DOI:
10.1039/D4DT01384K
(Paper)
Dalton Trans., 2024,
53, 12080-12089
Tetracycline: structural characterization and antimicrobial properties of its water-soluble di-anionic bi-sodium salt†
Received
10th May 2024
, Accepted 23rd May 2024
First published on 3rd June 2024
Abstract
The new water-soluble di-anionic bi-sodium salt of tetracycline (TC), an antibiotic in clinical use, with the formula {[TC]2−[Na+(MeOH)(H2O)] [Na+]·(H2O)}n (TCNa) was synthesized. The compound was characterized by m.p., attenuated total reflectance-Fourier transform infra-red (ATR-FTIR) spectroscopy, and ultraviolet (UV) and proton nuclear magnetic resonance (1H NMR) spectroscopy in the solid state and in solution. The molecular weight (MW) was determined by cryoscopy. The crystal structure of TCNa was also determined by X-ray crystallography. The antibacterial activity of TCNa was evaluated against the bacterial species Pseudomonas aeruginosa (P. aeruginosa), Escherichia coli (E. coli), Staphylococcus epidermidis (S. epidermidis) and Staphylococcus aureus (S. aureus) by means of minimum inhibitory concentration (MIC), minimum bactericidal concentration (MBC) and inhibition zones (IZs). Moreover, the ability of the compound to eradicate biofilm formation was also evaluated. The results are compared with those obtained for the commercially available drug TCH2. The in vitro and in vivo toxicities of TCNa were tested against human corneal epithelial cells (HCECs) and Artemia salina.
Introduction
Tetracycline is a widely used antibiotic with a broad spectrum of bactericidal properties against both Gram-positive and Gram-negative bacteria.1,2 Tetracyclines, initially employed as topical drops and ointments, represent a group of antibiotics utilized in the treatment of blepharitis.3 In particular, tetracycline ointment continues to be utilized as a treatment for trachoma (chlamydial infections) due to its status as the sole topical treatment available for numerous years.4 The current applications of tetracycline include both therapy and prophylaxis against human infections such as ocular diseases.5 In particular, tetracycline is used in the treatment or inhibition of corneal ulceration.6 Recently, an ophthalmic solution containing tetracycline was patented against eye surface inflammation.7 Although tetracycline is usually available as a peroral drug only, it is characterized by low hydrophilicity and poor absorption after administration.8 One of the methods used to overcome its low solubility in water is its hydrochlorination.9 As has already been highlighted, bacteria have acquired resistance to tetracyclines.10 Consequently, it is imperative to advance the development of novel tetracycline-based antibiotics capable of addressing the challenge posed by bacterial resistance. Tetracycline derivatives exhibit multiple functional groups (Scheme 1, labeled A–D) that can serve as potential ligand sites for complex formation leading to novel antibiotics.
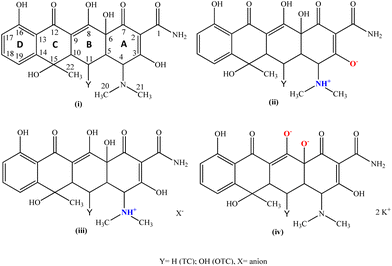 |
| Scheme 1 The structural motifs of oxy-tetracyclines, OTCs, (Y = OH) (neutral (i), zwitterionic (ii), cationic (iii) and dianionic (iv)) and the corresponding ones of tetracycline (TC) Y = H (zwitterionic (ii) and cationic (iii)). | |
Four main types of oxy-tetracycline (OTC) moieties have been found and characterized by X-ray analysis to date. These include the (i) neutral tetracyclines (Scheme 1),11,12 (ii) zwitterionic tetracyclines (Scheme 1),11,13–16 (iii) cationic tetracyclines (Scheme 1)17–20 and (iv) di-anionic tetracyclines (Scheme 1).13
However, only zwitterionic and cationic structures of tetracyclines have been reported to date: (ii) zwitterionic tetracyclines (Scheme 1)11,14–16 and (iii) cationic tetracyclines (Scheme 1).17,18,21
During our investigations into the design and creation of innovative formulations aimed at overcoming bacterial resistance experienced by existing antibiotics,22–30 we have successfully synthesized and characterized a modified version of the commercially available drug tetracycline (TCH2 = tetracycline) (Scheme 1). The crystal structure of {[TC]2−[Na+(MeOH)(H2O)] [Na+]·(H2O)} (TCNa) was refined from an X-ray diffraction data set. TCNa was characterized by melting point (m.p.) and ATR-FTIR, 1H-NMR and UV–vis spectroscopic techniques. The molecular weight of the compound was determined by cryoscopy in solution. The Gram-negative strains Pseudomonas aeruginosa (P. aeruginosa) and Escherichia coli (E. coli), as well as the Gram-positive bacteria, including Staphylococcus epidermidis (S. epidermidis) and Staphylococcus aureus (S. aureus), prevalent in microbial keratitis, were subjected to an assessment of the antibacterial activity of TCNa. In order to accomplish this, we assessed the minimum inhibitory concentration (MIC), minimum bactericidal concentration (MBC), and inhibition zone (IZ). Additionally, we evaluated the impact of TCNa on the formation of biofilm by both Staphylococcus aureus (S. aureus) and Pseudomonas aeruginosa (P. aeruginosa). The in vitro toxicity of TCNa was estimated using normal human corneal epithelial cells (HCECs). Additionally, the Artemia salina model was employed to evaluate the in vivo toxicity of TCNa.
Results and discussion
General aspects
Tetracycline reacts with sodium hydroxide (NaOH) in the presence of carbon disulfide (CS2) in methanol (MeOH) in a 1
:
3
:
3 molar ratio (Scheme 2). The yellow precipitate was crystallized by slow evaporation of MeOH solution. TCNa is stable when it is stored under ambient conditions in the dark and it is soluble in H2O, MeOH, MeCN, DMSO and acetone. The water solubility of TCNa was verified using UV–vis spectroscopy. Fig. S1† illustrates the UV–vis spectra of TCNa in water.
 |
| Scheme 2 Synthetic route of TCNa. | |
Solid state studies
Crystal and molecular structure of TCNa.
A molecular diagram of TCNa along with selected bond lengths and angles are shown in Fig. 1.
