Effects of fuel composition and vehicle operating temperature on in vitro toxicity of exhaust emissions†
Received
12th September 2023
, Accepted 8th March 2024
First published on 12th March 2024
Abstract
Traffic as an important part of the energy sector significantly contributes to global air pollution. To mitigate the hazardous components of traffic emissions regulations have been implemented resulting in technological solutions such as exhaust after-treatment systems. However, fuels also play a crucial role in emissions and components such as the aromatic compounds in fuel have been linked to increased exhaust emissions. Several current emissions regulations neglect environmental factors, such as cold operating temperatures, that can significantly increase emissions. Moreover, the effect of fuel aromatics and cold temperature on emissions toxicity has not been adequately studied. This study evaluates the impact of after-treatment systems, aromatic fuel content, and cold operating temperature on emission toxicity. To achieve this, four different light-duty vehicles were used in a temperature-controlled dynamometer room, with a co-culture of A549 and THP-1 cell lines exposed to online exhaust emissions using a thermophoresis-based air–liquid interface (ALI) system. The results demonstrate that the aromatic content of both diesel and gasoline fuels increases exhaust toxicity. The study additionally emphasises the potential of particulate filters as after-treatment systems to reduce the toxicity of emissions and highlights how cold running temperatures result in higher exhaust toxicity. The study also assessed the diesel particulate filter (DPF) active regeneration event, which leads to multi-fold emissions and higher toxicological responses. Overall, the study provides crucial novel results on how various factors affect the toxicity of exhaust emissions from modern light-duty vehicles, providing insights into decreasing the emissions from this energy sector.
Environmental significance
The traffic sector is one of the main energy users globally with significant contributions to global emissions and air pollution. Despite the increasingly strict emissions regulations, several exhaust components such as ultra-fine particulates and black carbon still pose a significant risk to public health and climate change. Therefore, decreasing the harmful emissions from the traffic sector is of high importance. One of the methods to reveal the most toxic exhaust components is the utilisation of novel in vitro methods, such as air–liquid interface (ALI) exposure systems. In the present study, thermophoresis-based ALI was used to evaluate the toxicity of exhaust emissions and how factors such as fuel composition, after-treatment systems, and operating temperatures affect the emission toxicity. Results revealed that the higher aromatic content of diesel and gasoline fuel corresponds to the increase in PM emissions and higher toxicological potential. The importance of after-treatment systems, especially the particle filters, was highlighted. Cold operating temperature further increased the emissions, especially those of PM and gaseous compounds such as NOx. The study provides crucial and novel information about light-duty vehicle emissions and how their toxicological and environmental effects could be decreased with solutions such as cleaner fuels and after-treatment technologies.
|
1 Introduction
Traffic is one of the major atmospheric emission sources, accounting globally for up to 27% of particulate matter (PM) pollution in urban areas and one-quarter of greenhouse gas emissions.1–3 Overall, decreasing air pollution from traffic would have a beneficial public health impact. To mitigate exhaust emissions, several emissions standards have been introduced globally to the vehicle fleet, such as the Euro emissions standards in the EU, Tier standards in the US, L standards in Brazil, and the China emissions standards in China.5–10 Consequently, the hazardous components of the exhaust have been decreasing in countries with emission regulations standards11 and the effect of emissions regulations on traffic exhaust toxicity has been demonstrated in vitro.12,13 Despite this, over 99% of the global population lives in environments with ambient air pollution concentrations exceeding the WHO limits.4 Air quality in developing countries is especially impacted by traffic, with growth in fleet numbers and a lack of emissions regulations.11 The current emissions regulations are constantly progressing, with future inclusions of traffic emission components such as the smallest particulate matter fractions, semi-volatile organic compounds (SVOC), and tire wear. Therefore, multi-technical solutions, such as after-treatment systems and cleaner fuels, are still considered necessary globally to decrease traffic emissions further.4,14,15 After-treatment systems are developed to decrease the concentrations of hazardous components of the exhaust. Systems such as three-way catalysts (TWCs) reduce emissions by converting hazardous pollutants to less hazardous ones with catalytic reactions. Particulate filters, such as the diesel particulate filter (DPF), decrease the PM emissions by use of honeycomb structure filters, efficiently decreasing PM emissions.16,17 Notably, in most cases, the cost of air pollution management is shown to be outweighed by the benefits of clean air policies.18
