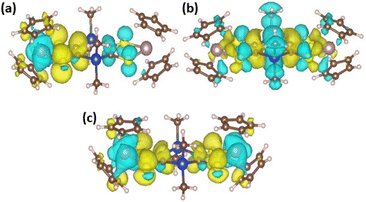 |
| Fig. 11 Charge density difference plot of the first excited state of (a) Mo2Si2˙+ (boat conformer), (b) Mo2Si2˙+ symmetric form and (c) Mo2Si22+ (boat conformation) corresponding to the low absorption band around 1100 nm (positive values in yellow and negative values in blue, with an isosurface of 0.001). | |
Spectral analysis of the chemically oxidized species
In order to bring some experimental support to these calculations for the oxidized species, we attempted to isolate the mono and dicationic complexes derived from Mo2S2 and Mo2Si2. We performed a chemical oxidation of Mo2S2 using two different approaches. We first succeeded in isolating the mono-oxidized species Mo2S2˙+ after oxidation of the neutral complex with one equivalent of [Fc][BF4] in CH2Cl2. The UV-vis-NIR spectrum in DMSO (Fig. S7a†), shows a band in the NIR region at 1150 nm, which corresponds to the formation of Mo2S2˙+ as observed during the spectroelectrochemical investigation after oxidation of the neutral complex Mo2S2 to the mono-oxidized one Mo2S2˙+ (see Fig. 8a). We also oxidized the Mo2S2 complex in H2SO4 in air. Then this solution is poured into an aqueous solution of KPF6 affording a dark purple precipitate. A part of this precipitate is soluble in acetonitrile and the UV-vis spectrum of this solution shows that it corresponds to the spectral signature of the dicationic species, deduced from the spectroelectrochemical studies (Fig. 8b), with a band centred at 848 nm Mo2S22+ (Fig. S7b†). As the dicationic salt is soluble in CH3CN we studied the redox behavior at higher potentials by cyclic voltammetry. Following the two redox processes at −0.26 and 0.11 V, associated with the successive oxidation of the complex from 0/+1 and +1/+2, another redox process can now be observed at higher potentials at +1.0 V vs. SCE. The EPR of this oxidized species, Mo2S22+, showed no signals associated with the presence of radical species indicating a closed-shell structure. These results are not only consistent with the spectroelectrochemical studies, but also to the theoretical calculations on the Mo2S2 complex for the formation of a closed shell singlet in the bis-oxidized complex Mo2S22+.
The chemical oxidation of the more soluble Mo2Si2 was performed with one or two equivalents of [Fc][BF4] in CH2Cl2. With only one equivalent of [Fc][BF4], the color of the solution changed to brown and after 1 h of stirring the addition of petroleum ether to the solution induced the formation of a precipitate. The UV-vis-NIR spectrum of this precipitate in a DMSO solution shows a broad band at 1240 nm together with a band at 500 nm (Fig. S8a†). The shape of this spectrum corresponds to the spectral signature of the mono-oxidized species, Mo2Si2˙+. Upon addition of 2 equivalents of [Fc][BF4], the color of the solution changed from purple to green, and a green precipitate appeared in the medium. The UV-vis-NIR spectrum of this precipitate in DMSO or CH3CN shows a broad band in the NIR region at 1160 nm (Fig. S8b†), which is consistent with the spectrum of the bis-oxidized complex Mo2Si22+ obtained from the spectroelectrochemical experiment (Fig. 10b). It is worthmentioning that the solution of the Mo2Si22+ complex either in CH3CN or DMSO easily decomposed, even under inert atmosphere. Interestingly, the EPR study carried out on the powder of [Mo2Si2][BF4]2 solubilized in CH3CN displays on the spectrum a signal at g = 2.0148 in accordance with the presence of an organic radical (Fig. S9†). The absence of any half-field signal around 1600–1700 Gauss in the frozen glass spectrum (66 K) confirms that Mo2Si22+ has a singlet ground state rather than a triplet one. This aligns with the theoretical calculations, indicating the formation of an open-shell singlet state in the bis(oxidized) complex Mo2Si22+ rather than a triplet state.