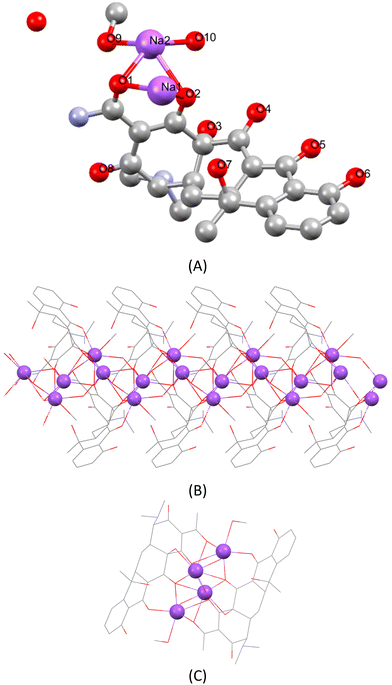 |
| Fig. 1 (A) Molecular diagram of TCNa. Selected bond lengths (Å) and angles [°]: Na1–O1: 2.444(3), Na1–O2: 2.462(3), Na2–O1: 2.256(2), Na2–O2: 2.530(3), Na2–O9: 2.464(3), Na2–O10: 2.306(3), O9–C23: 1.428(4), O1–C1: 1.236(3), O2–C7: 1.235(3), O3–C6: 1.401(3), O4–C8: 1.285(3), O5–C12: 1.259(3), O6–C16: 1.340(3), O7–C15: 1.449(3), O1–Na1–O2: 65.03(7), O1–Na2–O2: 66.58(7), Na2–O9: 93.29(8), O1–Na2–O10: 140.71(8), O2–Na2–O9: 125.98(8), O2–Na2–O10: 80.33(7), O9–Na2–O10: 89.35(8); 1D-ribbon supramolecular assembly along the a-axis (B) and the b-axis (C). | |
Thus far, only the zwitterionic form of tetracycline (TC) has been documented. In contrast, oxytetracycline (OTC) has exhibited a broader range of crystallized forms, including neutral, zwitterionic, cationic, and di-anionic, all characterized by X-ray crystallography.11–16,19 While the dianionic oxytetracycline (OTC) in the potassium salt form has been previously reported by Jogun et al.,13 with the formula {(OTC)2−2(K1+)2(H2O)2(CH3OH)}, the TCNa {[TC]2−[Na+(MeOH)(H2O)] [Na+]·(H2O)}n represents the first reported example of a di-anionic and non-zwitterionic form for tetracycline itself. Table 1 provides a summary of the reported C–O bond lengths for both tetracycline (TC) and oxytetracycline (OTC) across various forms documented thus far. Table 2 presents a compilation of the average bond lengths of C1–O, C3–O, C4–O, and C13–O measured so far for both tetracycline (TC) and oxy-tetracycline (OTC) in their reported forms (neutral, zwitterionic, cationic, and dianionic).
Table 1 The C–O bond lengths reported for the TCs and OTCs to date in their different forms
Name |
CCDC code |
Type |
C1–O (Å) |
C3–O (Å) |
C4–O (Å) |
C13–O (Å) |
Ref. |
This work.
|
TCs |
TCNa
|
Di-anionic |
1.236 |
1.235 |
1.285 |
1.251 |
|
|
TCYURT10 |
Zwitterionic |
1.241 |
1.231 |
1.316 |
1.277 |
14
|
|
TETCYH01 |
Zwitterionic |
1.254 |
1.244 |
1.339 |
1.245 |
15
|
|
TETCYH10 |
Zwitterionic |
1.262 |
1.237 |
1.333 |
1.237 |
11
|
|
TETCYH11 |
Zwitterionic |
1.248 |
1.233 |
1.347 |
1.247 |
16
|
|
TETCYH12 |
Zwitterionic |
1.255 |
1.233 |
1.334 |
1.241 |
16
|
Average |
|
|
1.252 ± 0.007 |
1.236 ± 0.005 |
1.334 ± 0.010 |
1.249 ± 0.014 |
|
OTCs |
OXYTET |
Neutral |
1.274 |
1.233 |
1.337 |
1.304 |
13
|
|
OXYTET01 |
Neutral |
1.27 |
1.229 |
1.34 |
1.306 |
12
|
|
OXYTETD |
Zwitterionic |
1.248 |
1.25 |
1.334 |
1.233 |
11
|
|
OXTETH10 |
Zwitterionic |
1.277 |
1.235 |
1.338 |
1.236 |
13
|
|
OTETCB |
Cationic |
1.333 |
1.235 |
1.356 |
1.264 |
19
|
|
OXTETK |
Di-anionic |
1.236 |
1.261 |
1.258 |
1.271 |
13
|
Table 2 C1–O, C3–O, C4–O and C13–O average bond lengths (Å) found in TCs and OTCs, respectively
Type |
C1–O (Å) |
C3–O (Å) |
C4–O (Å) |
C13–O (Å) |
Neutral |
1.272 ± 0.004 |
1.231 ± 0.004 |
1.339 ± 0.003 |
1.305 ± 0.002 |
Zwitterionic |
1.255 ± 0.009 |
1.238 ± 0.005 |
1.334 ± 0.007 |
1.245 ± 0.011 |
Cationic |
1.333 |
1.235 |
1.356 |
1.264 |
Di-anionic |
1.236 ± 0.000 |
1.248 ± 0.025 |
1.272 ± 0.026 |
1.261 ± 0.020 |
The asymmetric unit of TCNa includes one TC2− ion, which interacts with two Na+ ions through the oxygen atom of the amide bond and the corresponding one of the A-ring (Scheme 1 and Fig. 1A). Two consequent {[TC]2−[Na+(MeOH)(H2O)] [Na+]·(H2O)} units form a dimer, which are linked to each other via ten Na–O contacts, involving four sodium cations and two TC molecules. Finally, a 1D ribbon supramolecular assembly of the covalent polymer {[TC]2−[Na+(MeOH)(H2O)] [Na+]·(H2O)}n is established although an extended network is formed, held together by Na–O contacts ranging from 2.256 to 2.767 Å, ensuring that all Na atoms are 6-coordinated (Fig. 1B and C). This extended network of Na–O contacts, however, affects the C–O bond lengths, rendering their comparison (in Tables 1 and 2) pointless.
Vibrational spectroscopy.
The ν(H–Caromatic) and ν(H–Caliphatic) band vibrations in the FTIR spectrum of TCH2 and TCNa are observed at 3063–3048, 2955, and 2925 cm−1 and at 3070, 2936, and 2920 cm−1, respectively29,31,32 (Fig. S2†). The vibrational bands at 1639 and 1599 cm−1 in the FTIR spectrum of TCH2 are assigned to the amide ν(O
C(1)) and ν(O
C(7)) bond vibrations, respectively (Scheme 1).29,31,32 These bands are shifted at 1674 and 1609 cm−1, respectively, in the spectrum of TCNa. The pronounced change is a result of tetracycline coordinating with Na+via the O(C1) and O(C7) atoms. The vibration band at 1397 cm−1 is attributed to ν(O–C(8)), which is shifted at 1419 cm−1 in the spectrum of TCNa. This is a consequence of the deprotonation of the hydroxyl group at the C(8) position, leading to an increase in the bond order of the C(8)–O bond. The vibrational band observed at 1302 cm−1 in the IR spectrum of TCH2 is assigned to the ν(O–C(3)) bond, while in the case of TCNa, a slight shift is observed at 1297 cm−1.
Solution studies
Molecular weight (MW) measurement.
The molecular formula in solution was first determined by measuring its MW using the cryoscopic method in DMSO/ddw (1
:
49 v/v) (ddw = double distilled water). A solution of 1 μL of TCNa (1 mg per 100 μL of DMSO) was diluted to 49 μL of ddw. The molecular weight of TCNa was determined to be 573.4 g mol−1, consistent with the calculated value obtained from X-ray analysis. The MW of TCNa was found to be 573.4 g mol−1 {(C22H22N2O8)2− 2Na+ (MeOH) 2(H2O)} (calc. 556.44 g mol−1). Therefore, it can be inferred that the formulation of TCNa is retained in the solution.
