Estimating the bioaccessibility of atmospheric trace elements within the Athabasca bituminous sands region using the acid soluble ash fraction of Sphagnum moss†
Received
16th May 2023
, Accepted 21st February 2024
First published on 22nd February 2024
Abstract
Airborne trace elements (TEs) from the development of the Athabasca bituminous sands (ABS) in northern Alberta, occur in coarse and fine aerosols. Here, TEs in Sphagnum moss and acid soluble ash (ASA, obtained by leaching ash for 15 min using 2% HNO3) are used to estimate the distribution of TEs between these two aerosol fractions. Total concentrations of all elements increase toward industry, but chemical reactivity of the ash varies. Most of the Al is acid soluble, but most of the Th is not; the former is assumed to reflect the abundance and reactivity of light minerals, and the latter is a surrogate for heavy minerals. In the ASA, the trends in Ni and V, the dominant metals in bitumen, resemble Al. In contrast, Mo (also enriched in bitumen), plus Pb, Sb and Tl, are more like Th in exhibiting limited reactivity. Trace element enrichments in both the total and ASA fractions, relative to crustal abundance, are restricted to plant micronutrients (e.g., Cu, Mn, Mo, Zn), or elements that are passively taken up by plants (e.g., Cd and Rb, but apparently also Ag and Re). The greatest enrichments of TEs occur at the reference site, even though it is located 264 km from the centre of industrial activities. The ash of moss collected nearest industry is dominated by quartz (67%) which explains the low concentrations of TEs, absence of enrichment relative to crustal abundance, and limited chemical reactivity of Pb, Sb and Tl. In this region, total concentrations of TEs in moss are a poor guide to their bioaccessibility in the environment.
Environmental significance
Open pit bitumen mines and upgraders in northern Alberta generate large quantities of dust. Total concentrations of trace elements in Sphagnum moss increase with proximity toward industry, but their chemical reactivity is highly variable. In the ash fraction of the plants, most of the Al (surrogate for light minerals) is soluble in 2% HNO3 whereas Th (indicator of heavy minerals) is not. Nickel and V are similar to Al, whereas Pb, Sb and Tl follow Th. The abundance of quartz and other resistant minerals in plant ash explains the low concentrations and limited chemical reactivity of the dusts. Total concentrations of trace elements emitted to the atmosphere from bitumen mining and upgrading are a poor guide to their bioaccessibility.
|
1. Introduction
Upgrading and refining of hydrocarbons extracted from the Athabasca bituminous sands (ABS) in Alberta, Canada has remarkably propelled the economy of the province. Capital investment in oil production has accelerated at a considerable rate from more than $1 billion in 1996, to $16 billion per year from 2006 to 2008.1 Direct employment of the industry in the Fort McMurray area alone doubled between 1998 and 2008 from 6000 to 12
000.1 Total bitumen production was 2981 thousand barrels per day (bbl per d) in 2020, and is forecasted to increase to 4039 thousand bbl per d by 2030.2 However, with the increasing extent of the industry, environmental concerns regarding trace element (TE) contamination of the surrounding ecosystems are growing.3,4 Mechanical processing of ABS ores such as open-pit mining, quarrying, and road construction have yielded considerable amounts of dusts that are transported to the surrounding areas,5–14 especially within a 50 km radius of the mid-point between the two central bitumen upgraders.15 While the mid-point between the two central upgraders has traditionally been used as a point of reference for upgrader emissions, it continues to be helpful for characterizing other environmental impacts related to industrial development in the area.
Dusts from anthropogenic activities fall generally into one of two classes: coarse and fine aerosols.16–18 Coarse aerosols are usually generated during open pit mining activities by mechanical processes such as road construction, blasting operations, rock excavation and crushing activities: these are comparatively large (mainly 10–100 μm) and hence non-respirable.6,7,19 Given their size and density, they are typically removed from the air by sedimentation, giving them short atmospheric residence times (minutes to hours) and limited transport distances.16,18,20–24 These coarse aerosols are primarily in the forms of silicates and aluminosilicates which are enriched in elements such as Al, Ti, Sc, and Y25i.e. the conservative, lithophile elements which become residually enriched in soils during chemical weathering.26 Regarding the ABS, on average, they are approximately 85% mineral matter, predominantly dune and beach aeolian sands,27 with most particles >100 μm.28 The mineral composition is dominated by light minerals (99%), mainly quartz (60–90%) and phyllosilicates (kaolinite and illite), with minor amounts of feldspars (including orthoclase), mica (biotite and muscovite), carbonates (calcite, dolomite, and siderite), and chalcedony.29 Heavy minerals (density >2.96 g cm−3) account for 1% of the mineral fraction, and include ilmenite, tourmaline and zircon.29 Of this list of minerals, only the carbonate minerals are considered acid soluble in soil-derived, coarse aerosols.30
In contrast, fine aerosols are generated by high temperature processes such as the combustion of coal and other fossil fuels, smelting of metallic ores, cement production and waste incineration.8,31–34 The boundary between fine and coarse aerosols is commonly taken as 2 μm.35 Fine aerosols are typically generated by gas-to-particle conversion reactions, occur mainly in the size range of 0.1–1 μm, and are respirable.16,18,19,31,36–38 Due to their small size, they remain suspended in the air until they are removed by wet or dry deposition.18,22,24,31,38 With long atmospheric residence times (as long as a week), fine aerosols are easily transported for thousands of kilometers.23,31,37,38 These fine aerosols include acid soluble metal oxides and hydroxides and can be very rich in chalcophile elements such as Pb and Cd.36,37,39 Due to their small size and occurrence in acid soluble forms, the bioaccessibility of TEs is far greater in the fine aerosol fraction.36,40–43 When potentially toxic metals such as Pb are released to the environment in these forms, their enhanced bioaccessibility represents a threat to the health of many living organisms.19,32,44,45 Hence, to gain a better understanding of the associated human and ecosystem health risks of open pit bitumen mining and upgrading, it is important to distinguish between TEs in the coarse versus fine aerosol fractions.
Sphagnum mosses growing in ombrotrophic (rain-fed) bogs are excellent biomonitors of atmospheric deposition.46,47 They receive water and nutrients solely from the atmosphere, and exhibit remarkable capacities to retain atmospheric particles and metal ions.46,47 While there are many open questions about the mechanisms of retention, we know that physical interceptions of particles is made possible by the large surface area of moss leaves.47,48 Moreover, Sphagnum mosses have no cuticle:47,49 it is this hydrophobic layer, occurring on the leaves of higher plants, which minimizes particle adsorption and promotes particle removal by water.50 Further, Sphagnum moss is porous, which promotes the entrapment and retention of atmospheric particles: while the leaves lack stomata, the hyaline cells which constitute the bulk of the leaf structure, contain pores up to approximately 20 μm in diameter, to allow the passage of water.51–53 The leaves of Sphagnum moss are also ideally suited for the retention of metal ions: they have large cation exchange capacities:53–55 these are mainly attributed to the carboxyl groups of galacturonic acid which can account for up to 30% of the dry weight of the plants.49,56 These functional groups allow Sphagnum to retain ionic forms of essential metals such as K, Mg and Ca, micronutrients such as Ni, Cu and Zn, and potentially toxic metal ions such as Cd2+ and Pb2+.48,57 Finally, Sphagna are more resistant to higher levels of potentially toxic TEs than vascular plants,47,58 providing them with an additional advantage in polluted regions. Strong, positive, linear correlations have been observed between element concentrations in moss and independently measured atmospheric deposition rates, with results comparable to those obtained by conventional precipitation collection analysis (ref. 47,59 and references cited therein). For all of these reasons, environmental biomonitoring using mosses has proven to be an efficient, useful, and economical approach for assessing the spatial and temporal variations in atmospheric deposition of dusts and TEs worldwide.60–76
Previous studies found that total concentrations of Ag, Cd, Mo, Ni, Pb, Sb, V, and Tl in Sphagnum within the ABS region were similar to or lower than those in the “cleanest” ancient peat samples from Switzerland.14 Although their concentrations increased with distance toward the ABS region, these TEs were strongly correlated with conservative, lithophile elements which implicated mineral dust particles as the predominant source.14 Dust deposition rates within the ABS region were later quantified by distinguishing the acid soluble (ASA) and acid insoluble ash (AIA) fractions of Sphagnum.76 Mass accumulation rates of ash (6.0–27 g m−2 per year), AIA (1.0–12 g m−2 per year), and acid soluble concentrations of individual elements (Ca, Fe, K, Mg, P, and S) all showed generally increasing values toward industry.76 However, in that study, no measurements were made of any TEs in the ASA fraction. Given the ongoing concerns regarding potentially toxic TEs in the ABS region,77,78 we estimate the chemical reactivity of these dusts by determining the abundance of TEs in the ASA fraction. The ASA fraction is analyzed after filtering the leached ash through a 0.45 μm membrane filter.76,79 Thus, TEs in the ASA fraction of Sphagnum can be used as a surrogate for the fine aerosol fraction, with analyses of bulk moss representing the contributions of TEs from both the coarse and fine aerosols. Comparing the results obtained from these two fractions provides a first assessment of the chemical reactivity of the dusts emitted from bitumen mining and upgrading. The chemical reactivity thus determined, provides an estimate of the bioaccessibility of the TEs of interest, with implications for the health of surrounding ecosystems. Given the strong, positive, linear correlations between total concentrations of potentially toxic TEs in Sphagnum moss from this region, and conservative, lithophile elements such as Th14 as well as Sc, Th, Ti and Zr,80 we hypothesize that the chemical reactivity of these dusts is low in regard to TEs of concern such as Pb.
2. Materials and methods
2.1 Sample collection
The Sphagnum mosses used in this study were collected in the fall of 2015, 2019, and 2020. In September of 2015, Sphagnum moss was collected from 30 bogs within the ABS region, with moss from Utikuma (UTK), Birch Mountains Wildland (BMW), and Caribou Mountains Wildland (CMW) chosen as reference sites, as described by Mullan-Boudreau et al.76 (Fig. S1†). In October of 2019 and 2020, Sphagnum moss was collected in the ABS region again, from JPH4, McKay (McK), McMurray (McM), and Anzac (ANZ): these are listed in the order of increasing distance from the midpoint of two upgraders (Fig. S1†). Specifically, the four bogs are 12, 25, 49, and 68 km away from the mid-point between the two central bitumen upgraders. Utikuma (UTK), the reference site which is 264 km SW from this mid-point between the two central bitumen upgraders, was again included as a reference site during the 2019 and 2020 sampling campaigns. For comparison and context, samples were also collected from the Wagner Natural Area (WAG) and Elk Island National Park (EINP) which are both near the city of Edmonton.
At each location, samples were collected in triplicate from Sphagnum hummocks within relatively open areas, with individual samples taken approximately three meters apart. They were extracted by placing polypropylene (PP) boxes up-side-down on the hummocks, pressing down to have moss fill the container, and then cutting along the edges of the container with a medical-grade stainless steel knife. The mosses were then cut at the base of the box and sealed with the PP lid. Hair nets and polyethylene (PE) gloves were worn during collection. The PP boxes had been washed in the lab using soap and water, rinsed with tap water, and then three times with deionized water before departure to the field. Upon arrival in the lab, the samples were stored at −20 °C to prevent any changes before sample treatment. In total, 65 samples were used to determine total concentrations of TEs, and 69 samples for TEs in the ASA fraction.