Conclusions
In summary, we present the synthesis and the characterizations of two bimetallic complexes where two molybdenocene (dithiolene) moieties are fused to a six membered ring. The investigations carried out on these bimetallic complexes allowed us to evidence some striking discrepancies depending on the nature of the central fused six-membered ring: 1,4-dithiine or dihydro-1,4-disiline compared to benzene in the corresponding complexes Mo2S2 and Mo2Si2vs.Mo2B. First, the nature of the bridge modifies the overall electron donating ability of these complexes, as the bimetallic complex with the sulfur bridge, Mo2S2 oxidizes at the lowest potential, followed by Mo2B and Mo2Si2. The geometries of these bimetallic complexes are also different, as anticipated from the structural characterization of monometallic complexes and by theoretical calculations. Indeed, the benzene and the dihydro-1,4-disiline bridges lead to planar neutral complexes Mo2B and Mo2Si2, while the dithiine bridge generates a non-planar, butterfly Mo2S2 neutral complex. However, some common features are shared by all three complexes: (i) the two electroactive molybdenocene(dithiolene) moieties oxidize sequentially up to the tetracationic state, (ii) upon gradual oxidation, a broad absorption band in the NIR region corresponding to an IVCT band is observed. Electrochemical and spectro-electrochemical studies reveal that the strongest electronic interaction is observed in Mo2B, followed by Mo2S2 and then Mo2Si2. DFT calculations showed that the fused complexes Mo2B, Mo2S2 and Mo2Si2 adopt several conformations in their mono- and bis(oxidized) state, which are responsible for their unique NIR absorption properties. Moreover, we show by a combination of DFT calculations and EPR studies of the chemically oxidized complexes that the two fused bis(oxidized) complexes Mo2Si22(˙+) as Mo2B2(˙+) exhibit a pronounced diradicaloid character while Mo2S2(2+) displays a closed-shell singlet state. The origin of the diradicaloid character in both complexes is different. For Mo2B, the neutral conjugated structure with the benzene ring evolves upon oxidation towards a non-conjugated one in the bis(oxidized) species while the two non-conjugated electrophores in the neutral Mo2Si2 stay non conjugated in the bis(oxidized) complex with the dihydro-1,4-disiline junction. Contrariwise, for Mo2S2 the antiaromatic connection between the two electrophores in the neutral complex becomes planar and conjugated in the bis(oxidized) complex leading to a closed-shell species.
This work opens the door for a wide variety of future investigations such as the elaboration of other electroactive bi- and trimetallic complexes with different metal ions (Co, Ni, Fe…). These results are also encouraging for the use of these novel ditopic dithiolene ligands for building conducting MOFs, as through-bond and through-space electronic interactions between the electrophores have been evidenced, an important prerequisite for the charge transport within MOFs built out of such ligands.
Experimental section
General
Chemicals and materials from commercial sources were used without further purification. All the reactions were performed under an argon atmosphere. NMR spectra were obtained in CDCl3 unless indicated otherwise. Chemical shifts are reported in ppm, 1H NMR spectra were referenced to residual CHCl3 (7.26 ppm) and 13C NMR spectra were referenced to CHCl3 (77.2 ppm). Melting points were measured on a Kofler hot-stage apparatus and are uncorrected. Mass spectra were recorded by the Centre Régional de Mesures Physiques de l'Ouest, Rennes. Methanol, acetonitrile and dichloromethane were dried using Inert pure solvent column device. Cyclic voltammetry has been performed in a three-electrode cell equipped with a platinum disk working electrode and a glass carbon as counter-electrode. CVs were carried out on a 10−3 M solution of complex in CH2Cl2 with [Bu4][NPF6] 0.1 M. Potentials were measured versus Saturated Calomel Electrode (SCE). The spectroelectrochemical setup was performed in CH2Cl2–[NBu4][PF6] 0.2 M using a Pt grid as the working electrode, a Pt wire as the counter electrode and SCE reference electrode. A Shimadzu 3600 plus spectrophotometer was used to record the UV-vis-NIR spectra. X-band EPR spectra were recorded on a Bruker EMX spectrometer.