UV-vis and 1H NMR spectroscopy methods.
Methanol and DMSO were selected as solvents for recording UV-vis and 1H NMR spectra, instead of water, to facilitate a comparison between insoluble free TCH2 and TCNa.
Stability studies of TCNa.
The stability of TCNa was examined through 1H NMR in CD3OD over a 48-hour period (Fig. S3†). The period of 48 h was chosen for stability testing since the biological experiments require 48 h of incubation with TCNa. No changes were observed between the initial 1H NMR spectra and the corresponding ones after 48 h, confirming the retention of TCNa in solution.
UV-Vis spectroscopy.
The UV-Vis absorption spectra of TCNa and TCH2 were recorded in DMSO solution (Fig. S4†). The absorption band at 365 nm (log
ε = 2.8) in the spectrum of TCH2 is shifted to 381 nm in the spectrum of TCNa (log
ε = 3.7). The band at 265 nm (log ε = 2.8) in the spectrum of TCH2 remains unshifted in TCNa (265 nm, log
ε = 3.7). These bands are assigned to π* ← π transitions.
1H NMR spectroscopy.
The 1H NMR spectra of TCH2 and TCNa in MeOH-d4 were recorded (Fig. S5†). In the spectrum of TCH2, the resonance signals at 7.56–7.52, 7.19–7.17 and 6.96–6.94 ppm are assigned to H[C(18)aromatic], H[C(19)aromatic] and H[C(17)aromatic], respectively (Scheme 1).33 These signals are shifted downfield in the case of the spectrum of TCNa at 7.25–7.21 ppm, 6.92–6.90 ppm and 6.71–6.69 ppm, respectively. The resonance signal at 4.13 ppm is assigned to H[OC(8)], which is absent in TCNa, due to the deprotonation of the hydroxylic group. The resonance signal of the 6,H[OC(16)],H[OC(15)],H[OC(3)] proton remains unchanged at 4.86 ppm. The resonance signals at 3.06–2.95 ppm in the spectrum of TCH2 are assigned to the protons of H[C(10)] and they are shifted at 2.74–2.61 ppm in the case of TCNa. The signal at 2.29–2.26 ppm in the spectrum of TCH2 is assigned to the methyl protons of H[H3C(20)] and H[H3C(21)] and it is shifted downfield at 2.07–2.03 ppm in the case of TCNa. The signal at 2.00–1.91 ppm is assigned to the H[C(11)] proton in the spectrum of TCH2 and it is shifted at 1.92–1.88 ppm in the spectrum of TCNa. The signal at 1.65 ppm in the spectrum of TCH2 is assigned to the methyl protons of H[H3C(22)] and it is shifted downfield at 1.49 ppm in the case of TCNa. The new signals at 2.38 and 1.31 ppm in the spectrum of TCNa are assigned to the coordinated methanol (Fig. S5†).
Biological activity
Antibacterial activity.
The antimicrobial efficacy of TCNa was assessed through the minimum inhibitory concentration (MIC), minimum bactericidal concentration (MBC), inhibition zone (IZ) and biofilm elimination concentration (BEC) against Gram-negative bacterial strains Pseudomonas aeruginosa (P. aeruginosa) and Escherichia coli (E. coli) and the Gram-positive ones Staphylococcus epidermidis (S. epidermidis) and Staphylococcus aureus (S. aureus).
Minimum inhibitory concentration (MIC).
Since TCH2 is recommended for the treatment of microbial keratitis (MK), the effectiveness of TCNa was assessed against bacteria abundant in MK namely E. coli, P. aeruginosa, S. epidermidis and S. aureus upon their incubation for 20 h.23,25,27,30,33–35 The MIC values of TCH2 were previously established.29
TCH2 and TCNa were suspended in water for the antibacterial studies. TCNa demonstrated superior antimicrobial activity compared to TCH2, showing an increase of 3.2-fold against P. aeruginosa, 14.7-fold against E. coli, and 2.4-fold against S. aureus (Table 3 and Fig. S6†). However, there was a reduction of 0.4-fold in the MIC value when TCNa was utilized in place of TCH2 against S. epidermidis. The MIC values of TCNa against Gram-negative bacteria are lower than those against Gram-positive bacteria. It is very interesting that TCNa demonstrates nanomolar MIC values against E. coli and S. aureus (380 and 560 nM, respectively), whereas the corresponding values for TCH2 against E. coli and S. aureus are 5600 and 1320 nM, respectively. It is important to note that bacteria such as E. coli and S. aureus, associated with microbial keratitis, constitute 15% and 5–36% (15%) of bacterial colonies, respectively.36
Table 3 Minimum inhibitory concentrations (MICs), minimum bactericidal concentrations (MBCs), inhibition zones (IZs), and biofilm elimination concentration (BEC) of TCNa and TCH2, against P. aeruginosa, E. coli, S. epidermidis and S. aureus. Their IC50 values against human corneal epithelial cells (HCECs) are indicated
Compounds |
Gram negative |
Gram positive |
Ref. |
|
P. aeruginosa
|
E. coli
|
S. epidermidis
|
S. aureus
|
|
In this work.
|
MIC (μM) |
TCNa
|
8.92 ± 1.78 |
0.38 ± 0.10 |
87.36 ± 17.40 |
0.56 ± 0.07 |
|
TCH2 |
28.60 ± 4.90 |
5.60 ± 1.33 |
37.80 ± 14.06 |
1.32 ± 0.34 |
29
|
MBC (μM) |
TCNa
|
80.0 ± 0.0 |
26.00 ± 3.7 |
330.0 ± 24.0 |
21.7 ± 3.3 |
|
TCH2 |
92.0 ± 18.0 |
150.0 ± 25.3 |
171.4 ± 29.1 |
10.0 ± 0.0 |
29
|
MBC/MIC |
TCNa
|
9.0 |
68.4 |
3.8 |
38.8 |
|
TCH2 |
3.2 |
26.6 |
4.5 |
7.6 |
29
|
IZ (mm) |
TCNa
|
15.5 ± 0.9 |
26.0 ± 3.4 |
12.6 ± 1.2 |
35.0 ± 2.4 |
|
TCH2 |
15.2 ± 1.4 |
22.5 ± 0.6 |
11.8 ± 0.5 |
34.3 ± 1.8 |
29
|
BEC (μM) |
TCNa
|
704.4 ± 91.2 |
— |
— |
959.8 ± 63.5 |
|
TCH2 |
427 ± 84 |
— |
— |
>2304 |
29
|
IC50 (μM) HCECs |
TCNa
|
>300 |
|
TCH2 |
>300 |
|
Bacteria with MIC values less than 50 μM are classified as susceptible to an antimicrobial agent, whereas those with MIC values exceeding 100 μM are considered resistant.37 Thus, P. aeruginosa, E. coli and S. aureus exhibit susceptibility to TCNa, as the free drug TCH2 does. However, S. epidermidis is considered resistant to TCNa (Table 3).