2.2 Analytical methods
2.2.1 Sample preparation.
In the lab, the wet Sphagnum moss samples were cleaned of all dead and foreign plant materials using surgical stainless-steel tweezers while wearing PE gloves and a hair net (Fig. S2†). The cleaned samples were placed in PP jars and dried at 105 °C in a stainless-steel drying oven for two to three days until constant weight.
Subsamples of dried moss were ground by hand using an agate mortar and pestle and reserved for analyses of the bulk plant material. The remainder of each dried sample was ashed at 550 °C for 16 hours in a muffle furnace (MLS Pyro High-Temperature Microwave Muffle Furnace, Leutkirch, Germany) to obtain the ash.
2.2.2 Acid digestion of powdered moss and acid leaching of moss ash.
Total concentrations of TEs were obtained by digesting approximately 200 mg of powdered moss in a high-pressure microwave autoclave (UltraCLAVE, MLS, Leutkirch, Germany) as described in detail by Shotyk et al.14 The concentrated nitric acid (HNO3) used (3 ml) had been purified twice by sub-boiling distillation in high purity quartz (Duopur, MLS, Leutkirch, Germany). The tetrafluoroboric acid (HBF4) was used (0.1 ml) as supplied (Certified CAS, Fisher Scientific). For quality control, two standard reference materials (SRMs) were also digested: 1515 Apple Leaves from the National Institute of Standards and Technology (NIST), and Moss-1343 from the Finnish Forest Research Institute (FFRI).
To obtain the ASA fraction of Sphagnum moss, the procedure described by Sapkota et al.79 and Mullan-Boudreau et al.76 was modified by substituting HNO3 for HCl in order to minimize interferences (by chloride) in the mass spectra obtained using quadrupole ICP-MS.81 One hundred to 150 mg of ash was leached with 2% HNO3 for 15 min at room temperature, then filtered through 0.45 μm polytetrafluoroethylene (PTFE) filter membranes inside acid-cleaned PP filter holders with silicone gaskets. The filters were cleaned with 15 ml of 2% HNO3 before filtering the extracts. Blanks were obtained by filtering aliquots of 2% HNO3. The filtrates containing ASA and the filter membranes containing the acid insoluble ash (AIA) were both collected (Fig. S2†).
2.2.3 Determination of major and trace elements.
Digests of bulk Sphagnum moss and the ASA fraction were analyzed for both major and trace elements. Analyses of the digests of moss powders represent total concentrations, and include elements originally in and on the plant samples derived from all sources, both natural and anthropogenic, including the coarse and fine aerosol fractions. Acid soluble constituents are those which are leached from plant ash: this includes TEs originally in moss from biological uptake (e.g. micronutrients such as Cu and Zn), as well as most of the fine aerosol fraction (i.e. the fraction capable of passing through the 0.45 μm membrane filter), plus any acid soluble components in the coarse aerosol fraction (e.g. carbonate or phosphate minerals). Major elements were determined in our Department at the Natural Resources Analytical Laboratory (NRAL) using a Thermo iCAP 6300 Duo ICP-OES, and TEs in the metal-free, ultraclean SWAMP lab using a Thermo iCAP Qc ICP-MS. For quality control, along with the two SRMs noted above, the following water SRMs were analyzed: NIST 1643f Trace Elements in Freshwater, SPS-SW2 Surface Water (Spectrapure Standards), and SLRS-6 River Water (National Research Council of Canada). For both the ICP-MS and ICP-OES results, the limits of detection (LOD), limits of quantification (LOQ), method detection limits (MDL), and recoveries of SRMs were calculated and summarized in Tables S1–S4.†
2.2.4 Analysis of particle size, morphology, and mineralogy.
Selected ash samples from JPH4 (2019 and 2020), UTK (2020), and P6, S3, S16, S20 (all 2015) were analyzed for particle size in the range of 0.4–2000 μm using laser diffraction with a laser particle size analyzer (Beckman Coulter LS 13320, Beckman Coulter) at NRAL. Ash samples from JPH4 and UTK (both from 2020) were also examined using X-ray diffraction (XRD) (Rigaku Ultima IV) in the Department of Earth Sciences at the University of Alberta.
2.3 Data analyses and visualization
All data analyses and visualization were conducted in R 4.1.1.82
Concentration maps were created using packages ggmap83 and ggplot2.84
Principal component analysis (PCA) was performed on selected variables to explore the relationships between samples and between variables using the prcomp function. To make the variables comparable, the data were scaled (i.e. standardized) by setting “scale = TRUE” in the R code. The PCA was visualized using the factoextra package.85
Normality of variables was tested using the Shapiro–Wilk's test. With most of the variables being non-normally distributed, Spearman rank-based correlation tests were performed to examine the relationships among variables at a significance level of 0.05 using the cor function and visualized using the ggcorrplot package.86
Simple linear regressions were conducted to explore the specific correlations between certain elements using the lm function and visualized with the ggplot2 package.
3. Results and discussion
3.1 General description of element concentrations
First, distributions (boxplots) and ranges (ratio of maximum to minimum concentration) of the element concentration data were obtained to gain a general idea of their variation. The concentrations of the TEs in both bulk moss (i.e. total concentrations) and the ASA fraction showed that Al, Fe, and Mn (105–106 and 104–106 μg kg−1 in terms of total and ASA concentration, respectively) were the three most abundant TEs at both the industrial and the reference sites (Fig. 1). In contrast, the potentially toxic TEs of greatest concern, in order of decreasing abundance (Pb, Sb, Cd, Tl and Ag), exhibited low to very low concentrations (Fig. 1). The median TE concentrations in the samples of bulk moss were almost all higher at the industrial sites than at the reference sites (Fig. 1). In contrast, in the ASA, some elements were more abundant at the reference sites: again, in order of decreasing abundance, these were Zn, Cu, Cd and Ag (Fig. 1). The greatest differences in total concentrations occur with the conservative lithophile elements such as Y and Th: industrial and reference sites differed by approximately 40× and 30× in 2015 and 2019 (Fig. S3†), and 20× in 2020 (Fig. S4†). At the other extreme, the smallest differences (1×–4×) were seen in micronutrients such as Zn and Cu, and macronutrients such as K, P and S (Fig. S3 and S4†). The fact that the greatest differences in total concentrations of TEs are seen with the conservative lithophile elements reflects the increases in dust deposition rates with proximity toward industry. Even in the ASA fraction, these elements were more abundant at industrial sites compared to reference sites (Fig. 1 and S4†).
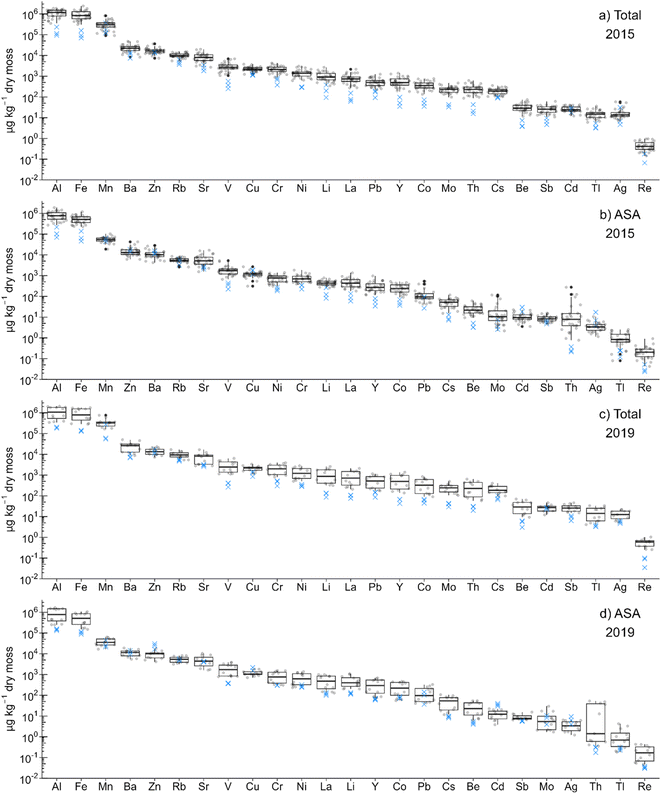 |
| Fig. 1 Boxplots of element concentrations for bulk moss (i.e. total) and acid soluble ash (ASA) at industrial sites. Element concentrations in samples from reference sites (BMW, CMW, and UTK for 2015, and UTK for 2019) are indicated in blue using the multiplication sign “×”. All values represent concentrations (μg kg−1) in dry moss. Samples were collected in fall 2015 (a and b) and 2019 (c and d). The 2015 EINP and WAG samples were excluded. | |
Conservative lithophile elements such as Al are abundant in the earth's silicate crust87 and occur either in minerals that are resistant to chemical weathering, or weather to form secondary minerals that are thermodynamically stable.26,80,87 Thus, the abundance of these elements in Sphagnum moss can be used as indicators of the amounts of mineral dust being deposited from the air, and can be used to distinguish the natural background values from anthropogenic inputs.88 In addition, Th and the rare earth elements, including Y and La, are abundant in heavy minerals such as zircon and monazite (density 4.6 to 5.4 g cm−3) which are particularly stable in weathering environments.80,89,90 Various heavy minerals are also found in the mineral fraction of the ABS29 which consists primarily of beach and dune sands.3,27,80,91 Strong positive correlations of lanthanides and Al with the mineral content in the ABS are well established.92 Elements such as Al, La, Th and Y are not essential to plants and therefore it can be assumed that there is no active uptake. Given the limited solubility of their host minerals, there is little potential for passive uptake of these elements from solution. Thus, the concentrations of these elements in Sphagnum moss can reasonably be taken to indicate the deposition of the insoluble mineral dusts onto the surface of the plants.
Aluminum is the most abundant metal in the Upper Continental Crust (UCC) at approximately 81
500 mg kg−1.87 In contrast, the abundances (mg kg−1) of the TEs of environmental concern are far lower i.e. Pb, 17; Tl, 0.9; Sb, 0.4; Cd, 0.09, and Ag, 0.05. For calculating element ratios, Y was selected as the reference element of choice (see Sections 3.2, 3.3, 3.4, and 3.8), as its abundance in the UCC (21 mg kg−1) is most similar to that of Pb, the element which has generated so much environmental concern.
3.2 Total concentrations of trace elements
3.2.1 Conservative, lithophile elements.
Spatial variations in total Y exhibited an increasing trend toward industry (Fig. 2) which resembles the distribution of ash content (Fig. S5†). The other conservative lithophile elements, such as Al, Th, La, and Cr, behaved similarly to Y (Fig. S6†). Total Fe also increased toward industry and showed a strong positive correlation with total Y (Spearman's r = 0.93, p < 0.05; Fig. S7 and S8†). Ferric iron is also a conservative lithophile element,90 and as such has been used as an indicator of soil-derived dusts in other studies.93,94 However, given that bog waters are naturally acidic, organic-rich, and generally anoxic,95 there is potential for Fe to be mobilized under these conditions. Despite this possibility, the correlation between Fe and Y in the bulk moss samples suggests that Fe concentrations too, reflect increases in dust deposition toward industry. While the mid-point between the two central bitumen upgraders is commonly viewed as the centre of industrial development, wind direction must also be considered in any analysis of dust distribution. For example, the predominant wind direction from May to August in 2015 was southwest, in addition to north and south (Fig. S9†): this may help explain the relatively high concentrations of Y seen at sites southeast of Fort McKay (Fig. 2) and sites such as S7, S9, S19, and P11 (Fig. 2 and S1†).