Synthesis of bis([1,3]dithiolo)[4,5-b:4′,5′-e][1,4]dithiine-2,6-dithione 1.
(TBA)2[Zn(dmit)2] (179 mg, 0.25 mmol), diiodo-1,3-dithiole-2-thione (200 mg, 0.5 mmol), Cu2O (7.1 mg, 0.05 mmol), ethyl acetoacetate (86 μL, 0.68 mmol), and DMF (3 mL) were placed in a two-necked flask and heated to 80 °C overnight. The orange suspension was cooled down to room temperature and after the addition of 10 mL of CH2Cl2, the precipitate was filtered and washed with dichloromethane, water, and diethyl ether, yielding the product as an orange solid in 89% yield (147 mg, 0.44 mmol). Decomposition at 260 °C; HRMS (ASAP) calcd for C6S8 [M˙+] 327.7760, found 327.7755 (2 ppm).
Synthesis of bis([1,3]dithiolo)[4,5-b:4′,5′-e][1,4]dithiine-2,6-dione 2.
In a 50 mL flask containing bis([1,3]dithiolo)[4,5-b:4′,5′-e][1,4]dithiine-2,6-dithione 1 (120 mg, 0.36 mmol) in chloroform (20 mL), mercuric acetate (688 mg, 2.16 mmol) was added, and the suspension was stirred overnight at 70 °C. The suspension was filtered over Celite and washed with chloroform. The filtrate was evaporated to afford compound 2 as a yellow solid in 65% yield (70 mg, 0.23 mmol). Crystals of sufficient quality for X-ray diffraction were obtained by recrystallization in hot chloroform. Mp 254 °C; IR:
CO = 1681 cm−1; HRMS (ASAP) calcd for C6S8 [M˙+] 295.8217, found 295.8212 (2 ppm).24 This compound is not enough soluble in common organic solvents preventing any further characterization.
Synthesis of Mo2S2.
A solution of NaOMe, freshly prepared from sodium (161 mg, 7 mmol) in 20 mL of dry methanol, was added to a Schlenck tube containing compound 2 (200 mg, 0.67 mmol) in dry THF (20 mL) under an inert atmosphere. Upon the addition of MeONa, the color of the reaction mixture changed from light brown to black suspension. After stirring for 2 h at 45 °C, Cp2MoCl2 (398 mg, 1.34 mmol) was added to the medium, and the suspension was stirred overnight at 60 °C. The excess of NaOMe was quenched with water, and most of the solvents were removed under a vacuum. The residue was solubilized in CH2Cl2 and the organic phase was washed twice with water. Water was removed via cannula and the solvent was removed under vacuum. The precipitate was washed with diethyl ether, then with CH2Cl2 to afford Mo2S2 as a brown powder in 43% yield (245 mg, 0.35 mmol). Mp > 260 °C, HRMS (MALDI) calcd for C24H20S6Mo2 [M˙+] 695.7991, found 695.805 (8 ppm).
Synthesis of 4,4,8,8-tetramethyl-4,8-dihydro-[1,4]disilino[2,3-d:5,6-d′]bis([1,3]dithiole)-2,6-dithione 3.