Fuel composition is an aspect that has important consequences on vehicle emissions and different chemical compositions have immense potential to decrease emissions, particularly in vehicles without any after-treatment systems. An example of this is the ethanol concentration of gasoline, with the higher ethanol content decreasing exhaust emission concentrations and toxicity.12,19,20 Another important fuel component is unsaturated aromatic hydrocarbons. The concentration of aromatic hydrocarbons in both diesel and gasoline fuels has been associated with an increase in exhaust emissions concentrations and toxicological potential.21–24 Environmental factors can also affect emissions and conditions such as cold operation temperatures have been demonstrated to increase PM concentrations in exhaust.25,26 Thus far, emissions from cold-start and operating are included in a few emissions regulations and testing, one example being the European type 6 test. Similar testing measures are included in the USA (CFR 1066 Subpart H) (US. EPA), South Korea (MOE, 2014), and China (China 6, 2017) regulations. However, so far, there have been only a few studies regarding the toxicity of emissions at cold temperatures.12
Increasingly combining the novel knowledge from toxicological and epidemiological studies with emissions regulations would be highly impactful.4In vitro toxicological research is one of the methods to further reveal the most hazardous aspects of traffic exhaust, and several in vitro submerged studies have been conducted on the toxicity of particulate matter in exhaust emissions.12,27,28 However, toxicological experiments should increasingly represent the actual biological system to better establish the data from the most toxic emission components.28 A possible solution for this would be advanced in vitro models, such as air–liquid interface (ALI) exposure systems, where the cells can be exposed to total exhaust emissions, including gaseous emissions such as SVOCs and nitrogen oxides (NOx).29–32 Additional systems, such as primary cells and organ-on-a-chip systems, would provide further advantages to presenting real-life exposure.33 Toxicological studies should also be combined with a detailed examination of the aerosol, increasingly identifying the components in emissions that prominently impact human health.34 Overall, toxicological studies could provide vital information to further improve emissions regulations.
The present study aimed to examine how the aromatic content of the fuel, after-treatment systems, and temperature affect exhaust emission toxicity. One emphasis in the after-treatment systems was on the gasoline particle filter (GPF), as the PM emissions from modern gasoline vehicles are substantially higher than those from modern diesel vehicles.35 For the in vitro exhaust exposure experiments, a thermophoresis-based ALI system with a co-culture of A549 and THP secondary cell lines was utilised. A total of four different light-duty vehicles complying with the most recent Euro 6d emission regulation were used at warm and cold running temperatures. Detailed results from light-duty vehicle emissions are presented in the study of Saarikoski et al., 2023.36 Following the in vitro exhaust exposure, several toxicological analyses were conducted to reveal the toxicity of different exhaust emissions.
2 Materials and methods
2.1 Vehicle exhaust experiments
In the present study, four different vehicles with distinct fuel options and after-treatment systems were run at two different temperatures (Table 1.). Vehicle and fuel properties are presented in more detail in the ESI.† Briefly, the vehicles were preconditioned in the temperature-controlled dynamometer room overnight until the emission measurement test on each vehicle. Before the measurement run, the vehicles were moved on the dynamometer without starting the engine; thus, the engine experienced a cold start in all measurements. The driving cycle was a modification of the Worldwide Harmonized Light Vehicles Test Cycle (WLTC) test cycle. To achieve enough emission exposure for ALI measurements, two consecutive WLTC cycles were combined, resulting in a test cycle with a total of 1 hour duration. The instrumental setup is shown in ESI S1 Fig. 1.†
Table 1 Test point descriptions. CI = compressed ignition, SI = spark ignition, TDI = turbocharged direct injection, TSI = turbocharged stratified injection, DPF = diesel particulate filter, SCR = selective catalyst reduction, DOC = diesel oxidative catalyst, GPF = gasoline particulate filter, TWC = three-way catalyst, CNG = compressed natural gas
Vehicle |
Diesel, Euro 6d |
Gasoline, Euro 6d |
Gasoline, Euro 6d T GPF |
NGV, Euro 6d CNG |
Abbrev. |
Diesel DPF |
Gasoline |
Gasoline GPF |
CNG |
Fuels |
EN590 |
EN228 |
EN228 |
CNG |
HVO |
Alkylate |
After treatment |
DPF, SCR, DOC |
TWC |
TWC, GPF |
TWC |
Temperatures (°C) |
Cold |
Cold |
Cold |
Cold |
Warm |
Warm |
Warm |
Warm |
Ignition system |
CI |
SI |
SI |
SI |
Injection system |
TDI |
MPFI |
TSI |
TSI |
Displacement (cm3) |
1598 |
1199 |
999 |
1498 |
Power (kW) |
85 |
55 |
81 |
96 |
Torque (N m) |
250 |
110 |
200 |
200 |
A total of five fuel options were used in the study. Two diesel fuels with different aromatic contents were used with the diesel vehicle, fossil fuel “EN590” specification with 20 wt% total aromatics representing conventional diesel and essentially aromatic-free bio-based hydrotreated vegetable oil type diesel fuel containing 0.1 wt% total aromatics, referred to as “HVO” in the study. For gasoline vehicles, the fuels were the regular “E228” gasoline with approximately 34 wt% aromatics, and “alkylate” with 0 wt% aromatics. The light-duty vehicle fuelled by compressed natural gas used commercially available “CNG”.