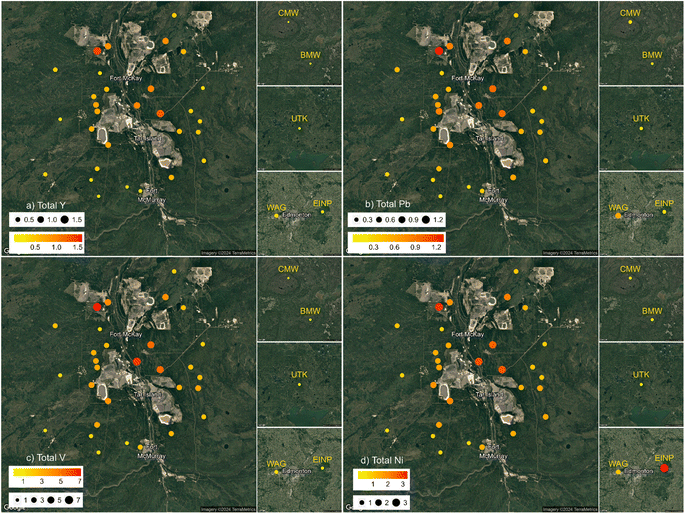 |
| Fig. 2 Spatial distribution of (a) Y, (b) Pb, (c) V, and (d) Ni concentrations in bulk Sphagnum moss collected in fall 2015. Concentrations are expressed on the basis of mg kg−1 in dry moss. CMW = Caribou Mountains Wildland, BMW = Birch Mountains Wildland, UTK = Utikuma, WAG = Wagner Natural Area, EINP = Elk Island National Park. | |
3.2.2 Elements enriched in bitumen.
The two most abundant metals in bitumen, namely V and Ni,96 strongly resembled Y in their distribution (Fig. 2). This was also reflected by their strong linear correlations with Y (R2 = 0.89; Fig. S10†), implying that mineral dust inputs also dictate the total concentrations of these two metals, an observation made in previous studies.10,74,95 In addition, the slopes of the regression lines of V (4.5) and Ni (2.0) versus Y (Fig. S10†) are similar to their corresponding ratios in the UCC (97/21 = 4.6 and 47/21 = 2.2, respectively)87 which further supports mineral dusts as the predominant sources of these metals in this region.3,14,80,97–100 Strong, positive, linear correlations between V and mineral matter contents of the ABS are well known.92
Molybdenum, the third most abundant metal in bitumen,96 also increased in concentration near industry, but did not correlate as strongly with total Y (R2 = 0.57; Fig. S7 and S10†) as V or Ni. There are several possible reasons why the variation in Mo is less dependent upon the amounts of mineral matter in the moss. First, Mo is used as a catalyst for upgrading bitumen in this area.101,102 Second, Mo differs from V in that Mo is an essential micronutrient for plants.103,104 Thus, Sphagnum moss may also be affected by throughfall, as precipitation leaches Mo from dead tissues of other plants growing in the vicinity of the moss.73,105–107 However, Ni is also an essential plant micronutrient,103 and there is no evidence to suggest that Ni is affected in this way. Molybdenum forms anionic species in soil solutions108,109 which render it more mobile than cationic TEs, but this is true also of V,110 and there is little evidence of V mobilization. Clearly, the variation in atmospheric Mo deposition in this area requires further study to understand its predominant sources and transformations in bogs.
3.2.3 Chalcophile elements.
The distribution of Pb followed that of Y (Fig. 2) and there is a very strong, positive, linear correlation (R2 = 0.95) between the two elements (Fig. S10†). The slope of the regression line of Pb plotted against Y (0.79) is very similar to the corresponding ratio in the UCC (17/21 = 0.81).87 Clearly, mineral dust is by far the dominant source of Pb to the moss samples. For perspective, total concentrations of Pb in the ABS region (0.23–1.3 mg kg−1) are low: these are comparable to the values reported for moss (0.65–1.1 mg kg−1) collected from a very remote Canadian bog near Great Slave Lake.69 Total concentrations of Sb and Tl also followed the distribution of Y (Fig. S11†) and were moderately or strongly correlated with Y (R2 = 0.76 and 0.89, respectively; Fig. S10†).
In contrast to Pb and Tl, which were strongly correlated with Y, Ag (R2 = 0.13) and Cd (R2 = 0.16) are independent of Y (Fig. S10 and S12†). Thus, it appears that aerial deposition of Ag and Cd is effectively independent of mineral dust inputs. Silver is in the same group as Cu in the periodic table, and Cd in the same group as Zn, giving Ag and Cd some physical and chemical properties similar to Cu and Zn, respectively.111–113 Copper and Zn are both essential to plants,103 whereas Ag and Cd are not. However, there is evidence of passive uptake of Ag by plants114,115 and a voluminous literature on active uptake of Cd.116–119 In fact, Cd uptake by plants has been found to be performed by transporters of essential elements such as Cu, Fe, Mn, and Zn.120,121 There are also published studies showing that some moss species were able to accumulate Ag and Cd.104,122 Thus, the total concentrations of Ag and Cd in the Sphagnum moss samples studied here, may have been affected by plant uptake.
3.2.4 Elements essential to plants.
Total Cu showed a slightly increasing trend toward industry and a moderately positive correlation with total Y (Fig. S8 and S13†). However, total Mn and Zn did not significantly correlate with Y, indicating that processes other than atmospheric dust inputs alone, dominate their distribution.74,80,94,99 We assume that the limited spatial variation in Mn and Zn concentrations is due to active plant uptake of both elements.
3.3 Trace elements in the acid soluble ash (ASA) fraction
Total and acid soluble concentrations of selected TEs are shown in Fig. 3. The range in behaviours exhibited by these elements (Al, Th, Ca, Mn, V, Ni, Mo, Tl, Pb and Sb) is representative of all the studied elements, and provides a concise description of main trends. In all cases, the results obtained from sampling in 2019 and 2020 were very consistent, indicating the reproducibility of the findings. Although the general trend is an increase in the acid soluble concentrations of the elements with distance toward industry (Fig. 3), the magnitude of the changes is dichotomous. For example, Al and Ca, along with V and Ni, clearly show increasing concentrations in the ASA fraction with distance toward industry. In contrast, Th, Mn, Mo, and Sb show much less change toward the mines and upgraders. For example, consider the two conservative lithophile elements shown here: acid soluble Al consistently accounts for the majority of total Al, while acid soluble Th represents only a small fraction of total Th. We assume that the Al concentrations in the moss samples are mainly contributed by light minerals such as those of the plagioclase group, and phyllosilicates (clays), whereas Th is assumed to be supplied from heavy minerals such as zircon which is extremely stable.
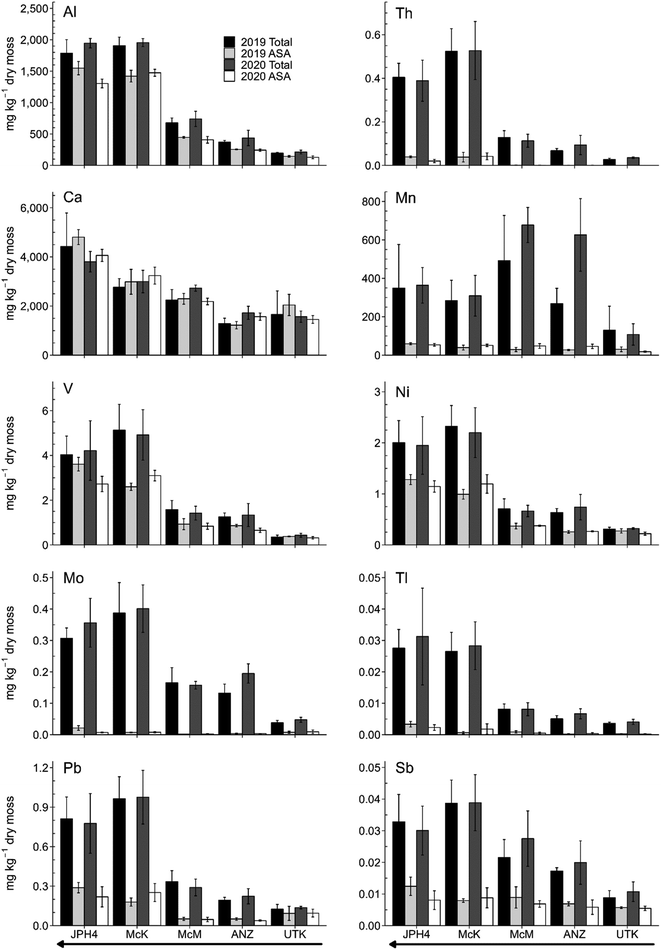 |
| Fig. 3 Total and acid soluble concentrations of Al, Th, Ca, Mn, V, Ni, Mo, Tl, Pb, Sb in Sphagnum moss collected in October 2019 and 2020. Concentrations are expressed on the basis of mg kg−1 in dry moss. ASA = acid soluble ash. McK = Fort Mckay, McM = Fort McMurray, ANZ = Anzac, UTK = Utikuma. The arrow points from the reference site (UTK) toward industry. Error bars represent ± standard deviation. | |
3.3.1 Significance of the “soluble” trace elements.
Calcium is by far the most reactive of the elements shown in Fig. 3. It is well known that carbonate minerals are the most reactive species found in the mineral fraction of the ABS, with calcite, dolomite and siderite all having been identified.29
Although Al is a conservative lithophile element, during chemical weathering it is converted from primary silicate minerals to secondary minerals, especially clays such as kaolinite.90 The mineral fraction of the ABS contains significant quantities of clays, ranging from 1 to 37.5 wt%,123 consisting mainly of kaolinite and illite.29,123,124 It is well known that some of the clays in the ABS occur as ultrafine particles as small as 20 nm.125 For convenience, ultrafine clay minerals are defined here as mineral particles able to pass through a 0.45 μm membrane filter.3 Given that silicate mineral dissolution rates increase with decreasing particle size,126 we assume that the clay minerals occurring in the ash of Sphagnum moss after ashing, would react very quickly in the 2% HNO3 solutions used here. Thus, the elevated concentrations of Al found in the ASA fraction of Sphagnum is assumed to reflect the dissolution of ultrafine clays. Other conservative lithophile elements (Y, Cr, La) behave similarly to Al (Fig. S14 and S15†) which reflects the broader significance of the phyllosilicates.