To a Schlenck tube containing a solution of 1,3-dithiole (200 mg, 1.5 mmol) in dry THF (20 mL) at −78 °C, a freshly prepared solution of LDA (nBuLi 1.6 M solution, 2 mL, 3.2 mmol and diisopropylamine (0.46 mL, 3.3 mmol) in THF 5 mL at 0 °C), was added slowly. After 3 h at −78 °C, Me2SiCl2 (0.24 mL, 1.99 mmol) was added, and the temperature was gradually raised to room temperature overnight. The yellow precipitate was collected by filtration, then solubilized in CH2Cl2, and the organic layer was washed with water. After drying over MgSO4, the evaporation of the solvent afforded 3 a yellow powder in 56% yield (160 mg, 0.42 mmol). Crystals of sufficient quality for X-ray diffraction were obtained after slow evaporation of a CH2Cl2 solution. Mp > 260 °C. 1H NMR (300 MHz, CDCl3) δ 0.55 (s, 12H); 13C NMR (75 MHz, CDCl3) δ 216.4, 152.3, 0.4; HRMS (ASAP) calcd for C10H13S6Si2Mo [M + H]+ 380.8874, found 380.8873 (0 ppm); anal. calcd for [C10H12S6Si2·0.25 CH2Cl2]: C, 30.63; H, 3.13 found: C, 30.46; H, 3.47.
Synthesis of 4,4,8,8-tetramethyl-4,8-dihydro-[1,4]disilino[2,3-d:5,6-d′]bis([1,3]dithiole)-2,6-dione 4.
A suspension of compound 3 (150 mg, 0.39 mmol) and Hg(OAc)2 (753 mg, 2.36 mmol) in 15 mL of toluene were stirred under reflux overnight. The suspension was filtered over Celite and washed with dichloromethane. Evaporation of the solvent afforded white precipitate in quantitative yield (135 mg, 0.387 mmol). Crystals of sufficient quality for X-ray diffraction were obtained by slow evaporation of a CH2Cl2 solution. Mp 242 °C, 1H NMR (300 MHz, CDCl3) δ 0.51 (s, 12H); 13C NMR (75 MHz, CDCl3) δ 196.5, 141.1, 0.2; IR:
CO = 1646 cm−1; HRMS (ASAP) calcd for C10H12O2S4Si2 [M˙+] 347.9253, found 347.9250 (1 ppm); anal. calcd for [C10H12O2S4Si2]: C, 34.45; H, 3.47, found: C, 34.24; H, 3.49.
Synthesis of Modt.
To a two-neck flask containing compound 4 (70 mg, 0.2 mmol) dissolved in dry THF (7 mL) under an inert atmosphere, a solution of NaOMe (freshly prepared from sodium (48 mg, 2 mmol) in 7 mL of dry methanol) was added. The reaction mixture was stirred for 2 h at 45 °C, then the addition of Cp2MoCl2 (118 mg, 0.4 mmol), and the suspension was stirred overnight at 60 °C. Evaporation of solvent, then the residue solubilized in CH2Cl2. The organic layer was washed with water and dried with MgSO4. After the evaporation of the solvent, a flash column with pure CH2Cl2 afforded the product as a purple powder (40 mg, 0.12 mmol) in 31% yield. Crystals of sufficient quality for X-ray diffraction were obtained by slow evaporation of a dichloromethane solution. Mp 260 °C; 1H NMR (300 MHz, CDCl3) δ 6.21 (s, 2H), 5.25 (s, 10H). 13C NMR (75 MHz, CDCl3) δ 125.4, 97.9. HRMS (ASAP) calcd for C12H13S2Mo [M + H]+ 318.9507, found 318.9506 (0 ppm); anal. calcd for [C12H12S2Mo + 0.2THF]: C, 46.49; H, 4.15 found: C, 46.68; H, 4.12.
Synthesis of Mo2Si2 and MoSi2.