In addition to aromatic content, several other properties between the fuels varied substantially, such as cetane number. Fuel properties were analysed by ASG Analytik-Service GmbH and can be seen in ESI S1 Tables 2 and 3.†
2.2 Instrument setup
A detailed introduction to instrument setup is provided in the study of Saarikoski et al., 2023.36 Briefly, the test setup consisted of standard exhaust measurement instrumentation including a constant volume sampler (CVS) dilution system, multicomponent exhaust gas analyser (AMA, AVL List GmbH), and a large set of additional instrumentation divided into two groups (Fig. 1). Before the exhaust reached the ALI system, it was guided across primary dilution (dilution ratio (DR) = 12) with a porous tube diluter (PTD) combined with a residence time tube (RTT), followed by secondary dilution with an ejector diluter (DR = 11). The dilution air temperature was maintained at 30 °C. Together these dilution systems simulate the process of atmospheric dilution of the exhaust.37,38 The dilution air used in the ALI line was compressed air dried with an adsorption unit before being passed through a set of high-efficiency filters. Simultaneous to the ALI exposure, the exhaust was analysed for particle number (PN) concentrations using a condensation particle counter (CPC) battery consisting of CPCs at particle size cut points of 1.2 nm (A10, Airmodus, Finland), 2.5 nm (3756, TSI Inc., USA), 10 nm, and 23 nm (A20, Airmodus, Finland). The chemical composition of the particles was determined with a Soot Particle Aerosol Mass Spectrometer (SP-AMS, Aerodyne Research Inc., US) and equivalent black carbon (eBC) concentration was measured with an Aethalometer (AE33, Magee Scientific, Slovenia39). Moreover, gravimetric PM sampling was conducted from the CVS-diluted sample with PM samples collected on polytetrafluoroethylene membrane filters (Fluoropore, Merck KGaA), and the gaseous components of NOx, NO2, N2O, and NH3 were measured with Fourier-transform infrared spectroscopy (FTIR) (Rowaco AB). A detailed display of the instrument arrangement is available in ESI S1 Fig. 1.†
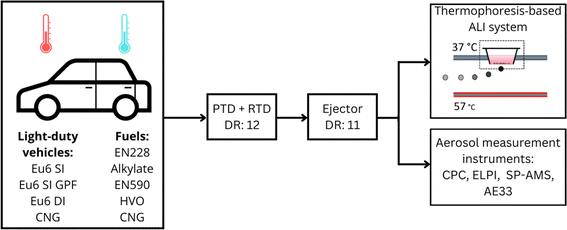 |
| Fig. 1 Simplified overview of the instrumentation set-up. Light-duty vehicle exhaust was directed to the thermophoresis-based ALI exposure system and aerosol measurement instruments under cold and warm operation temperatures. Eu6 = Euro6 emission regulation, DI = direct injection, SI = spark ignition, GPF = gasoline particulate filter, CNG = compressed natural gas, EN228 = regular gasoline, 34% aromatics, alkylate = aromatic free gasoline, HVO = hydrotreated vegetable oil, aromatic free renewable diesel, EN590 = fossil fuel diesel, 20% aromatics, DR = dilution ratio, PTD = porous tube diluter, RTT = residence time tube, ALI = air–liquid interface, CPC = condensation particle counter, ELPI = electrical low-pressure impactor, SP-AMS = soot particle aerosol mass spectrometer, AE33 = aethalometer. | |
2.3 Exposures
The vehicles used different after-treatment systems with the diesel-fuelled vehicles having the diesel particulate filter (DPF), selective catalyst reduction (SCR), and diesel oxidative catalyst (DOC), one gasoline and CNG vehicle equipped with a TWC, and the second gasoline vehicle including a gasoline particle filter (GPF) in addition to a TWC. The diesel vehicle used compression-ignition (CI) and turbocharged direct injection (TDI), while the gasoline vehicle without a GPF used spark ignition (SI) and multi-point fuel injection (MPFI, the gasoline vehicle with a GPF used SI and turbocharged stratified injection (TSI), and the CNG vehicle used SI and TSI) (Table 1). All vehicles complied with the newest European Union Euro6d emissions regulations. The running temperatures for the tests were cold (−3.3 ± 2.4 °C) and warm (22.4 ± 0.2 °C). The repetition of each exposure was three (n = 3), except for the two exposures of HVO at the cold temperature, due to the DPF regeneration event. As the emissions increased multi-fold due to filter regeneration, this exposure was separated into one repetition of the normal circumstance (n = 1) and two repetitions of DPF regeneration (n = 2) in the results. Incubator control (n = 12) was used as a control for the ALI exposure system, clean air control (synthetic air number 4.0, n = 12) as a control for toxicological endpoints, and the positive controls of lipopolysaccharide (LPS) for cytokine and methyl methanesulfonate (MMS) for genotoxicity analyses (n = 12).