A large percentage of V and Ni is acid soluble (Fig. 3). While V and Ni are the most abundant metals in bitumen, the strong, positive, linear correlation exhibited by these metals with Y (Fig. S10 and S16†) argues against bitumen as the most important source of these metals. Most of the dust being generated is from the mines, gravel roads, and dry tailings. The dry tailings are essentially the mineral residues left behind after bitumen (and the V and Ni it contains) has been extracted:1,127 we assume that V and Ni are hosted by the clay minerals in the dry tailings. Given the small size of the clays contained in the tailings, they are easily suspended in the air by wind and then transported long distances. Whether or not the TEs associated with these clays will be released to the environment at ambient conditions (i.e. far less acidic than the reactant employed here) will depend on how much of the elements are in an exchangeable form, versus being incorporated within the silicate structures of these minerals.128 Similar considerations apply to other light minerals such as feldspars and mica.90,128 Thus, TEs found in the ASA fraction of Sphagnum moss is not necessarily an indicator of the risk they pose to living organisms. Certainly, the mineralogy and chemical reactivity of the ASA fraction requires further investigation.
The acid soluble concentrations of Ag and Cd and their acid soluble proportions were both greater at the reference site, UTK (Fig. S14 and S15†). The higher proportion of acid soluble Ag and Cd at UTK can be understood in regard to the limited amount of dust being supplied to this remote location. However, the greater concentrations of Ag and Cd found in the ASA at the reference site, is unclear.
3.3.2 Significance of the “insoluble” trace elements.
The dearth of Th in the ASA fraction of Sphagnum moss can reasonably be assumed to reflect its occurrence in heavy minerals, and their chemical stability. But it is much less obvious how to explain the limited chemical reactivity of Mn, Mo, Pb, Sb and Tl (Fig. 3). Both Mn and Mo are essential to plants.103 However, even if they were to occur exclusively in organic forms within the living plants, these should then be found in the ASA fraction, along with the major elements K, Mg, Ca, P and S.129,130 Thus, there is no obvious explanation for the low proportion of Mn and Mo in the ASA. Further, given that the mineral dusts being released from the mines, gravel roads, and dry tailings must contain both elements, the lack of increase in concentration within the ASA fraction (Fig. 3), with proximity to industry, is puzzling. Similarly, the potentially toxic TEs, Pb, Sb, and Tl, showed limited reactivity (Fig. 3). Of these three, Tl had the lowest proportion of acid soluble metal. The lack of reactivity of particles containing Pb, Sb and Tl in 2% HNO3 may be related to the size of the host phases, or their limited solubility. Regardless of the reason, even less reactivity is expected at the ambient pH of the soils and waters of the region.
3.4 Enrichments of trace elements
The enrichments of TEs were calculated by dividing the TE concentrations in the ash fraction by their corresponding crustal abundance in the UCC.87,95 The calculations were performed for both the total and acid soluble concentrations of TEs, using samples collected from industrial and reference sites. Taking into account natural variation, only enrichments greater than a factor of 2 times are considered significant.95 Regarding the total concentrations, very few elements were significantly enriched: Cu, Mn, Mo, and Zn (all are plant micronutrients), as well as Ag, Cd, Rb, and Re (Fig. 4). Although this latter group is not required by plants, they can be seen as pairs that share some chemical properties with plant micronutrients e.g., Cd and Zn,111,112 Rb and K,131 Re and Mo.132 Specifically, the predominance area diagrams and solubility diagrams for Cd and Zn are very similar;128,133 this is true also of Re and Mo.134 In regard to Rb and K, their ions have the same electrical charge and similar ionic radii.135 Regarding the enrichment calculations, the lack of significant enrichment of potentially toxic TEs (i.e. the elements of greatest concern, namely Pb, Sb, Tl), is notable.
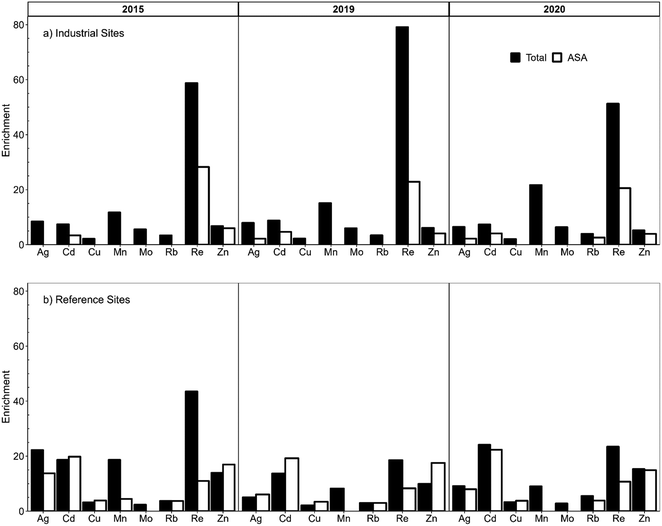 |
| Fig. 4 Enrichments of trace elements in Sphagnum moss and acid soluble ash (ASA), relative to the Upper Continental Crust.87 Samples were collected from (a) industrial sites and (b) reference sites (average of BMW, CWM, and UTK for 2015, and UTK for 2019 and 2020). Concentrations in the ASA fraction are expressed on the basis of their abundance in the ash (mg kg−1 ash). Total concentrations in dry moss are normalized to 100% ash content and expressed as mg kg−1 in the ash.95 2015 EINP & WAG were excluded. Only enrichments greater than 2× are presented. | |
Regarding TE enrichments in the acid soluble fraction, the results were similar (Fig. 4). Moreover, the enrichments of two potentially toxic elements, Ag and Cd, were greater at the reference sites compared to the industrial sites. Given the long list of TEs investigated here, it is rather remarkable how few elements exhibit significant enrichments in the Sphagnum moss collected near industrial development, relative to the corresponding crustal values. The results obtained from samples collected in 2019 and 2020 are very consistent, illustrating the reproducibility of the geochemical data and the approach used to study atmospheric deposition of TEs.
3.5 Abundance and particle size of minerals in ash from proximal and distal locations
X-Ray diffraction (XRD) analyses were used to identify the minerals present in ash samples of moss collected from JPH4 (the site closest to industry) and UTK (the main reference site). Quartz was the predominant mineral in moss from JPH4, followed by hydroxyapatite, arcanite, microcline, muscovite, and CaO (Fig. 5 and S17†). The abundance of quartz (36.7%) in the ash of moss from JPH4 was approximately 6 times that of the moss from UTK (6.1%). At UTK, the minerals identified, in decreasing order of abundance, were fairchildite, arcanite, hydroxyapatite, albite, diopside, quartz, and halite (Fig. 5 and S17†). Among these minerals, hydroxyapatite, arcanite, CaO, fairchildite, and halite are artefacts that were created during ashing.136,137 Correcting the mineral abundances for those created during ashing, quartz accounted for 66.7% of JPH4 ash, but only 15.5% at UTK. The corrected value obtained for quartz at JPH4 is within the range reported (60–90%) for quartz in the mineral fraction of the ABS.29 It is well known that quartz is a very poor host for TEs, and most TEs are rare in quartz.3,92,138 The preponderance of quartz grains in the ash fraction of Sphagnum moss collected near industry explains why their ash contents are so high while, at the same time, their TE concentrations are so low.
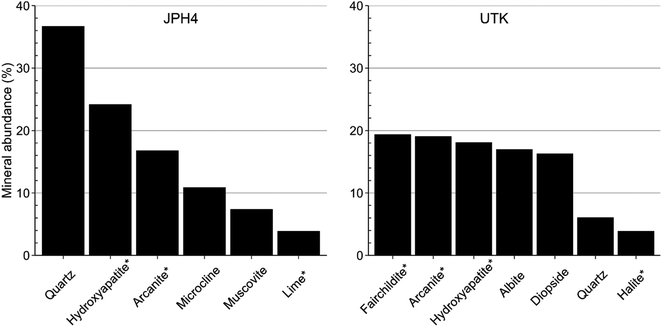 |
| Fig. 5 Mineral abundance (%) from XRD analyses of Sphagnum moss ash samples collected from JPH4 and UTK in October 2020. UTK = Utikuma. The asterisks (*) indicate minerals created during ashing. Note: Ignoring the minerals created during ashing, quartz accounts for 66.7% of the ash at JPH4 and 15.5% of the ash at UTK. | |
Particle size analyses showed increasing numbers of large particles on Sphagnum with distance toward industry, with diameters typically ranging from 10 to 100 μm, and some particles as large as 200 μm (Fig. S18†). Given the size of these particles and the density of minerals such as quartz, these coarse dusts will be removed from the air by sedimentation, giving them short atmospheric residence times, and limited transport distances.16,22
3.6 Principal component analysis
The results of the PCA were consistent with the observations described above. The PCA for the total concentrations of TEs in the 2015 mosses showed that principal component (PC) 1 explained 60.0% of the variation in the dataset (Fig. 6a). The greatest contributors to this PC were the conservative lithophile elements (Y, La, Th, Cr, Al), potentially toxic elements (Pb, Tl, Sb), elements enriched in bitumen (V, Ni, Mo), some other lithophile elements such as Be, Li, and Fe, and the ash content (Fig. S19†). PC 1 is clearly related to the abundance of mineral matter, and separated the reference sites (BMW, CMW, and UTK) from the industrial sites. PC 2 explained 12.3% of the variation in the dataset, which was primarily contributed by plant macro- and micronutrients such as P, Mg, K, Zn, Mn, Ca, and S.
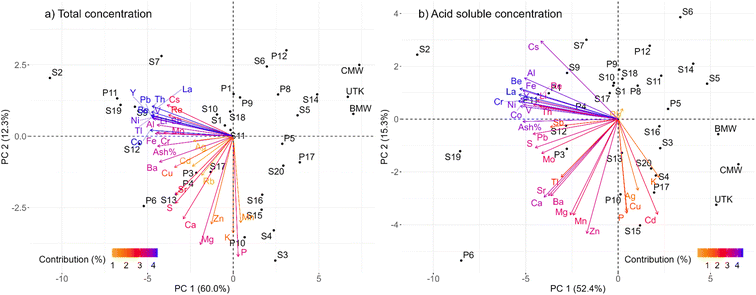 |
| Fig. 6 Principal component analysis of 2015 Sphagnum mosses using (a) total concentrations and ash content and (b) acid soluble concentrations and ash content. The contribution reflects the total contribution of the variables to principal component (PC) 1 and 2. BMW = Birch Mountains Wildland, CMW = Caribou Mountains Wildland, UTK = Utikuma. | |
The PCA for acid soluble concentrations of the 2015 mosses showed that PC 1 and 2 explained 52.4 and 15.3% of the variations, respectively (Fig. 6b). Again, conservative lithophile elements (Cr, La, Y and Al), elements enriched in bitumen (Ni and V), and some other elements (Be, Co, Fe) contributed the most to PC 1 (Fig. S19†). These elements are more abundant in samples collected near industry (e.g., S2, S19, and P6) compared to the reference sites (BMW, CMW, and UTK). Plant macro-and micronutrients including Zn, Mn, Mg, P, Cu, and K (in order of decreasing importance), contributed the most to PC 2 but little to PC 1 (Fig. S19†), suggesting that their abundance in this fraction is relatively unaffected by dust emissions. Included in PC 2 of the ASA fraction are Cd, Cs, Ba, Sr, Ag and Tl (Fig. S19†): none of these are required by plants,103 but their inclusion with PC 2 may be a consequence of passive uptake by Sphagnum.