To a Schlenck tube containing a solution of compound 4 (200 mg, 0.57 mmol) in dry THF (20 mL) at −78 °C, nBuli (1.58 mL, 2.52 mmol) was added slowly and the mixture was stirred for 2 h at −78 °C. After the addition of Cp2MoCl2 (340 mg, 1.15 mmol) at −78 °C, the cold bath was removed and the suspension was stirred overnight at room temperature. Addition of dry methanol (25 mL) to the reaction mixture led to the formation of a precipitate which was filtered off. The precipitate was solubilized into CH2Cl2 and the organic phase was washed twice with water, and the water was removed via cannula. After evaporation of solvents, the precipitate was washed with diethyl ether affording the complex Mo2Si2 as a purple powder in 47% yield (200 mg, 0.27 mmol). Mp > 260 °C; 1H NMR (400 MHz, DMSO-d6) δ 5.17 (s, 20H), 0.13 (s, 12H); 13C NMR (101 MHz, DMSO-d6) δ 147.1, 97.7, 0.2. HRMS (MALDI) calcd for C28H32Si2S4Mo2 [M˙+] 747.9028, found 747.904 (2 ppm); anal. calcd for [C28H34OS4Si2Mo2·H2O]: C, 44.08; H, 4.49; S, 16.81. Found: C, 44.44; H, 4.49; S, 16.10.
MoSi2
.
The methanol solution was evaporated under vacuum, then the crude product was flash chromatographied on silica gel with pure CH2Cl2 to afford MoSi2 as a red powder in 6% yield. Crystals of sufficient quality for X-ray diffraction were obtained by slow evaporation of a dichloromethane solution. Mp > 260 °C; 1H NMR (400 MHz, CDCl3) δ 5.15 (s, 10H), 0.37 (s, 12H); 13C NMR (75 MHz, CDCl3) δ 145.03, 143.68, 104.71, 104.56, 97.78, 0.16. HRMS (ASAP) calcd for C19H23Si2S4OMo [M + H]+ 548.9218, found 548.9214 (1 ppm).
X-Ray crystallography
Data collections were performed on an APEXII Kappa-CCD (Bruker-AXS) diffractometer equipped with a CCD plate detector for compounds 2, 3 and Cp2MoS2, and on a D8 Venture (Bruker-AXS) diffractometer equipped with a CMOS-PHOTON detector for 4, 5, Modt, Cp2MoS2CO and MoSi2 using Mo-Kα radiation (λ = 0.71073 Å, multilayer monochromator). Structures were solved by dual-space algorithm using SHELXT program,36 and then refined with full-matrix least-squares methods based on F2 (SHELXL)program.37 All non-hydrogen atoms were refined with anisotropic atomic displacement parameters. H atoms were finally included in their calculated positions and treated as riding on their parent atom with constrained thermal parameter. Details of the final refinements are summarized in Tables S3 and S4 in ESI. CCDC 2333119–2333126 contain the supplementary crystallographic data for this paper.†
Computational details
All calculations were done using ORCA 5.0 program package.38 Geometry optimizations were performed at the B3LYP-D3/def2-TZVP level.39,40 Geometry optimizations were followed by frequency calculations to ensure the validity of the minima. UV-Visible absorption spectra were obtained with TD-DFT calculations at the CAM-B3LYP/def2-TZVP level,41 computing the first 35 electronic transitions. In addition, RIJCOSX approximations were used to accelerate the SCF procedures.42 In all steps, solvent (DMSO) effects were included through a Polarizable Continuum Model (PCM). Molecular dynamics calculations were performed using the r2SCAN-3c composite functional with a CPCM model of DMSO, a runtime of 5000 fs and timesteps of 1 fs at 300 K with a Nosé–Hoover chain thermostat.43
Conflicts of interest
There is no conflict of interest to declare.
Acknowledgements
Financial support was obtained from ANR (Paris France) under contracts no. 19-CE08-0029-02 and 20-CE09-0002-01, and a PhD grant (to K. Youssef) from Région Bretagne.
References
- G. Schrauzer and V. P. Mayweg, J. Am. Chem. Soc., 1962, 84, 3221 CrossRef CAS
.