Cell lines were cultured in a humidified incubator at +37 °C and 5% CO2 in Dulbecco's Modified Eagle's Medium (DMEM) supplemented with 10% (v/v) fetal bovine serum (FBS), 2 mM L-glutamine, and 1% penicillin and streptomycin (Sigma-Aldrich or Gibco, Life Technologies). A detailed description of the cell culture protocol can be found in our previous studies of Hakkarainen et al., 2022 and 2023.24,40
Briefly, the A549 human alveolar epithelial cells (ATCC®, CCL-158™) were cultured on the basal side of the inserts' membrane four to six days preceding the exposures. The seeding densities of the cells depended on the exposure day and were 400
000 cells per mL, 220
000 cells per mL, 200
000 cells per mL, and 180
000 cells per mL for inserts exposed three, four, five, and six days later, respectively. The ALI environment was formed 48 hours before exhaust exposure by removing the cell medium from the basal side of the inserts. THP-1 human monocyte cells (DSMZ ACC 16; German Collection of Micro-organisms and Cell Cultures; DSMZ, Germany) were differentiated with 0.5 μg mL−1 phorbol-12-myristate-13-acetate (PMA) and seeded on the apical side of the inserts 24 h before the exposures, resulting in approximately 10% of the total cell count being THP-1s.
Preceding the light-duty vehicle exhaust exposure, the medium was replaced with serum-free medium supplemented with 25 mM 4-(2-hydroxyethyl)-1-piperazineethanesulfonic acid (HEPES) buffer (Sigma-Aldrich, USA), for buffering the pH due to lack of CO2 during the exposure. Following the 1 h exposure, the medium was collected, and cells were supplied with a new serum-free medium and incubated for 24 h at +37 °C and 5% CO2. After the incubation, the medium was collected from the inserts 24 h after the exposure for the cytokine analysis.
Trypsin-ethylenediaminetetraacetic acid (EDTA) solution was added to the cells, followed by incubation for 5 min at +37 °C with 5% CO2, detaching the cells from the inserts. To inhibit the trypsin, 100 μL of FBS was added, and cells were collected from the inserts. 60 μL of the cells were mixed with a freezing medium (50% DMEM, 40% FBS, and 10% DMSO) and stored at −80 °C, for genotoxicity analysis.
2.3.1 ALI exposure.
The ALI exposure system used in the present study utilised the thermophoresis effect for the PM deposition. The ALI system has a temperature gradient between the top side (+37 °C) and bottom side (+57 °C), resulting in the uplift force for the aerosols. The cell inserts are on the top side of the equipment and A549 cells, cultured on the basal side of the inserts, are directly exposed to the aerosol. The THP-1 cells cultured on the apical side of the inserts simulate the alveolar macrophages. The main aerosol flow in the ALI was 5 L min−1 with a partial flow of 150 mL min−1 leading to the cells as a laminar flow, and the relative humidity of the ALI system approaching 100%. A detailed description of the ALI system is provided in the article by Ihalainen et al., 2019.29 Deposition of PM number and mass components were calculated from the measured aerosol concentrations, as in our previous studies using the identical ALI system.24,40–42 The deposition estimations in the ALI system are presented in ESI S1 Table S4.†