Efforts to determine whether TCNa exhibits bactericidal or bacteriostatic properties involved the utilization of the minimum bactericidal concentration (MBC). The MBC value represents the concentration needed to eliminate 99.9% of the initial bacterial inoculum. The MBC values complement MIC, as MIC indicates the minimum concentration of an antimicrobial agent that inhibits growth, while MBC indicates the minimum concentration of the antimicrobial agent that leads to microbial death.38
The MBC values for TCNa and TCH2 range from 22 to 330 μM and 10 to 171 μM, respectively, against all tested bacterial strains (Table 3 and Fig. S7†). TCNa exhibits lower MBC values against Gram-negative bacteria compared to TCH2. Conversely, for Gram-positive bacteria, TCH2 demonstrates lower MBC values than TCNa.
To determine whether the formulation can be categorized as bacteriostatic (MBC/MIC ≥ 4), where the organism is inhibited but not killed, or bactericidal (MBC/MIC ≤ 2), where 99.9% of microorganisms are killed, the MBC/MIC ratios were calculated.22–30,39 For TCNa, the MBC/MIC ratios are ≥4, indicating that it can be classified as bacteriostatic. This implies that TCNa inhibits the growth of bacteria without causing their death, similar to TCH2 (Table 3).
The disc diffusion method was additionally employed to evaluate the antimicrobial activity of TCNavs. TCH2 at a concentration of 1 mM against P. aeruginosa, E. coli, S. epidermidis and S. aureus (Table 3 and Fig. 2). There was a notable increase in the inhibition zone size compared to the corresponding one of TCH2 against both Gram-negative and positive bacteria when TCNa was used. This confirms the strongest activity of TCNa compared to the antibiotic drug, TCH2. The range of the diameter of the inhibition zones formed by TCNa or TCH2 lies between 12.6 and 35.0 mm and 11.5 and 33.0 mm, respectively (Table 3).
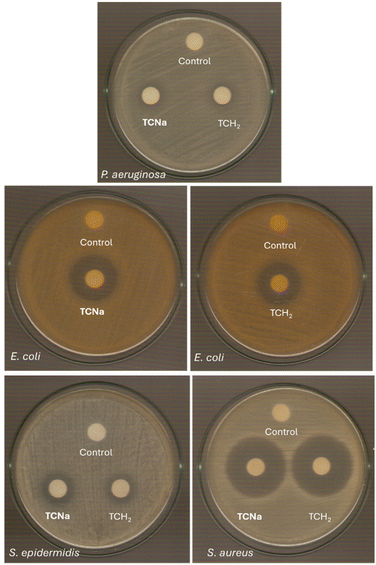 |
| Fig. 2 IZs developed in agar plates of P. aeruginosa, E. coli, S. epidermidis and S. aureus by TCNa and TCH2 at 1 mM. | |
Based on the inhibition zone diameter, bacteria can be categorized into three groups: sensitive if they cause an IZ ≥ 17 mm, intermediate if they cause an IZ between 13 mm and 16 mm, and resistant if the IZ is ≤12 mm.22–30,39 As a result, E. coli and S. aureus are characterized as sensitive to both TCNa and TCH2, while P. aeruginosa and S. epidermidis fall into the category of intermediate sensitivity.
Effect of TCNa on biofilm formation.
Eliminating biofilms presents a significant challenge in addressing bacterial infections like those caused by P. aeruginosa and S. aureus on the cornea. This is particularly crucial as 80% of clinical infections are associated with biofilms. In general, biofilms have demonstrated greater resilience compared to planktonic cell systems, primarily because of their high impermeability to external antimicrobial agents.40
The efficacy of TCNa in inhibiting biofilm formation was assessed using the biofilm elimination concentration (BEC), while the formation of bacterial biofilms was tracked using crystal violet staining22–31 (Table 3 and Fig. S8†). The BEC value is defined as the concentration required to achieving a minimum 99.9% reduction in the viability of biofilm-forming bacteria. The BEC values for TCNa are 704 and 960 μM, respectively, against P. aeruginosa and S. aureus (Table 1 and Fig. S8†). TCNa demonstrates greater efficiency (up to 2-fold) than TCH2 against S. aureus biofilms, while its efficacy in eliminating P. aeruginosa biofilms is lower compared to TCH2.
Assessment of the in vitro toxicity of TCNa on human corneal epithelial cells (HCECs).
The initial stage of assessing agent biocompatibility involves cytotoxicity investigations using cell culture-based methods. Cytotoxicity assays provide both qualitative and quantitative assessments of an agent's potential hazard.41 For bacterial keratitis, employing human corneal epithelial cells (HCECs) appears more suitable, given that human lens epithelial cells are the preferred model for studying intraocular infections.41
The cytotoxicity of TCNa and TCH2 was assessed over a 48 hour period, revealing their non-toxic characteristics against HCECs at a concentration of up to 300 μM. According to the ISO 10993-5 guidelines, an agent is considered non-cytotoxic when the percentage of cell viability exceeds 70%.42 For HCECs treated with TCNa and TCH2, the percentages of viable cells are (83.1 ± 3.5) % and (69.3 ± 4.3)%, respectively, when they are treated with a concentration of 300 μM. Consequently, TCNa should be regarded as an in vitro non-toxic agent.
Moreover, the selectivity index (SI) serves as a relative indicator of agent safety. The SI is a ratio between the agent dosage inducing toxicity and the dosage producing a therapeutic effect (SIMIC = IC50 (against HCECs)/MIC). A higher therapeutic index indicates a greater desired effect compared to undesired cellular toxicity. Conversely, a narrow therapeutic index (low ratio) means there is a small margin between achieving therapeutic success and encountering toxicity.23,30,43
The selectivity index (SI) range for TCNa is between 3 and 789, whereas for TCH2, it ranges from 8 to 227. TCNa exhibits a higher selectivity index and a more desirable effect compared to TCH2. Despite the narrower therapeutic window of TCNa for S. epidermidis, the corresponding ones for P. aeruginosa, E. coli, and S. aureus are 3, 14.7, and 2.4 times higher than that of TCH2, respectively.
In vivo toxicity evaluation by brine shrimp Artemia salina.
Artemia salina, a zooplanktonic crustacean, is regarded as a model organism for in vivo toxicological tests by the United States Environmental Protection Agency (US-EPA).44,45 Furthermore, this acute model assay demonstrates a strong correlation with toxicity data obtained from rodents and humans.44 The survival rates (%) of Artemia salina larvae exposed to TCNa are assessed at concentrations corresponding to the minimum inhibitory concentration (MICmin = 0.5 μM), observed against the strains tested here, as well as the maximum concentration (MICmax = 150 μM), and twice the maximum concentration (2 × MICmax = 300 μM), over a 24 hour period.
The survival rates are 100.0 ± 0.0, 94.4 ± 6.9 and 71.8 ± 7.0 % for 0.5, 150 and 300 μM concentrations, respectively. Furthermore, the antibiotic TCH2 has been evaluated at concentrations up to 100 μM, with no observed impact on the survival of A. salina.31 The in vivo toxicity results align with those of the in vitro studies (see above), as no toxic effects of the substance were observed on human corneal epithelial cells (HCECs) up to a concentration of 300 μM. Similarly, the survival rate of A salina exceeds 70% when incubated at 300 μM. This suggests that TCNa does not exhibit in vivo toxic behavior.