3.7 Temporal trends in element accumulation
The ash contents of the Sphagnum moss samples collected in 2019 and 2020 as well as the total concentrations of TEs showed limited variation between the two years (Fig. S20†). The acid soluble concentrations were also comparable between the two years (Fig. S21†). Sphagnum mosses were collected from the same five sites in October of both years and only the living layer (i.e. top 2 cm of the plants) were processed each time. In all cases, therefore, the samples collected reflect summertime bulk deposition i.e. wet deposition, plus dry deposition of atmospheric dusts and aerosols. Assuming similar rates of Sphagnum growth, the comparable concentrations indicate that dust and aerosol deposition from bitumen mining and upgrading did not vary significantly between the two years. Further, the mosses from 2015 were collected from sites different from those from 2019 and 2020, and yet they all yielded similar concentrations. Taken together, the reproducibility of the findings suggests that the Sphagnum moss samples have provided a consistent record of atmospheric deposition of dust and aerosol particles and their associated TEs.
3.8 Deposition of dusts and aerosols in other locations within Alberta
3.8.1 Elk Island National Park.
Moss collected at EINP was high in both total Ni (3.3 mg kg−1) and acid soluble Ni (2.1 mg kg−1) (Fig. 2 and S22†). Total Ni is disproportionate to total Y (Fig. S10†) and the enrichment factor (EF) for Ni (8.3) was the highest among all sites (Fig. S23†). Clearly, there were additional sources of Ni to these moss samples other than windblown soil dust.14,80,97 The obvious source of excess Ni in these samples is the nickel refinery in Fort Saskatchewan, Alberta which is only approximately 26 km northwest of EINP. So, even though Ni is enriched in the bitumen deposits being mined on a vast scale in northern Alberta,96 the moss samples most enriched in this metal occur in central Alberta, because of nickel refining.
3.8.2 Wagner Natural Area.
The moss samples collected at WAG were high in total Sb (63 μg kg−1) and acid soluble Sb (30 μg kg−1) (Fig. S11†). These samples represent an outlier in the linear regression of Sb with total Y (Fig. S10†) and yielded the greatest EF value (9.5) among the sites investigated (Fig. S23†). The elevated concentrations of Sb at WAG could be ascribed to road traffic.139 Antimony sulphide (Sb2S3) has been used in brake linings since the late 1990s, having replaced asbestos, and has become a significant source of atmospheric Sb, especially in urban areas.105,140,141 The particles generated by brake abrasion are respirable, with an aerodynamic diameter (common mass median) of 2.8 μm, and particle numbers increase with increasing temperature.141,142 The high temperatures generated during braking promote the oxidation of Sb2S3 to the more easily dissolved antimony trioxide,143 making it more bioaccessible. Vehicle fuel combustion is also a source of anthropogenic Sb.105,140 In aerosols collected from urban areas of Europe, EF values for Sb >50 are common,105 but EF values in the hundreds and in the thousands have also been reported.136 From this perspective, the EF values for WAG reported here, are remarkably low: in fact, they are only twice the pre-anthropogenic values obtained from peat samples dating from the mid-Holocene.136
3.9 Trace elements in acid soluble and acid insoluble ash: Quo vadis?
The comparison of TEs in ASA with total concentrations in Sphagnum moss has provided new insight into the contrasting behaviours and potential bioaccessibility of these elements in the environment (Fig. 3). For context, the determination of AIA in biological materials has a long history, having been used for many decades as an index of the digestibility of animal feeds (ref. 144 and references cited therein). This parameter, determined by combustion and acid leaching in HCl, continues to be measured today,145 not only for these applications, but also to quantify inorganic contaminants in medicinal plants.146 The AIA content of peat was first determined in a bog profile from Finland, to determine the accumulation rate of organic matter.147 Later, AIA was used for mineralogical studies of atmospheric dust particles in peat;148 in both cases, the insoluble residue was collected on filter paper. A subsequent study of AIA in peat involved filtration using a 0.2 μm membrane filter, but again, the focus was chemical and mineralogical analyses of the insoluble fraction:79 the soluble components were not analyzed. It was only recently that the ASA fraction of Sphagnum moss from bogs was analyzed, but as noted earlier, only for major elements.76 In the latter case, as well as in the present investigation, a 0.45 μm membrane filter was used to obtain the ASA fraction. Given that fine aerosols are defined as particles smaller than 2 μm,35 the analytical procedure that we have used here may have underestimated the concentrations of TEs in the fine aerosol fraction. With the commercial availability of PTFE membrane filters with nominal pore sizes of 0.2 μm, 0.45 μm, and 2 μm, additional studies of TEs in these size fractions of ASA is warranted. Although Pb is not lost upon ashing of peat at 550 °C,149 the possible importance of losses of other TEs upon combustion should be investigated. Finally, the surfaces of moss leaves have been found to preferentially retain coarse particles compared to fine aerosols.150 Given this circumstance, an intercomparison is warranted, to compare the concentrations of TEs in ASA from Sphagnum moss with ASA obtained from aerosols collected directly using conventional aerosol sampling devices installed at the same location.
4. Conclusions
Mineral dust deposition to bogs in the region, reflected by the ash content of Sphagnum moss, increases with proximity toward industry. The total concentrations of elements enriched in bitumen (V, Ni, Mo) and potentially toxic elements (Pb, Sb, Tl) also increase toward industry, resembling the trends in conservative lithophile elements (Al, Cr, La, Th, Y). These trends reflect the importance of mineral dust deposition and its contribution to total concentrations of most TEs. However, the chemical reactivity of TEs in these mineral dusts varies. Acid soluble concentrations of V and Ni clearly increase with distance toward industry, resembling Al in the same fraction which suggests clay minerals such as kaolinite and illite may be important sources. In contrast, the proportions of Pb, Sb, and Tl in the acid soluble fraction are much lower, resembling the trend in Th and inferring important contributions from more stable, acid-resistant minerals. Trace elements that are enriched in both total concentration and the ASA fractions, relative to their crustal abundances, are either plant micronutrients such as Cu, Mn, Mo, Zn, or elements that resemble them in behaviour such as Ag, Cd, Rb, and Re, with plants from the reference site exhibiting the greatest enrichments. The study highlights the importance and necessity to determine not only the total concentration but also the chemical reactivity of TEs in atmospheric dusts when evaluating their associated health risks to living organisms.
Author contributions
N. Chen: 2019 and 2020 field work, lab work, formal analysis, data curation, statistical analysis, visualization, writing original draft and revising. F. Barraza: supervision, 2020 field work, 2019 and 2020 ICP-MS analysis, data validation, manuscript review and editing. R. J. Belland: supervision, manuscript review and editing. M. B. Javed: supervision, manuscript review and editing. I. Grant-Weaver: 2015 sample preparation and ICP-MS analysis, manuscript review and editing. C. W. Cuss: supervision, methodology, manuscript review and editing. W. Shotyk: conceptualization, methodology, 2015 fieldwork, supervision, manuscript review and editing, project administration, funding acquisition.
Conflicts of interest
The authors have no conflicts of interest to declare.
Acknowledgements
Sincere thanks to the Natural Sciences and Engineering Research Council (NSERC) of Canada (CRD 531329) and Canada's Oil Sands Innovation Alliance (COSIA; WE 0057) for their financial support. Special thanks to Dr Mandy Krebs, Mika Little-Devito, Jinping Xue, and Yu Wang for their helpful comments, to Lukas Frost for leading the Metals vs. Minerals field trips, and Dr Jaqueline Dennett, Ron Goyhman, and Andrii Oleksandrenko for helping with the 2019/2020 fieldwork, and to Dr Mark Donner, Tommy Noernberg, and Gillian Mullan-Boudreau for 2015 sample collection. Thanks to Tracy Gartner for administrative support, Karen Lund for creating the TOC, and Rick Pelletier for helping with the map of sampling locations. This manuscript was considerably improved thanks to the constructive comments of three helpful reviewers.
References
-
P. Gosselin, S. Hrudey, M. Naeth, A. Plourde, R. Therrien, G. Van Der Kraak and Z. Xu, Environmental and Health Impacts of Canada's Oil Sands Industry, Royal Society of Canada, Ottawa, Ontario, 2010, https://rsc-src.ca/sites/default/files/RSCOilSandsPanelMainReportOct2012.pdf Search PubMed.
-
Alberta Energy Regulator, Crude Bitumen Production, https://www.aer.ca/providing-information/data-and-reports/statistical-reports/st98/crude-bitumen/production Search PubMed.
- W. Shotyk, B. Bicalho, C. W. Cuss, M. Donner, I. Grant-Weaver, M. B. Javed and T. Noernberg, Trace elements in the Athabasca Bituminous Sands: A geochemical explanation for the paucity of environmental contamination by chalcophile elements, Chem. Geol., 2021, 581, 120392 CrossRef CAS.
- C. A. Cooke, J. L. Kirk, D. C. G. Muir, J. A. Wiklund, X. Wang, A. Gleason and M. S. Evans, Spatial and temporal patterns in trace element deposition to lakes in the Athabasca oil sands region (Alberta, Canada), Environ. Res. Lett., 2017, 12, 124001 CrossRef.
- C. Phillips-Smith, C.-H. Jeong, R. M. Healy, E. Dabek-Zlotorzynska, V. Celo, J. R. Brook and G. Evans, Sources of particulate matter components in the Athabasca oil sands region: Investigation through a comparison of trace element measurement methodologies, Atmos. Chem. Phys., 2017, 17, 9435–9449 CrossRef CAS.
-
J. G. Watson, J. C. Chow, X. Wang and S. D. Kohl, Windblown fugitive dust characterization in the Athabasca Oil Sands Region. WBEA-DRI Agreement Number: T108-13, 2014, https://wbea.org/wp-content/uploads/2018/03/watson_j_g_et_al_2014_windblown_fugitive_dust_characterization_in_the_athabasca_oil_sands_region.pdf.
- X. Wang, J. C. Chow, S. D. Kohl, L. N. R. Yatavelli, K. E. Percy, A. H. Legge and J. G. Watson, Wind erosion potential for fugitive dust sources in the Athabasca Oil Sands Region, Aeolian Res., 2015, 18, 121–134 CrossRef.
- Z. Xing and K. Du, Particulate matter emissions over the oil sands regions in Alberta, Canada, Environ. Rev., 2017, 25, 432–443 CrossRef CAS.
-
J. R. Graney, M. S. Landis and S. Krupa, in Alberta Oil Sands, ed. K. E. Percy, Elsevier, 2012, pp. 343–372 Search PubMed.
-
M. S. Landis, J. P. Pancras, J. R. Graney, R. K. Stevens, K. E. Percy and S. Krupa, in Alberta Oil Sands, ed. K. E. Percy, Elsevier, 2012, pp. 427–467 Search PubMed.
- M. S. Landis, J. Patrick Pancras, J. R. Graney, E. M. White, E. S. Edgerton, A. Legge and K. E. Percy, Source apportionment of ambient fine and coarse particulate matter at the Fort McKay community site, in the Athabasca Oil Sands Region, Alberta, Canada, Sci. Total Environ., 2017, 584–585, 105–117 CrossRef CAS PubMed.