-
(a) N. Robertson and L. Cronin, Coord. Chem. Rev., 2002, 227, 93–127 CrossRef CAS
;
(b) R. Kato, Chem. Rev., 2004, 104, 5319–5346 CrossRef CAS PubMed
;
(c) R. Kato, Bull. Chem. Soc. Jpn., 2014, 87, 355–374 CrossRef CAS
.
-
(a) J. R. Reynolds, C. A. Jolly, S. Krichene, P. Cassoux and C. Faulmann, Synth. Met., 1989, 31, 109–126 CrossRef CAS
;
(b) J. R. Reynolds, F. E. Karasz, C. P. Lillya and J. C. W. Chien, J. Chem. Soc., Chem. Commun., 1985, 268–269 RSC
;
(c) J. R. Reynolds, J. C. W. Chien and C. P. Lillya, Macromolecules, 1987, 20, 1184–1191 CrossRef CAS
;
(d) R. Vicente, J. Ribas, P. Cassoux and L. Valade, Synth. Met., 1986, 13, 265–280 CrossRef CAS
.
- Y. Sun, L. Qiu, L. Tang, H. Geng, H. Wang, F. Zhang, D. Huang, W. Xu, P. Yue, Y.-S. Guan, F. Jiao, Y. Sun, D. Tang, C. A. Di, Y. Yi and D. Zhu, Adv. Mater., 2016, 28, 3351–3358 CrossRef CAS PubMed
.
- W. Shi, G. Wu, K. Hippalgaonkar, J.-S. Wang, J. Xu and S.-W. Yang, J. Am. Chem. Soc., 2018, 140, 13200–13204 CrossRef CAS PubMed
.
- C. W. Dirk, M. Bousseau, P. H. Barrett, F. Moraes, F. Wudl and A. J. Heeger, Macromolecules, 1986, 19, 266–269 CrossRef CAS
.
-
(a) C. A. Downes and S. C. Marinescu, J. Am. Chem. Soc., 2015, 137, 13740–13743 CrossRef CAS PubMed
;
(b) C. A. Downes and S. C. Marinescu, Dalton Trans., 2016, 45, 19311–19321 RSC
.
-
(a) R. Matsuoka, R. Sakamoto, T. Kambe, K. Takada, T. Kusamoto and H. Nishihara, Chem. Commun., 2014, 50, 8137–8139 RSC
;
(b) B. Liu, W. Qiao and Z. Y. Wang, RSC Adv., 2015, 5, 6815–6822 RSC
.
-
(a) H. Poleschner, W. John, F. G. Hoppe, E. Fanghänel and S. Roth, J. Prakt. Chem., 1983, 325, 957–975 CrossRef CAS
;
(b) N. M. Rivera, E. M. Engler and R. R. Schumaker, J. Chem. Soc., 1979, 4, 184–185 Search PubMed
.
-
(a) J. Xie, J.-A. Pan, B. Cheng, T. Ma, A. S. Filatov, S. N. Patel, J. Park, D. V. Talapin and J. S. Anderson, J. Am. Chem. Soc., 2022, 144, 19026–19037 CrossRef CAS PubMed
;
(b) J. Xie, S. Ewing, J. N. Boyn, A. S. Filatov, B. Cheng, T. Ma, G. L. Grocke, N. Zhao, R. Itani, X. Sun, H. Cho, Z. Chen, K. W. Chapman, S. N. Patel, D. V. Talapin, J. Park, D. A. Mazziotti and J. S. Anderson, Nature, 2022, 611, 479–484 CrossRef CAS PubMed
.
- S. L. Xie, G. Skorupskii and M. Dincă, Chem. Rev., 2020, 120, 8536–8580 CrossRef PubMed
.
- G. Li, D. Zhu, X. Wang, Z. Su and M. R. Bryce, Chem. Soc. Rev., 2020, 49, 765–838 RSC
.