Conclusion
At present, fifty percent of the drug approvals issued by the United States Food and Drug Administration (US FDA) involve pharmaceutical ingredients formulated as salts, and the same percentage of the top 200 prescription drugs consists of pharmaceutical salts.46 In particular, sodium is among the most commonly preferred cations utilized in oral or parenteral formulations, as well as in topical products.46 Moreover, 95% of the existing medications for ophthalmic infections are comprised of eye drops and hydrophilic drugs. Water-soluble hydrophilic drugs offer notably higher bioavailability and are more easily administered to the eye in drop form. Consequently, the development of a water-soluble hydrophilic tetracycline formulation is a matter of significant research and technological and financial importance.
Furthermore, the corneal epithelium, conversely, allows only hydrophilic compounds with molecular weights below 500 g mol−1 to penetrate due to its paracellular pore diameter of 2.0 ± 0.2 nm.47
The chemical characteristics of tetracycline were altered within the compound {[TC]2−[Na+(MeOH)(H2O)] [Na+]·(H2O)} (TCNa) to enhance its water solubility and biocompatibility for use in eye drops. In addition, its molecular weight is 556.44 g mol−1, which falls within the range of molecular weights that can permeate the corneal epithelium through paracellular pores.
As a result, TCNa was evaluated for the treatment of ophthalmic infections, particularly microbial keratitis. TCNa exhibited enhanced antimicrobial efficacy compared to TCH2, as evidenced by MIC values, showing a 3.2-fold increase against P. aeruginosa, a 14.7-fold increase against E. coli, and a 2.4-fold increase against S. aureus.
The aforementioned bacteria are susceptible to TCNa. Moreover, TCNa can be categorized as bacteriostatic, indicating that it inhibits the growth of bacteria without causing their death, similarly to TCH2. The range of the diameter of the inhibition zones of TCNa is higher than that of TCH2. Based on the length of the IZ diameter, E. coli and S. aureus are characterized as sensitive to both TCNa and TCH2, while P. aeruginosa and S. epidermidis fall into the category of intermediate sensitivity. TCNa demonstrates greater elimination efficiency (up to 2-fold) than TCH2 against S. aureus biofilms.
The biocompatibility of the new formulation was first assessed using an in vitro cytotoxicity assay, followed by an in vivo cytotoxicity assay using the Artemia salina test. The findings confirmed that both TCNa and TCH2 exhibited non-toxicity against HCECs when tested at concentrations of up to 300 μM. This conclusion was drawn from the cell viability percentage exceeding 70%, in accordance with the ISO 10993-5 guidelines. Additionally, the findings from the in vivo tests were consistent with those from the in vitro studies. Overall, TCNa demonstrates a wider therapeutic window, exhibiting a more favorable balance between desirable effects and toxicity compared to TCH2.
In conclusion, TCNa represents a water-soluble, non-toxic bacteriostatic formulation effective against the bacteria associated with microbial keratitis, which have shown susceptibility to TCNa. This positions TCNa as a potential candidate for the development of new pharmaceutical ingredients for eye drops aimed at treating microbial keratitis.
Experimental
Materials and instruments
All solvents utilized were of reagent grade. The tryptone tryptophan medium, beef extract powder, bacteriological peptone, and soy peptone were procured from Biolife. Agar and yeast extract were acquired from Fluka Analytical. Sodium chloride, D(+)-glucose, dipotassium hydrogen phosphate trihydrate, trichloroacetic acid, and acetic acid were sourced from Merck. Dulbecco's modified Eagle's medium (DMEM), fetal bovine serum, glutamine, and trypsin were obtained from Gibco, Glasgow, UK. Sulforhodamine B was purchased from Alfa Aesar. Phosphate buffer saline (PBS) was acquired from Sigma-Aldrich. Dimethyl sulfoxide was sourced from Riedel-de Haën. Melting points were determined in open tubes using a Stuart Scientific apparatus and remained uncorrected. IR spectra spanning the region of 4000–370 cm−1 were recorded using a Cary 670 FTIR spectrometer, Agilent Technologies. The 1H-NMR spectra were captured on a Bruker AC 400 MHz FT-NMR instrument in DMSO-d6 solution. Electronic absorption spectra were obtained using a UV-1600 PC series spectrophotometer from VWR.
Synthesis of TCNa
0.240 g (0.5 mmol) of tetracycline hydrochloride was dissolved in 10 mL of distilled water (ddH2O), followed by the addition of 0.5 mL of 1 N KOH (0.5 mmol) solution, resulting in the formation of a yellow precipitate. The precipitate was filtered, dried, and transferred to a flask containing 1.5 mmol NaOH and 1.5 mmol CS2 in 20 mL of methanol (MeOH). The mixture was stirred at 0 °C for 3 hours and then filtered. Crystals were observed after two days, dried at room temperature, and stored in a freezer.
TCNa: yield 30%; melting point: >250 °C; elemental analysis found: C: 51.28; H: 5.55; N: 5.64%; calculated for (C23H28N2Na2O10)n: C: 51.31; H: 5.24; N: 5.20%. IR (cm−1): 3412br, 2935 m, 1610 m, 1578vs, 1465 m, 1420vs, 1361s, 1320 m, 1298 m, 1259 m, 1160 m, 1131 m, 1113 m, 1067 m, 1035 m, 1003s, 971s, 936 m, 877s, 852s, 756s, 697s, 616 m, 596 m, 568 m, 485 m, 462s, 425s; 1H NMR (ppm) in MeOH-d4: 7.25–7.21 (t, H[C(18)aromatic], J = 8.56, 7.58), 6.92–6.90 (d, H[C(19)aromatic], J = 7.34), 6.71–6.69 (d, H[C(17)aromatic], J = 7.09), 4.89 (s, 6,H[OC(16)],H[OC(15)], H[OC(3)]), 2.74–2.61 (m, H[C(10)]), 2.38 (s, coordinated methanol), 2.07–2.03 (m, H[H3C(20)] and H[H3C(21)]), 1.92–1.88 (m, H[C(11)]), 1.49 (s, H[H3C(22)]), 1.31(s, coordinated methanol); UV-vis (DMSO): λmax = 271 (log
ε = 3.8), 369 (log
ε = 3.8)
X-ray structure determination
Single crystals of TCNa suitable for crystal structure analysis were obtained through slow evaporation of their mother liquids at room temperature. They were then mounted at room temperature on a Bruker Kappa APEX2 diffractometer equipped with a triumph monochromator using MoKα radiation. Unit cell dimensions were determined and refined by utilizing the angular settings of at least 200 high-intensity reflections (>10 σ(I)) within the range of 2.9 < 2θ < 27.2°. Intensity data were recorded using φ and ω scans. All crystals exhibited no decay during the data collection process. The frames collected for each crystal were integrated using the Bruker SAINT software package48 using a narrow-frame algorithm. Data were corrected for absorption using the numerical method (SADABS) based on crystal dimensions.49 The structure was solved using the SUPERFLIP package50 incorporated into Crystals. Data refinement (full-matrix least-squares methods on F2) and all subsequent calculations were carried out using the Crystals version 14.40b program package. All non-hydrogen atoms were refined anisotropically. Hydrogen atoms were located by difference maps at their expected positions and refined using soft constraints. By the end of the refinement, they were positioned geometrically using riding constraints to bonded atoms.