- M. S. Landis, W. B. Studabaker, J. Patrick Pancras, J. R. Graney, K. Puckett, E. M. White and E. S. Edgerton, Source apportionment of an epiphytic lichen biomonitor to elucidate the sources and spatial distribution of polycyclic aromatic hydrocarbons in the Athabasca Oil Sands Region, Alberta, Canada, Sci. Total Environ., 2019, 654, 1241–1257 CrossRef CAS PubMed.
- S. M. Jordaan, Land and water impacts of oil sands production in Alberta, Environ. Sci. Technol., 2012, 46, 3611–3617 CrossRef CAS PubMed.
- W. Shotyk, R. Belland, J. Duke, H. Kempter, M. Krachler, T. Noernberg, R. Pelletier, M. A. Vile, K. Wieder, C. Zaccone and S. Zhang,
Sphagnum mosses from 21 ombrotrophic bogs in the Athabasca Bituminous Sands region show no significant atmospheric contamination of “heavy metals”, Environ. Sci. Technol., 2014, 48, 12603–12611 CrossRef CAS PubMed.
-
E. S. Edgerton, J. M. Fort, K. Baumann, J. R. Graney, M. S. Landis, S. Berryman and S. Krupa, in Developments in Environmental Science: Alberta Oil Sands, ed. K. E. Percy, Elsevier, 2012, pp. 315–342 Search PubMed.
- K. Willeke and K. T. Whitby, Atmospheric aerosols: Size distribution interpretation, J. Air Pollut. Control Assoc., 1975, 25, 529–534 CrossRef.
- K. T. Whitby, R. Husar and B. Liu, The aerosol size distribution of Los Angeles smog, J. Colloid Interface Sci., 1972, 39, 177–204 CrossRef CAS.
-
S. Ramachandran, Atmospheric Aerosols: Characteristics and Radiative Effects, CRC Press, Boca Raton, FL, 2018 Search PubMed.
- D. L. Swift and D. F. Proctor, Human respiratory deposition of particles during oronasal breathing, Atmos. Environ., 1982, 16, 2279–2282 CrossRef.
-
L. Schuetz, in Paleoclimatology and Paleometeorology: Modern and Past Patterns of Global Atmospheric Transport, ed. M. Leinen and M. Sarnthein, Kluwer Academic Publishers, Dordrecht, The Netherlands, 1989, pp. 359–384 Search PubMed.
- J. M. Coe and S. E. Lindberg, The morphology and size distribution of atmospheric particles deposited on foliage and inert surfaces, JAPCA, 1987, 37, 237–243 CrossRef CAS.
-
M. J. Zufall and C. I. Davidson, in Air Pollution in the Ural Mountains: Environmental, Health and Policy Aspects, ed. I. Linkov and R. Wilson, Springer Netherlands, Dordrecht, 1998, pp. 55–73 Search PubMed.
- L. Schütz, Long range transport of desert dust with special emphasis on the Sahara, Ann. N. Y. Acad. Sci., 1980, 338, 515–532 CrossRef.
- K. T. Whitby, The physical characteristics of sulfur aerosols, Atmos. Environ. (1967), 1978, 12, 135–159 CrossRef CAS.
- L. Schütz and K. A. Rahn, Trace element concentrations in erodible soils, Atmos. Environ., 1982, 16, 171–176 CrossRef.
- V. Goldschmidt, The principles of distribution of chemical elements in minerals and rocks, J. Chem. Soc., 1937, 655–673 RSC.
- J. B. F. Champlin and H. N. Dunning, A geochemical investigation of the Athabasca bituminous sands, Econ. Geol., 1960, 55, 797–804 CrossRef.
- K. Clark, Athabasca bituminous sands, Fuel, 1951, 49–53 CAS.
-
J. A. Bichard, Oil Sands Composition and Behaviour Research: The Research Papers of John A. Bichard, 1957–1965, Alberta Oil Sands Technology and Research Authority, Edmonton Alta, Canada, 1987 Search PubMed.
-
C. Tomasi and A. Lupi, in Atmospheric Aerosols, ed. C. Tomasi, S. Fuzzi and A. A. Kokhanovsky, Wiley-VCH, Weinheim, 1st edn, 2016, pp. 1–86 Search PubMed.
-
M. L. Corrin and D. F. Natusch, in Lead in the Environment, ed. W. R. Boggess and B. G. Wixson, Castle House Publications, 1979 Search PubMed.
- M. Xu, D. Yu, H. Yao, X. Liu and Y. Qiao, Coal combustion-generated aerosols: Formation and properties, Proc. Combust. Inst., 2011, 33, 1681–1697 CrossRef CAS.
- Y. Gao, E. Nelson, M. Field, Q. Ding, H. Li, R. Sherrell, C. Gigliotti, D. van Ry, T. Glenn and S. Eisenreich, Characterization of atmospheric trace elements on PM2.5 particulate matter over the New York–New Jersey harbor estuary, Atmos. Environ., 2002, 36, 1077–1086 CrossRef CAS.
- H.-N. Jang, Y.-C. Seo, J.-H. Lee, K.-W. Hwang, J.-I. Yoo, C.-H. Sok and S.-H. Kim, Formation of fine particles enriched by V and Ni from heavy oil combustion: Anthropogenic sources and drop-tube furnace experiments, Atmos. Environ., 2007, 41, 1053–1063 CrossRef CAS.
-
P. Kulkarni, P. A. Baron and K. Willeke, Aerosol Measurement. Principles, Techniques, and Applications, Wiley, Hoboken, N.J., 3rd edn, 2011 Search PubMed.
- D. F. S. Natusch, J. R. Wallace and C. A. Evans, Toxic trace elements: Preferential concentration in respirable particles, Science, 1974, 183, 202–204 CrossRef CAS.
- J. O. Nriagu, Global metal pollution: Poisoning the biosphere?, Environ.: Sci. Policy Sustainable Dev., 1990, 32, 7–33 Search PubMed.
- M. Amodio, S. Catino, P. R. Dambruoso, G. de Gennaro, A. Di Gilio, P. Giungato, E. Laiola, A. Marzocca, A. Mazzone, A. Sardaro and M. Tutino, Atmospheric deposition: Sampling procedures, analytical methods, and main recent findings from the scientific literature, Adv. Meteorol., 2014, 2014, 1–27 Search PubMed.
- V. A. Dauvalter, N. Kashulin, J. Lehto and J. Jernstrom, Chalcophile elements Hg, Cd, Pb, As in Lake Umbozero, Murmansk Region, Russia, Int. J. Environ. Res. Public Health, 2009, 3, 411–428 CAS.
- A. Robache, F. Mathé, J. C. Galloo and R. Guillermo, Multi-element analysis by inductively coupled plasma optical emission spectrometry of airborne particulate matter collected with a low-pressure cascade impactor, Analyst, 2000, 125, 1855–1859 RSC.
- R. L. Davison, D. F. S. Natusch, J. R. Wallace and C. A. Evans, Trace elements in fly ash, Environ. Sci. Technol., 1974, 8, 1107–1113 CrossRef CAS.
- S. Mbengue, L. Y. Alleman and P. Flament, Bioaccessibility of trace elements in fine and ultrafine atmospheric particles in an industrial environment, Environ. Geochem. Health, 2015, 37, 875–889 CrossRef CAS.
- S. Balachandran, B. R. Meena and P. S. Khillare, Particle size distribution and its elemental composition in the ambient air of Delhi, Environ. Int., 2000, 26, 49–54 CrossRef CAS.
-
D. Rao, in Bryophyte Ecology, ed. A. Smith, Chapman and Hall Ltd, Holborn, London, UK, 1982, pp. 445–471 Search PubMed.
- D. Rao, G. Robitaille and F. LeBlanc, Influence of heavy metal pollution on lichens and bryophytes, J. Hattori Bot. Lab., 1977, 42, 213–239 CAS.
-
M. H. Martin and P. J. Coughtrey, Biological Monitoring of Heavy Metal Pollution. Land and Air, Springer Netherlands, Dordrecht, 1982 Search PubMed.
- G. Tyler, Bryophytes and heavy metals: a literature review, Bot. J. Linn. Soc., 1990, 104, 231–253 CrossRef.
- A. G. Gonzalez, O. S. Pokrovsky, A. K. Beike, R. Reski, A. Di Palma, P. Adamo, S. Giordano and J. Angel Fernandez, Metal and proton adsorption capacities of natural and cloned Sphagnum mosses, J. Colloid Interface Sci., 2016, 461, 326–334 CrossRef CAS PubMed.
-
S. K. Rice, in Encyclopedia of Inland Waters, ed. G. E. Likens, Elsevier, London, 2009, pp. 88–96 Search PubMed.
- W. Barthlott and C. Neinhuis, Purity of the sacred lotus, or escape from contamination in biological surfaces, Planta, 1997, 202, 1–8 CrossRef CAS.
- P. M. Hayward and R. S. Clymo, Profiles of water content and pore size in Sphagnum and peat, and their relation to peat bog ecology, Proc. R. Soc. London, Ser. B, 1982, 215, 299–325 Search PubMed.
- A. Merced, Novel insights on the structure and composition of pseudostomata of Sphagnum, Am. J. Bot., 2015, 102, 329–335 CrossRef.
-
V. Puustjärvi, Peat and its Use in Horticulture, Turveteollisuusliitto, Helsinki, 1977 Search PubMed.
- V. Puustjärvi, On the colloidal nature of peat-forming moss, Arch. Soc. Zool. Bot. Fenn. “Vanamo”, 1955, 9, 257–272 Search PubMed.
- R. Clymo, Ion exchange in Sphagnum and its relation to bog ecology, Ann. Bot., 1963, 27, 309–324 CrossRef CAS.
- S. Ballance, K. A. Kristiansen, N. T. Skogaker, K. E. Tvedt and B. E. Christensen, The localisation of pectin in Sphagnum moss leaves and its role in preservation, Carbohydr. Polym., 2012, 87, 1326–1332 CrossRef CAS.
- A. G. González and O. S. Pokrovsky, Metal adsorption on mosses: Toward a universal adsorption model, J. Colloid Interface Sci., 2014, 415, 169–178 CrossRef PubMed.
- A. Saxena, D. K. Saxena and H. S. Srivastava, The influence of glutathione on physiological effects of lead and its accumulation in moss Sphagnum Squarrosum, Water, Air, Soil Pollut., 2003, 143, 351–361 CrossRef CAS.
- H. Kempter, M. Krachler, W. Shotyk and C. Zaccone, Major and trace elements in Sphagnum moss from four southern German bogs, and comparison with available moss monitoring data, Ecol. Indic., 2017, 78, 19–25 CrossRef CAS.
- O. W. Archibold, The metal content of wind-blown dust from uranium tailings in Northern Saskatchewan, Water, Air, Soil Pollut., 1985, 24, 63–76 CrossRef CAS.
- A. Gupta, Heavy metal accumulation by three species of mosses in Shillong, North-Eastern India, Water, Air, Soil Pollut., 1995, 82, 751–756 CrossRef CAS.