- L. Chen, M. Wang, F. Gloaguen, D. Zheng, P. Zhang and L. Sun, Chem. – Eur. J., 2012, 18, 13968–13973 CrossRef CAS PubMed
.
-
(a) H. Köpf and H. Balz, J. Organomet. Chem., 1990, 387, 77–81 CrossRef
;
(b) H. Balz, H. Köpf and J. Pickardt, J. Organomet. Chem., 1991, 417, 397–406 CrossRef CAS
;
(c) K. Arumugam, R. Yu, D. Villagrán, T. G. Gray, J. T. Mague and J. P. Donahue, Inorg. Chem., 2008, 47, 5570–5572 CrossRef CAS PubMed
;
(d) K. Arumugam, M. C. Shaw, P. Chandrasekaran, D. Villagrán, T. G. Gray, J. T. Mague and J. P. Donahue, Inorg. Chem., 2009, 48, 10591–10607 CrossRef CAS PubMed
.
- M. Murata, S. Kaji, H. Nishimura, A. Wakamiya and Y. Murata, Eur. J. Inorg. Chem., 2016, 3228–3232 CrossRef CAS
.
-
(a) M. Nomura and M. Fourmigué, Inorg. Chem., 2008, 47, 1301–1312 CrossRef CAS PubMed
;
(b) B. H. Zhu, Y. Shibata, S. Muratsugu, Y. Yamanoi and H. Nishihara, Angew. Chem., Int. Ed., 2009, 48, 3858–3861 CrossRef CAS PubMed
.
- K. Youssef, C. Poidevin, A. Vacher, Y. Le Gal, O. Charpentier, M. Fourmigué, A. Fihey, F. Barrière, T. Roisnel and D. Lorcy, Chem. – Eur. J., 2023, e202300584 CrossRef CAS PubMed
.
-
(a) A. Vacher, Y. Le Gal, T. Roisnel, V. Dorcet, T. Devic, F. Barrière and D. Lorcy, Organometallics, 2019, 38, 4399–4408 CrossRef CAS
;
(b) K. Youssef, A. Vacher, F. Barrière, T. Roisnel and D. Lorcy, Polyhedron, 2022, 226, 116086 CrossRef CAS
;
(c) N. Bellec, A. Vacher, F. Barrière, Z. Xu, T. Roisnel and D. Lorcy, Inorg. Chem., 2015, 54(10), 5013–5020 CrossRef CAS PubMed
.
- W. Shi, G. Wu, X. Yong, T. Deng, J.-S. Wang, J.-C. Zheng, J. Xu, M. B. Sullivan and S.-W. Yang, ACS Appl. Mater. Interfaces, 2018, 10(41), 35306–35315 CrossRef CAS PubMed
.
-
(a) E. Aqad, J. Y. Becker, J. Bernstein, A. Ellern, V. Khodorkovsky and L. Shapiro, J. Chem. Soc., Chem. Commun., 1994, 2775–2776 RSC
;
(b) L. M. Goldenberg, V. Yu, V. Khodorkovsky, J. Y. Becker, M. R. Bryce and M. C. Petty, J. Mater. Chem., 1995, 5, 191–192 RSC
.
- F. Biaso, M. Geoffroy, E. Canadell, P. Auban-Senzier, E. Levillain, M. Fourmigué and N. Avarvari, Chem. – Eur. J., 2007, 13, 5394–5400 CrossRef CAS PubMed
.
- N. Svenstrup and J. Becher, Synthesis, 1995, 215–235 CrossRef CAS
.
- H. Yamochi and G. Saito, Synth. Met., 1997, 85, 1467–1468 CrossRef CAS
.
- J. Sun, X. Lu, J. Shao, Z. Cui, Y. Shao, G. Jiang, W. Yu and X. Shao, RSC Adv., 2013, 3, 10193–10196 RSC
.