TCNa: C23H28N2Na2O10, H2O, MW = 556.48, monoclinic, space group P21, a = 13.701 (7), b = 6.178 (3), c = 14.782 (8) Å, β = 111.527 (12)°, V = 1163.9(10) Å3, Z = 2, T = 295 K, ρ(calc.) = 1.588 g cm−3, μ = 0.157 mm−1, F(000) = 584. 14
569 reflections measured, 4372 unique (Rint = 0.020). The final R1 = 0.0379 (for 3532 reflections with I > 2 s(I)) and wR(F2) = 0.0562 (all data) S = 1.00 (CCDC 2330616).†
Biological tests
Bacterial strains.
For the antibacterial experiments, the strains Staphylococcus epidermidis (ATCC® 14990™), S. aureus subsp. aureus (ATCC® 25923™), P. aeruginosa and Escherichia coli were used. The Gram-negative bacterial strains P. aeruginosa and Escherichia coli were kindly donated by the Laboratory of Biochemistry at the University of Ioannina, Greece.
Effect of TCNa on the growth of microbial strains.
This study was performed as previously described.22–30 In short, bacterial strains were streaked onto trypticase soy agar plates and then incubated at 37 °C for 18–24 h. Following incubation, three to five isolated colonies showing similar morphological characteristics were selected from fresh agar plates using a sterile loop and transferred into tubes containing 2 mL of sterile saline solution. The optical density at 620 nm was adjusted to 0.1, corresponding to 108 colony-forming units per milliliter (cfu mL−1). The final inoculum size for broth dilution was set at 5 × 105 cfu mL−1. Each culture solution, including both the TCNa-treated and positive/negative controls, had a total volume of 2 mL. TCNa concentrations ranged from 0.05 to 150 μM. After incubation for 20 hours, growth was assessed. The minimal inhibitory concentration (MIC) was determined as the concentration of the compound that inhibits visible bacterial growth. The MIC value was obtained by plotting the optical density of the solution at 620 nm against different concentrations.22–30
Minimum bactericidal concentration testing.
This study was performed as previously described.22–30 The bacteria were first cultured with TCNa in broth for 20 h. MBC values were determined in duplicate by subculturing 4 μL of the broth onto agar plates. Colony growth indicated non-bactericidal activity of the compound. Therefore, the minimum bactericidal concentration was defined as the lowest concentration at which the tested compound completely inhibited microbial growth.22–30
Determination of the inhibition zone (IZ) through the agar disk-diffusion method.
This study was performed according to the standard procedure as previously described.22–30 Subsequently, agar plates were inoculated with a standardized inoculum (at 108 cfu ml−1) of the tested microorganism. Filter paper disks (9 mm in diameter), previously soaked with TCNa and TCH2 (at 1 mM concentration), were positioned on the agar surface. The Petri dishes were then incubated for 20 h, after which the diameters of the inhibition zones were measured.22–30
Effect of TCNa on biofilm formation.
Bacteria at a density of 1.3 × 106 cfu mL−1 were introduced into LB agar broth medium for P. aeruginosa or tryptic soy broth for S. aureus (total volume = 1500 μL) in test tubes and incubated for 20 hours at 37 °C. Subsequently, the contents of each test tube were carefully removed, and the tubes were rinsed with 1 mL of 0.9% saline solution followed by the addition of 2 mL of broth. The negative control consisted solely of broth. Next, the bacteria were exposed to TCNa at concentrations ranging from 20 to 900 μM for 20 h at 37 °C. The contents of each tube were then poured out and washed three times with 1 mL of methanol and 2 mL of 0.9% saline solution before being left to dry. The tubes were stained with a 0.1% w/v crystal violet solution for 15 minutes. Excess stain was removed by rinsing with 1 mL of methanol and 2 mL of 0.9% saline solution, followed by 3 mL of 0.9% saline solution. The tubes were left to dry for 24 hours, and the bound crystal violet stain was released by adding 30% glacial acetic acid. The optical density of the resulting solution was then measured at 550 nm to determine the biofilm biomass.22–30
Sulforhodamine B assay.
These studies were performed in accordance with the previously reported method.22–30 Briefly, HCECs were seeded in a 96-well plate at a density of 10
000 cells per well and after 24 h of cell incubation, the compounds were added in the concentration range of 50–300 μM for TCNa and TCH2. HCECs were exposed to compounds for a period of 48 h.
Evaluation of toxicity with the brine shrimp assay.
The brine shrimp assay was performed as previously described.22–30 For the toxicity experiments, brine shrimp eggs (Artemia salina) were purchased from Ocean Nutrition. Initially, 1 g of cysts was hydrated in freshwater for one hour in a separating funnel or cone-shaped container. Seawater was prepared by dissolving 17 g of sea salt in 500 ml of distilled water. The cone was provided with adequate aeration for 48 hours at room temperature and under continuous illumination. After hatching, nauplii released from the eggshells were gathered at the illuminated side of the cone (near the light source) using a micropipette. The larvae were separated from the eggs by transferring them into small beakers containing 0.9% NaCl solution. An aliquot (0.1 mL) containing approximately 5 to 15 nauplii was dispensed into each well of a 24-well plate, and micelles were added to each well at concentrations corresponding to the minimum inhibitory concentration (MICmin = 0.5 μM), observed against the strains tested here, as well as the maximum concentration (MICmax = 150 μM), and twice the maximum concentration (2 × MICmax = 300 μM), over a 24-hour period. The brine shrimps were observed after 24 hours using a stereoscope. Larvae were considered deceased if they showed no internal or external movement within 10 seconds of observation. Each experiment was repeated three times.
Author contributions
Conceptualization, S. K. Hadjikakou; investigation, A. S. Tsigara, C. N. Banti and A. Hatzidimitriou; methodology, C. N. Banti, A. Hatzidimitriou and S. K. Hadjikakou; supervision and validation, S. K. Hadjikakou; writing – original draft, C. N. Banti and S. K. Hadjikakou; writing – review & editing, C. N. Banti and S. K. Hadjikakou.
Conflicts of interest
The authors declare no conflict of interest.
Acknowledgements
(i) This work was carried out in fulfilment of the requirements for the master thesis of Ms. A. S. T. according to the curriculum of the International Graduate Program in “Biological Inorganic Chemistry”, which operates at the University of Ioannina within the collaboration of the Departments of Chemistry of the Universities of Ioannina, Athens, Thessaloniki, Patras, Crete and the Department of Chemistry of the University of Cyprus (https://bic.chem.uoi.gr/BIC-En/index-en.html) under the supervision of Prof. S. K. H. (ii) The International PhD Program, entitled “Biological Inorganic Chemistry (BIC)”, is acknowledged. This program is co-financed by Greece and the European Union (European Social Fund – ESF) through the Operational Program “Human Resources Development, Education and Lifelong Learning 2014-2020” in the context of subproject 6 “Biological Inorganic Chemistry (BIC)” (MIS 5162213). This paper was supported by the Special Account for Research Funds (Research Committee) of the University of Ioannina.