- A. W. Ireland, M. J. Clifford and R. K. Booth, Widespread dust deposition on North American peatlands coincident with European land-clearance, Veget. Hist. Archaeobot., 2014, 23, 693–700 CrossRef.
- H. Salo, P. Paturi and J. Mäkinen, Moss bag (Sphagnum papillosum) magnetic and elemental properties for characterising seasonal and spatial variation in urban pollution, Int. J. Environ. Sci. Technol., 2016, 13, 1515–1524 CrossRef CAS.
- R. K. Wieder, K. D. Scott, M. A. Vile and C. Herron, Are bog plant/lichen tissue concentrations of Ca, Mg, K, and P affected by fugitive dust released from oil sands development in the Fort McMurray region of Alberta?, Sci. Total Environ., 2022, 849, 157684 CrossRef CAS PubMed.
- X. Li, J. Talbot, J. King and M. Wang, Effects of road dust on vegetation composition and surface chemistry of three ombrotrophic peatlands in eastern Canada, Geoderma, 2023, 439, 116665 CrossRef.
- M. Salemaa, J. Derome, H.-S. Helmisaari, T. Nieminen and I. Vanha-Majamaa, Element accumulation in boreal bryophytes, lichens and vascular plants exposed to heavy metal and sulfur deposition in Finland, Sci. Total Environ., 2004, 324, 141–160 CrossRef CAS PubMed.
- H. Harmens, A. Buse, P. Buker, D. Norris, G. Mills, B. Williams, B. Reynolds, T. W. Ashenden, A. Ruhling and E. Steinnes, Heavy metal concentrations in European mosses: 2000/2001 survey, J. Atmos. Chem., 2004, 49, 425–436 CrossRef CAS.
- E. Steinnes, T. Berg and H. T. Uggerud, Three decades of atmospheric metal deposition in Norway as evident from analysis of moss samples, Sci. Total Environ., 2011, 412–413, 351–358 CrossRef CAS PubMed.
- P. Pakarinen and K. Tolonen, Regional survey of heavy metals in peat mosses (Sphagnum), Ambio, 1976, 5, 38–40 CAS.
- T. Berg, O. Røyset and E. Steinnes, Moss (Hylocomium splendens) used as biomonitor of atmospheric trace element deposition: Estimation of uptake efficiencies, Atmos. Environ., 1995, 29, 353–360 CrossRef CAS.
- K. Pilegaard, Heavy metals in bulk precipitation and transplanted Hypogymnia physodes and Dicranoweisia cirrata in the vicinity of a Danish steelworks, Water, Air, Soil Pollut., 1979, 11, 77–91 CrossRef CAS.
- G. Kosior, M. V. Frontasyeva, Z. Ziembik, I. Zincovscaia, A. Dołhańczuk-Śródka and B. Godzik, The moss biomonitoring method and neutron activation analysis in assessing pollution by trace elements in selected Polish national parks, Arch. Environ. Contam. Toxicol., 2020, 79, 310–320 CrossRef CAS PubMed.
- T. Berg and E. Steinnes, Recent trends in atmospheric deposition of trace elements in Norway as evident from the 1995 moss survey, Sci. Total Environ., 1997, 208, 197–206 CrossRef CAS PubMed.
- M. V. Santelmann and E. Gorham, The influence of airborne road dust on the chemistry of Sphagnum mosses, J. Ecol., 1988, 76, 1219 CrossRef CAS.
- Å. Rühling, G. Tyler and A. Ruhling, Sorption and retention of heavy metals in the woodland moss Hylocomium splendens (Hedw.) Br. et Sch, Oikos, 1970, 21, 92 CrossRef.
- G. Mullan-Boudreau, R. Belland, K. Devito, T. Noernberg, R. Pelletier and W. Shotyk,
Sphagnum moss as an indicator of contemporary rates of atmospheric dust deposition in the Athabasca Bituminous Sands Region, Environ. Sci. Technol., 2017, 51, 7422–7431 CrossRef CAS PubMed.
- E. N. Kelly, D. W. Schindler, P. V. Hodson, J. W. Short, R. Radmanovich and C. C. Nielsen, Oil sands development contributes elements toxic at low concentrations to the Athabasca River and its tributaries, Proc. Natl. Acad. Sci. U.S.A., 2010, 107, 16178–16183 CrossRef CAS PubMed.
- B. Weinhold, Alberta's oil sands: hard evidence, missing data, new promises, Environ. Health Perspect., 2011, 119, a126–a131 Search PubMed.
- A. Sapkota, A. K. Chebukin, G. Bonani and W. Shotyk, Six millennia of atmospheric dust deposition in southern South America (Isla Navarino, Chile), Holocene, 2007, 17, 561–572 CrossRef.
- W. Shotyk, B. Bicalho, C. W. Cuss, J. Duke, T. Noernberg, R. Pelletier, E. Steinnes and C. Zaccone, Dust is the dominant source of “heavy metals” to peat moss (Sphagnum fuscum) in the bogs of the Athabasca Bituminous Sands region of northern Alberta, Environ. Int., 2016, 92–93, 494–506 CrossRef CAS PubMed.
- X. Chen and R. S. Houk, Polyatomic ions as internal standards for matrix corrections in inductively coupled plasma mass spectrometry, J. Anal. At. Spectrom., 1995, 10, 837 RSC.
-
R Core Team, R: A Language and Environment for Statistical Computing, R Foundation for Statistical Computing, Vienna, Austria, 2021 Search PubMed.
-
D. Kahle and H. Wickham, ggmap: Spatial Visualization with ggplot2, R. J., 2013, vol. 5, pp. 144–161 Search PubMed.
-
H. Wickham, ggplot2: Elegrant Graphics for Data Analysis, Springer, Switzerland, 2nd edn, 2016 Search PubMed.
-
A. Kassambara and F. Mundt, factoextra: Extract and Visualize the Results of Multivariate Data Analyses, Comprehensive R Archive Network (CRAN), 2020 Search PubMed.
-
A. Kassambara, ggcorrplot: Visualization of a Correlation Matrix Using ‘ggplot2’, Comprehensive R Archive Network (CRAN), 2019 Search PubMed.
-
R. L. Rudnick and S. Gao, in Treatise on Geochemistry, ed. H. Holland and K. Turekian, Elsevier, 2nd edn, 2014, pp. 1–51 Search PubMed.
- W. Shotyk, D. Weiss, J. D. Kramers, R. Frei, A. K. Chebukin, M. Gloor and S. Reese, Geochemistry of the peat bog at Etang de la Grue're, Jura Mountains, Switzerland, and its record of atmospheric Pb and lithogenic trace metals (Sc, Ti, Y, Zr, and REE) since 12,370 14C yr BP, Geochim. Cosmochim. Acta, 2001, 65, 2337–2360 CrossRef CAS.
-
M. S. Wickleder, B. Fourest and P. K. Dorhout, in The Chemistry of the Actinide and Transactinide Elements, ed. L. R. Morss, N. M. Edelstein and J. Fuger, Springer, Dordrecht, 4th edn., 2010, pp. 52–160 Search PubMed.
- S. S. Goldich, A study in rock-weathering, J. Geol., 1938, 46, 17–58 CrossRef CAS.
-
G. Mellon, Geology of the McMurray Formation. Part II: Heavy Minerals of the McMurray, Edmonton, AB, CA, 1956, https://ags.aer.ca/document/REP/REP_72.pdf Search PubMed.
- K. K. Donkor, B. Kratochvil and M. J. M. Duke, Estimation of the fines content of Athabasca oil sands using instrumental neutron activation analysis, Can. J. Chem., 1996, 74, 583–590 CrossRef CAS.
-
K. A. Rahn, The Chemical Composition of the Atmospheric Aerosol, Graduate School of Oceanography, University of Rhode Island, Kingston, Rhode Island, USA, 1976 Search PubMed.
- P. R. Harrison, K. A. Rahn, R. Dams, J. A. Robbins, J. W. Winchester, S. S. Brar and D. M. Nelson, Areawide trace metal concentrations measured by multielement neutron activation analysis, J. Air Pollut. Control Assoc., 1971, 21, 563–570 CrossRef CAS.
- W. Shotyk, W. H. Nesbitt and W. S. Fyfe, Natural and anthropogenic enrichments of trace metals in peat profiles, Int. J. Coal Geol., 1992, 20, 49–84 CrossRef CAS.
- B. Bicalho, I. Grant-Weaver, C. Sinn, M. W. Donner, S. Woodland, G. Pearson, S. Larter, J. Duke and W. Shotyk, Determination of ultratrace (<0.1 mg/kg) elements in Athabasca Bituminous Sands mineral and bitumen fractions using inductively coupled plasma sector field mass spectrometry (ICP-SFMS), Fuel, 2017, 206, 248–257 CrossRef CAS.
- W. Shotyk and C. W. Cuss, Atmospheric Hg accumulation rates determined using Sphagnum moss from ombrotrophic (rain-fed) bogs in the Athabasca Bituminous Sands region of northern Alberta, Canada, Ecol. Indicat., 2019, 107, 105626 CrossRef CAS.
- M. B. Javed, C. W. Cuss, I. Grant-Weaver and W. Shotyk, Size-resolved Pb distribution in the Athabasca River shows snowmelt in the bituminous sands region an insignificant source of dissolved Pb, Sci. Rep., 2017, 7, 43622 CrossRef PubMed.
- W. Shotyk, Trace elements in wild berries from reclaimed lands: Biomonitors of contamination by atmospheric dust, Ecol. Indicat., 2020, 110, 105960 CrossRef CAS.
- D. Weiss, W. Shotyk, A. K. Cheburkin, M. Gloor and S. Reese, Atmospheric lead deposition from 12,400 to ca. 2,000 yrs BP in a peat bog profile, Jura Mountains, Switzerland, Water, Air, Soil Pollut., 1997, 100, 311–324 CrossRef CAS.
- J. M. Robertson, J. A. Nesbitt and M. B. J. Lindsay, Aqueous- and solid-phase molybdenum geochemistry of oil sands fluid petroleum coke deposits, Alberta, Canada, Chemosphere, 2019, 217, 715–723 CrossRef CAS.
- S. Dehkissia, F. Larachi and E. Chornet, Catalytic (Mo) upgrading of Athabasca bitumen vacuum bottoms via two-step hydrocracking and enhancement of Mo–heavy oil interaction, Fuel, 2004, 83, 1323–1331 CrossRef CAS.
- P. J. White and P. H. Brown, Plant nutrition for sustainable development and global health, Ann. Bot., 2010, 105, 1073–1080 CrossRef CAS PubMed.
- S. Stachiw, B. Bicalho, I. Grant-Weaver, T. Noernberg and W. Shotyk, Trace elements in berries collected near upgraders and open pit mines in the Athabasca Bituminous Sands Region (ABSR): Distinguishing atmospheric dust deposition from plant uptake, Sci. Total Environ., 2019, 670, 849–864 CrossRef CAS PubMed.
- M. Anicić, M. V. Frontasyeva, M. Tomasević and A. Popović, Assessment of atmospheric deposition of heavy metals and other elements in Belgrade using the moss biomonitoring technique and neutron activation analysis, Environ. Monit. Assess., 2007, 129, 207–219 CrossRef PubMed.