-
(a) R. Gompper, J. Hock, K. Polborn, E. Dormann and H. Winter, Adv. Mater., 1995, 7, 41–43 CrossRef CAS
;
(b) B. Domercq, T. Devic, M. Fourmigué, P. Auban-Senzier and E. Canadell, J. Mater. Chem., 2001, 11, 1570–1575 RSC
.
- J. Larsen and K. Bechgaard, J. Org. Chem., 1987, 52, 3285–3288 CrossRef CAS
.
- Z. Lu, H. Liu, X. Lv, W. Lv, Y. Liu and K. Zhu, Chem. – Eur. J., 2023, 29, e202300101 CrossRef CAS PubMed
.
-
(a) K. Kobayashi and C. L. Gajurel, J. Sulfur Chem., 1986, 7, 123–148 CAS
;
(b) S. I. Etkind and T. M. Swager, Synthesis, 2022, 54, 4843–4863 CrossRef CAS
.
- S. Kim, Y. Kwon, J.-P. Lee, S.-Y. Choi and J. Choo, J. Mol. Struct., 2003, 655, 451–458 CrossRef CAS
.
-
(a) A. E. Bruce, M. R. M. Bruce, A. Sclafani and D. R. Tyler, Organometallics, 1984, 3, 1610–1614 CrossRef CAS
;
(b) H. Köpf, S. K. S. Hazari and M. Leitner, Z. Naturforsch., B: Anorg. Chem., Org. Chem., 1978, 33, 1398–1403 CrossRef
.
- T. Klapötke and H. Köpf, Z. Anorg. Allg. Chem., 1988, 558, 217–222 CrossRef
.
-
(a) F. Barrière and W. E. Geiger, J. Am. Chem. Soc., 2006, 128, 3980–3989 CrossRef PubMed
;
(b) W. E. Geiger and F. Barrière, Acc. Chem. Res., 2010, 43, 1030–1039 CrossRef CAS PubMed
.
- J. K. Hsu, C. J. Bonangelino, S. P. Kaiwar, C. M. Boggs, J. C. Fettinger and R. S. Pilato, Inorg. Chem., 1996, 35, 4743–4751 CrossRef CAS
.
- A. L. Whalley, A. J. Blake, D. Collison, E. S. Davie, H. J. Disley, M. Helliwell, F. E. Mabbs, J. McMaster, C. Wilson and C. D. Garner, Dalton Trans., 2011, 40, 10457–10472 RSC
.
- A. J. Taylor, E. S. Davies, J. A. Weinstein, I. V. Sazanovich, O. V. Bouganov, S. A. Tikhomirov, M. Towrie, J. McMaster and C. D. Garner, Inorg. Chem., 2012, 51, 13181–13194 CrossRef CAS PubMed
.
- G. M. Sheldrick, Acta Crystallogr., Sect. A: Found. Adv., 2015, 71, 3–8 CrossRef PubMed
.
- G. M. Sheldrick, Acta Crystallogr., Sect. C: Struct. Chem., 2015, 71, 3–8 Search PubMed
.
- F. Neese, F. Wennmohs, U. Becker and C. Riplinger, J. Chem. Phys., 2020, 152, 224108 CrossRef CAS PubMed
.
- A. D. Becke, J. Chem. Phys., 1993, 98, 5648–5652 CrossRef CAS
.
- F. Weigand and R. Ahlrichs, Phys. Chem. Chem. Phys., 2005, 7, 3297–3305 RSC
.
- T. Yanai, D. P. Tew and N. C. Handy, Chem. Phys. Lett., 2004, 393, 51–57 CrossRef CAS
.
- F. Neese, F. Wennmohs, A. Hansen and U. Becker, Chem. Phys., 2009, 356, 98–109 CrossRef CAS
.
- S. Grimme, A. Hansen, S. Ehlert and J. M. Mewes, J. Chem. Phys., 2021, 154, 064103 CrossRef CAS PubMed
.
|
This journal is © The Royal Society of Chemistry 2024 |
Click here to see how this site uses Cookies. View our privacy policy here.