References
- D. Ramdhani, S. A. F. Kusuma, D. Sediana and A. P. H. B. Khumairoh, Comparative study of cefixime and tetracycline as an evaluation policy driven by the antibiotic resistance crisis in Indonesia, Sci. Rep., 2021, 11, 18461 CrossRef CAS PubMed.
- T. S. B. Møller, G. Liu, H. B. Hartman, M. H. Rau, S. Mortensen, K. Thamsborg, A. E. Johansen, M. O. A. Sommer, L. Guardabassi, M. G. Poolman and J. E. Olsen, Global responses to oxytetracycline treatment in tetracycline-resistant Escherichia coli, Sci. Rep., 2020, 10, 8438 CrossRef PubMed.
- R. R. Vernhardsdottir, M. S. Magn, L. Hynnekleiv, N. Lagali, D. A. Dartt, J. Vehof, C. J. Jackson and T. P. Utheim, Antibiotic treatment for dry eye disease related to meibomian gland dysfunction and blepharitis – A review, Ocul. Surf., 2022, 26, 211–221 CrossRef PubMed.
- D. Bremond-Gignac, F. Chiambaretta and S. Milazzo, A European Perspective on Topical Ophthalmic Antibiotics: Current and Evolving Options, Ophthalmol. Eye Dis., 2011, 3, 29–43 CrossRef CAS PubMed.
- T. J. Federici, The non-antibiotic properties of tetracyclines: Clinical potential in ophthalmic disease, Pharmacol. Res., 2011, 64, 614–623 CrossRef CAS PubMed.
- R. A. Ralph, Tetracyclines and the treatment of corneal stromal ulceration: A review, Cornea, 2000, 19, 274–277 CrossRef CAS PubMed.
-
J. P. Gilbard, Opthalmic solution with tetracycline for topical treatment of dry eye disease, Canadian Patent, 2339792C, 2000 Search PubMed.
-
R. Vardanyan and V. Hruby, Antibiotics, In Synthesis of Best-Seller Drugs, ed R. Vardanyan and V. Hruby, Academic Press, 2016, ch 30, pp. 573–643, ISBN 9780124114920 Search PubMed.
- A. I. Caço, F. Varanda, M. J. Pratas de Melo, A. M. A. Dias, R. Dohrn and I. M. Marrucho, Solubility of Antibiotics in Different Solvents. Part II. Non-Hydrochloride Forms of Tetracycline and Ciprofloxacin, Ind. Eng. Chem. Res., 2008, 47, 8083–8089 CrossRef.
- I. Chopra and M. Roberts, Tetracycline Antibiotics: Mode of Action, Applications, Molecular Biology, and Epidemiology of Bacterial Resistance, Microbiol. Mol. Biol. Rev., 2001, 232–260 CrossRef CAS PubMed.
- J. J. Stezowski, Chemical-Structural Properties of Tetracycline Derivatives. 1. Molecular Structure and Conformation of the Free Base Derivatives, J. Am. Chem. Soc., 1976, 98, 6012 CrossRef CAS PubMed.
- R. Prewo and J. J. Stezowski, Chemical-Structural Properties of Tetracycline Derivatives. 3. The Integrity of the Conformation of the Nonionized Free Base, J. Am. Chem. Soc., 1977, 99, 1117 CrossRef CAS PubMed.
- K. H. Jogun and J. J. Stezowski, Chemical-Structural Properties of Tetracycline Derivatives. 2. Coordination and Conformational Aspects of Oxytetracycline Metal Ion Complexation, J. Am. Chem. Soc., 1976, 98, 6018 CrossRef CAS PubMed.
- G. J. Palenik and M. Mathew, Structural Studies of Tetracyclines. Crystal and Molecular Structure of Tetracycline-Urea Tetrahydrate, J. Am. Chem. Soc., 1978, 100, 4464 CrossRef CAS.
- M. R. Caira, L. R. Nassimbeni and J. C. Russell, The crystal and molecular structure of tetracycline hexahydrate, Acta Crystallogr., Sect. B: Struct. Crystallogr. Cryst. Chem., 1977, 33, 1171 CrossRef.
- F. W. Heinemann, C. F. Leypold, C. R. Roman, M. O. Schmitt and S. Schneider, X-Ray, Crystallography of Tetracycline, Doxycycline and Sancycline, J. Chem. Cryst., 2013, 43, 213 CrossRef CAS.
- K. Kamiya, M. Asai, Y. Wada and M. Nishikawa, Opposite chirality of pillaromycin A to tetracyclines: The X-ray analysis of achromycin hydrochloride, Experientia, 1971, 27, 363 CrossRef CAS PubMed.
- R. Prewo and J. J. Stezowski, Chemical-Structural Properties of Tetracycline Derivatives. 9. 7-Chlorotetracycline Derivatives with Modified Stereochemistry, J. Am. Chem. Soc., 1980, 102, 7015 CrossRef CAS.
- K. H. Jogun and J. J. Stezowski, Cryst. Struct. Commun., 1976, 5, 381 CAS.
- J. Robertson, I. Robertson, P. F. Eiland and R. Pepinsky, X-Ray, Measurements of Terramycin Salts, J. Am. Chem. Soc., 1952, 74, 841 CrossRef CAS.
- S. Inouye and Y. Iitaka, Crystallographic data on the molecular complexes of tetracycline salts, Acta Crystallogr., 1964, 17, 207 CrossRef CAS.
- I. Sainis, C. N. Banti, A. M. Owczarzak, L. Kyros, N. Kourkoumelis, M. Kubicki and S. K. Hadjikakou, New antibacterial, non-genotoxic materials, derived from the functionalization of the anti-thyroid drug methimazole with silver ions, J. Inorg. Biochem., 2016, 160, 114–124 CrossRef CAS PubMed.
- I. Milionis, C. N. Banti, I. Sainis, C. P. Raptopoulou, V. Psycharis, N. Kourkoumelis and S. K. Hadjikakou, Silver ciprofloxacin (CIPAG): a successful combination of chemically modified antibiotic in inorganic–organic hybrid, J. Biol. Inorg. Chem., 2018, 23, 705–723 CrossRef CAS PubMed.
- M.-E. K. Stathopoulou, C. N. Banti, N. Kourkoumelis, A. G. Hatzidimitriou, A. G. Kalampounias and S. K. Hadjikakou, Silver complex of salicylic acid and its hydrogel-cream in wound healing chemotherapy, J. Inorg. Biochem., 2018, 181, 41–55 CrossRef CAS PubMed.
- M. P. Chrysouli, C. N. Banti, I Milionis, D. Koumasi, C. P. Raptopoulou, V. Psycharis, I. Sainis and S. K. Hadjikakou, A water-soluble silver(I) formulation as an effective disinfectant of contact lenses cases, Mater. Sci. Eng., C, 2018, 93, 902–910 CrossRef CAS PubMed.