- E. Steinnes, A critical evaluation of the use of naturally growing moss to monitor the deposition of atmospheric metals, Sci. Total Environ., 1995, 160–161, 243–249 CrossRef.
- S. Dragović and N. Mihailović, Analysis of mosses and topsoils for detecting sources of heavy metal pollution: multivariate and enrichment factor analysis, Environ. Monit. Assess., 2009, 157, 383–390 CrossRef.
- K. J. Reddy and S. P. Gloss, Geochemical speciation as related to the mobility of F, Mo and Se in soil leachates, Appl. Geochem., 1993, 8, 159–163 CrossRef.
- W. Zimmer and R. Mendel, Molybdenum metabolism in plants, Plant Biol., 1999, 1, 160–168 CrossRef CAS.
- S. M. Shaheen, D. S. Alessi, F. M. G. Tack, Y. S. Ok, K.-H. Kim, J. P. Gustafsson, D. L. Sparks and J. Rinklebe, Redox chemistry of vanadium in soils and sediments: Interactions with colloidal materials, mobilization, speciation, and relevant environmental implications-A review, Adv. Colloid Interface Sci., 2019, 265, 1–13 CrossRef CAS PubMed.
- M. Brzóska and J. Moniuszko-Jakoniuk, Interactions between cadmium and zinc in the organism, Food Chem. Toxicol., 2001, 39, 967–980 CrossRef.
- C. H. Hill and G. Matrone, Chemical parameters in the study of in vivo and in vitro interactions of transition elements, Federation, 1970, 29, 1474–1481 CAS.
- M. D. Vazquez, O. Wappelhorst and B. Markert, Determination of 28 elements in aquatic moss Fontinalis Antipyretica Hedw. and water from the upper reaches of the River Nysa (CZ, D), by ICP-MS, ICP-OES and AAS, Water, Air, Soil Pollut., 2004, 152, 153–172 CrossRef CAS.
- H. V. Koontz and K. L. Berle, Silver uptake, distribution, and effect on calcium, phosphorus, and sulfur uptake, Plant Physiol., 1980, 65, 336–339 CrossRef CAS PubMed.
- K. C. Jones and P. J. Peterson, The influence of humic and fulvic acids on silver uptake by perennial ryegrass, and its relevance to the cycling of silver in soils, Plant Soil, 1986, 95, 3–8 CrossRef CAS.
- Y. Zhi, K. He, T. Sun, Y. Zhu and Q. Zhou, Assessment of potential soybean cadmium excluder cultivars at different concentrations of Cd in soils, J. Environ. Sci., 2015, 35, 108–114 CrossRef CAS.
- J. Shentu, Z. He, X. E. Yang and T. Li, Accumulation properties of cadmium in a selected vegetable-rotation system of southeastern China, J. Agric. Food Chem., 2008, 56, 6382–6388 CrossRef CAS PubMed.
- A. Jamers, R. Blust, W. de Coen, J. L. Griffin and O. A. H. Jones, An omics based assessment of cadmium toxicity in the green alga Chlamydomonas reinhardtii, Aquat. Toxicol., 2013, 126, 355–364 CrossRef CAS PubMed.
- L. Di Sanità Toppi and R. Gabbrielli, Response to cadmium in higher plants, Environ. Exp. Bot., 1999, 41, 105–130 CrossRef.
- X. Huang, S. Duan, Q. Wu, M. Yu and S. Shabala, Reducing cadmium accumulation in plants: Structure-function relations and tissue-specific operation of transporters in the spotlight, Plants (Basel, Switzerland), 2020, 9, 223 CAS.
- S. Thomine, R. Wang, J. M. Ward, N. M. Crawford and J. I. Schroeder, Cadmium and iron transport by members of a plant metal transporter family in Arabidopsis with homology to Nramp genes, Proc. Natl. Acad. Sci. U.S.A., 2000, 97, 4991–4996 CrossRef CAS PubMed.
- Y. Jiang, M. Fan, R. Hu, J. Zhao and Y. Wu, Mosses are better than leaves of vascular plants in monitoring atmospheric heavy metal pollution in urban areas, Int. J. Environ. Res. Public Health, 2018, 15, 1105 CrossRef PubMed.
- M. Osacky, M. Geramian, D. G. Ivey, Q. Liu and T. H. Etsell, Mineralogical and chemical composition of petrologic end members of Alberta oil sands, Fuel, 2013, 113, 148–157 CrossRef CAS.
- M. Osacky, M. Geramian, M. D. Dyar, E. C. Sklute, M. Valter, D. G. Ivey, Q. Liu and T. H. Etsell, Characterisation of petrologic end members of oil sands from the athabasca region, Alberta, Canada, Can. J. Chem. Eng., 2013, 91, 1402–1415 CrossRef CAS.
-
M. A. Hooshiar Fard, PhD thesis, University of Alberta Libraries, 2011.
-
Insecticides Design Using Advanced Technologies, ed. I. Ishaaya, A. R. Horowitz and R. Nauen, Springer, Berlin, Heidelberg, 2007 Search PubMed.
-
Government of Alberta, Lower Athabasca Region: Tailings Management Framework for the Mineable Athabasca Oil Sands, Edmonton, Alberta, 2015, https://open.alberta.ca/dataset/9781460121740/resource/7c49eb63-751b-49fd-b746-87d5edee3131 Search PubMed.
-
M. E. Essington, Soil and Water Chemistry: An Integrative Approach, CRC Press, Boca Raton, 2004 Search PubMed.
- W. Mertz, The essential trace elements, Science, 1981, 213, 1332–1338 CrossRef CAS PubMed.
-
Essential Plant Nutrients. Uptake, Use Efficiency, and Management, ed. M. Naeem, A. A. Ansari and S. S. Gill, Springer International Publishing, Cham, 2017 Search PubMed.
- A. S. Relman, The physiological behavior of rubidium and cesium in relation to that of potassium, Yale J. Biol. Med., 1956, 29, 248–262 CAS.
-
N. N. Greenwood and A. Earnshaw, Chemistry of the Elements, Butterworth-Heinemann, Oxford, 2nd edn, 1997 Search PubMed.
- N. Abdu and I. Mohammed, Adsorption-solubility equilibria and speciation of Pb, Cd, and Zn in a savanna soil, Span. J. Soil Sci., 2016, 6, 244–260 Search PubMed.
- M. Shariat and M. Hassani, Rhenium recovery from Sarcheshmeh molybdenite concentrate, J. Mater. Process. Technol., 1998, 74, 243–250 CrossRef.
- R. D. Shannon, Revised effective ionic radii and systematic studies of interatomic distances in halides and chalcogenides, Acta Crystallogr., Sect. A: Cryst. Phys., Diffr., Theor. Gen. Crystallogr., 1976, 32, 751–767 CrossRef.
- C. Maschowski, M. C. Zangna, G. Trouvé and R. Gieré, Bottom ash of trees from Cameroon as fertilizer, Appl. Geochem., 2016, 72, 88–96 CrossRef CAS.
- A. A. Shaltout, M. A. Allam and M. A. Moharram, FTIR spectroscopic, thermal and XRD characterization of hydroxyapatite from new natural sources, Spectrochim. Acta, Part A, 2011, 83, 56–60 CrossRef CAS PubMed.
- K. Breiter, L. Ackerman, J. Ďurišova, M. Svojtka and M. Novák, Trace element composition of quartz from different types of pegmatites: A case study from the Moldanubian Zone of the Bohemian Massif (Czech Republic), Mineral. Mag., 2014, 78, 703–722 CrossRef CAS.
- C. Dietl, W. Reifenhäuser and L. Peichl, Association of antimony with traffic—occurrence
in airborne dust, deposition and accumulation in standardized grass cultures, Sci. Total Environ., 1997, 205, 235–244 CrossRef CAS.
-
W. Shotyk, M. Krachler and B. Chen, in Metal Ions in Biological Systems, ed. A. Sigel, H. Sigel and R. K. O. Sigel, CRC Press, Boca Raton, 2005, pp. 171–204 Search PubMed.
- B. D. Garg, S. H. Cadle, P. A. Mulawa, P. J. Groblicki, C. Laroo and G. A. Parr, Brake wear particulate matter emissions, Environ. Sci. Technol., 2000, 34, 4463–4469 CrossRef CAS.
- P. Wåhlin, R. Berkowicz and F. Palmgren, Characterisation of traffic-generated particulate matter in Copenhagen, Atmos. Environ., 2006, 40, 2151–2159 CrossRef.
- M. Fort, J. O. Grimalt, X. Querol, M. Casas and J. Sunyer, Evaluation of atmospheric inputs as possible sources of antimony in pregnant women from urban areas, Sci. Total Environ., 2016, 544, 391–399 CrossRef CAS PubMed.
- J. van Keulen and B. A. Young, Evaluation of acid-insoluble ash as a natural marker in ruminant digestibility studies, J. Anim. Sci., 1977, 44, 282–287 CrossRef.
- K. Liu, New and improved methods for measuring acid insoluble ash, Anim. Feed Sci. Technol., 2022, 288, 115282 CrossRef CAS.
- D. Kim, B. Kim, E. Yun, J. Kim, Y. Chae and S. Park, Statistical quality control of total ash, acid-insoluble ash, loss on drying, and hazardous heavy metals contained in the component medicinal herbs of “Ssanghwatang”, a widely used oriental formula in Korea, J. Nat. Med., 2013, 67, 27–35 CrossRef CAS PubMed.
- A. W. H. Damman, K. Tolonen and T. Sallantaus, Element retention and removal in ombrotrophic peat of Haadetkeidas, a boreal Finnish peat bog, Suo, 1992, 43, 137–145 Search PubMed.
- P. Steinmann and W. Shotyk, Geochemistry, mineralogy, and geochemical mass balance on major elements in two peat bog profiles (Jura Mountains, Switzerland), Chem. Geol., 1997, 138, 25–53 CrossRef CAS.
- D. Weiss, W. Shotyk, A. K. Cheburkin and M. Gloor, Determination of Pb in the ash fraction of plants and peats using the Energy-dispersive Miniprobe Multielement Analyser (EMMA), Analyst, 1998, 123, 2097–2102 RSC.
- W. S. Clough, The deposition of particles on moss and grass surfaces, Atmos. Environ. (1967), 1975, 9, 1113–1119 CrossRef.
Footnotes |
† Electronic supplementary information (ESI) available. See DOI: https://doi.org/10.1039/d3ea00071k |
‡ Current address: H2nanO, 4633 92 Ave NW, Edmonton, AB T6B 2J4, Canada. |
§ Current address: Hatfield Consultants, Fort McMurray, AB T9H 4C4, Canada. |
¶ Current address: Modern West Advisory, Suite 506, 10104 103 Ave NW, Edmonton, AB T5J 3G1, Canada. |
|| Current address: School of Science and the Environment, Grenfell Campus, Memorial University of Newfoundland, Corner Brook, NL A2H 5G5, Canada. |
|
This journal is © The Royal Society of Chemistry 2024 |
Click here to see how this site uses Cookies. View our privacy policy here.