- I. Ketikidis, C. N. Banti, N. Kourkoumelis, C. G. Tsiafoulis, C. Papachristodoulou, A. G. Kalampounias and S. K. Hadjikakou, Conjugation of Penicillin-G with Silver(I) Ions Expands Its Antimicrobial Activity against Gram negative Bacteria, Antibiotics, 2020, 9, 25 CrossRef CAS PubMed.
- A. K. Rossos, C. N. Banti, A. Kalampounias, C. Papachristodoulou, K. Kordatos, P. Zoumpoulakis, T. Mavromoustakos, N. Kourkoumelis and S. K. Hadjikakou, pHEMA@AGMNA-1: A novel material for the development of antibacterial contact lens, Mater. Sci. Eng., C, 2020, 111, 110770 CrossRef CAS PubMed.
- M. P. Chrysouli, C. N. Banti, N. Kourkoumelis, E. E. Moushi, A. J. Tasiopoulos, A. Douvalis, C. Papachristodoulou, A. G. Hatzidimitriou, T. Bakas and S. K. Hadjikakou, Ciprofloxacin conjugated to diphenyltin(IV): a novel formulation with enhanced antimicrobial activity, Dalton Trans., 2020, 49, 11522–11535 RSC.
- A. Meretoudi, C. N. Banti, P. Siafarika, A. G. Kalampounias and S. K. Hadjikakou, Tetracycline Water Soluble Formulations with Enhanced Antimicrobial Activity, Antibiotics, 2020, 9, 845 CrossRef CAS PubMed.
- C. N. Banti, M. Kapetana, C. Papachristodoulou, C. Raptopoulou, V. Psycharis, P. Zoumpoulakis, T. Mavromoustakos and S. K. Hadjikakou, Hydrogels containing water soluble conjugates of silver(I) ions with amino acids, metabolites or natural products for non infectious contact lenses, Dalton Trans., 2021, 50, 13712–13727 RSC.
- P. Z. Trialoni, Z.-C. M. Fyrigou, C. N. Banti and S. K. Hadjikakou, Conjugation of tetracycline and penicillin with Sb(V) and Ag(I) against breast cancer cells, Main Group Met. Chem., 2022, 45, 152–168 CrossRef CAS.
- C. F. Leypold, M. Reiher, G. Brehm, M. O. Schmitt, S. Schneider, P. Matousek and M. Towrie, Tetracycline and derivatives – assignment of IR and Raman spectra via DFT calculations, Phys. Chem., 2003, 5, 1149–1157 CAS.
- D. E. Williamson and G. W. Jr Everett, A proton nuclear magnetic resonance study of the site of metal binding in tetracycline, J. Am. Chem. Soc., 1975, 97, 2397–2407 CrossRef CAS PubMed.
- K. F. Tabbara, H. F. El-Sheikh and B. Aabed, Extended wear contact lens related bacterial keratitis, Br. J. Ophthalmol., 2001, 85, 842–847 CrossRef PubMed.
- F. Stapleton and N. Carnt, Contact lens-related microbial keratitis: how have epidemiology and genetics helped us with pathogenesis and prophylaxis, Eye, 2012, 26, 185–193 CrossRef CAS PubMed.
- D. S. J. Ting, C. S. Ho, R. Deshmukh, D. G. Said and H. S. Dua, Infectious keratitis: an update on epidemiology, causative microorganisms, risk factors, and antimicrobial resistance, Eye, 2021, 35, 1084–1101 CrossRef PubMed.
- D. L. Shungu, E. Weinberg and H. H. Gadebusch, Tentative interpretive standards for disk diffusion susceptibility testing with norfloxacin (MK-0366, AM-715), Antimicrob. Agents Chemother., 1983, 23, 256–260 CrossRef CAS PubMed.
- M. Azizi-Lalabadi, A. Ehsani, B. Divband and M. Alizadeh-Sani, Antimicrobial activity of Titanium dioxide and Zinc oxide nanoparticles supported in 4A zeolite and evaluation the morphological characteristic, Sci. Rep., 2019, 9, 17439 CrossRef PubMed.
-
M. Motyl, K. Dorso, J. Barrett and R. Giacobbe, Basic microbiological techniques used in antibacterial drug discovery. In Current protocols in pharmacology unit 13A.3.1–13A.3.22, Wiley, New York, 2005 Search PubMed.
- Q. Yang, K. Olaifa, F. P. Andrew, P. A. Ajibade, O. M. Ajunwa and E. Marsili, Assessment of physiological and electrochemical effects of a repurposed zinc dithiocarbamate complex on Acinetobacter baumannii biofilms, Sci. Rep., 2022, 12, 11701 CrossRef CAS PubMed.
- M. Bernard, E. Jubeli, M. D. Pungente and N. Yagoubi, Biocompatibility of polymer-based biomaterials and medical devices – regulations, in vitro screening and risk-management, Biomater. Sci., 2018, 6, 2025–2053 RSC.
- C. Koski, N. Sarkar and S. Bose, Cytotoxic and osteogenic effects of crocin and bicarbonate from calcium phosphates for potential chemopreventative and anti-inflammatory applications In Vitro and In Vivo, J. Mater. Chem. B, 2020, 8, 2048–2062 RSC.
- T. M. Rawson, R. C. Wilson, D. O'Hare, P. Herrero, A. Kambugu, M. Lamorde, M. Ellington, P. Georgiou, A. Cass, W. W. Hope and A. H. Holmes, Optimizing antimicrobial use: challenges, advances and opportunities, Nat. Rev. Microbiol., 2021, 19, 747–758 CrossRef CAS PubMed.
- C. N. Banti and S. K. Hadjikakou, Evaluation of Genotoxicity by Micronucleus Assay in vitro and by Allium cepa Test in vivo, Bio-Protoc., 2019, 9, e3311 Search PubMed.
- A. Shokry, M. Khalil, H. Ibrahim, M. Soliman and S. Ebrahim, Acute toxicity assessment of polyaniline/Ag nanoparticles/graphene oxide quantum dots on Cypridopsis vidua and Artemia salina, Sci. Rep., 2021, 11, 5336 CrossRef CAS PubMed.
- D. Gupta, D. Bhatia, V. Dave, V. Sutariya and S. V. Gupta, Salts of Therapeutic Agents: Chemical, Physicochemical, and Biological Considerations, Molecules, 2018, 23, 1719 CrossRef PubMed.
- M. Löscher, C. Seiz, J. Hurst and S. Schnichels, Topical Drug Delivery to the Posterior Segment of the Eye, Pharmaceutics, 2022, 14, 134 CrossRef PubMed.
-
Bruker Analytical X-ray Systems, Inc., Apex2, Version 2 User Manual, M86-E01078, Madison WI, 2006 Search PubMed.
-
Siemens Industrial Automation, Inc., SADABS: Area–Detector Absorption Correction, Madison, WI, 1996 Search PubMed.
- L. Palatinus and G. Chapuis, SUPERFLIP – a computer program for the solution of crystal structures by charge flipping in arbitrary dimensions, J. Appl. Cryst., 2007, 40, 786–790 CrossRef CAS.
|
This journal is © The Royal Society of Chemistry 2024 |
Click here to see how this site uses Cookies. View our privacy policy here.