2.4 Toxicological analysis
2.4.1 Genotoxicity.
Genotoxicity was assessed with a slightly modified alkaline version of single-cell gel electrophoresis (SCGE), also known as comet assay. In the SCGE assay, the cells are lysed within the agarose gel under alkaline conditions, which is succeeded by electrophoresis. The cell nucleoids are revealed by lysing and alkaline conditions make DNA coils more relaxed, thus increasing the robustness of the assay. During the electrophoresis, negatively charged DNA fragments due to strand breaks migrate towards the positive anode. The DNA strands are stained with ethidium bromide and quantified with fluorescence microscopy. The level of genotoxicity is presented by the ratio of migrated DNA compared to unmigrated with data analysis done using the geometric median from the percentage of DNA in the tail. Data are presented as a percentage compared to the clean air control. The detailed procedure for the SCGE assay is described in our previous study.40
2.4.2 Cytokines.
The inflammatory response of the cells was investigated by measuring the concentrations of the chemokine (C–X–C motif) ligand 1 (CXCL1) and interleukin 8 (IL-6) from the cell culture medium collected 24 h after the exposures. The secretion of cytokines was measured using an ELISA kit (R&D Systems, Abington, UK) on 96-well plates (Nunc Maxisorp), according to the manufacturer's instructions. The measurements were conducted with a hybrid multi-mode reader, Synergy H1 (BioTek Instruments, USA), at 450 nm. Data from cytokine measurements are shown as a percentage compared to the clean air control.
2.5 Statistical methods
Significant differences in toxicological endpoints were compared using Welch's one-way analysis of variance (ANOVA) with Dunnett's T3 pairwise comparison, due to the nonparametric distribution. A p-value of <0.05 was considered statistically significant.
The Multivariate Mixed Model (MMM)43 was employed to assess how different aerosol components contribute to observed toxicological responses. This model extends beyond basic mean estimation, also capturing the variance–covariance structure in the data. This is particularly valuable for aerosol measurement data, which often don't meet the standard assumptions of independence and homogeneity required by typical regression or ANOVA models.44 By incorporating a covariance structure, the model improves accuracy and prevents residual autocorrelation. It enhances the linear model (y = βX + ε) by introducing a random component (Zu), where Z represents the design matrix for random covariates (u). Thus, the model is represented as y = βX + Zu + ε. Random effects were assigned to experimental factors like experiment type and sample number to account for the correlated chemical composition within each level of these factors. Moreover, as the DPF regeneration event was such a special event, the effect of it was analysed by the model.
Statistical analyses were performed using IBM SPSS Statistics for Windows, Version 27.0 (IBM Corp. Armonk, NY).
3 Results
3.1 Aerosol results
The emissions from vehicles using different fuels and operating at different temperatures showed significant variation, as shown in Table 2. When using aromatic EN228 fuel and operating at a cold temperature, the vehicle without a GPF had the highest concentrations of PM, BC, and organic matter. However, the use of non-aromatic gasoline fuel significantly reduced emissions in both operating temperatures. The GPF-equipped gasoline vehicle had significantly lower particulate emissions compared to the vehicle without a GPF but had higher gaseous emissions of NOx and N2O. The diesel vehicle had the lowest PM emissions among all vehicles, but an increase in PM emissions was observed with EN590 fuel or at cold operating temperatures. Additionally, the cold temperature led to a significant increase in NOx emissions. Active regeneration of the DPF led to a ten-fold increase in PN emissions, a three-fold increase in PM mass, and a three-fold increase in NOx emissions, especially seen with the rise of NO2 emissions. PM emissions from the CNG-fueled vehicle were higher compared to vehicles equipped with particle filters, and the NH3 emissions were the highest among all vehicles. The cold operating temperature also resulted in higher PM emissions in CNG vehicles. A detailed description of the emissions from light-duty vehicles used in the present study is displayed by Saarikoski et al., 2023.36
Table 2 Distinct light-duty vehicles, fuels, and temperatures used with raw exhaust emissions of PM, eBC, NOx, NO2, N2O, NH3 organic matter mass concentrations, and PN (>10 nm). <DL indicates measurements below the detection limit
Light-duty vehicle |
Fuel |
Temperature |
PM (μg m−3) |
eBC (μg m−3) |
Organic matter (μg m−3) |
NOx (μg m−3) |
NO2 (μg m−3) |
N2O (μg m−3) |
NH3 (μg m−3) |
PN (# cm−3) |
Eu6 SI |
EN228 |
Cold |
49.50 |
28.66 |
8.34 |
184.98 |
0.00 |
1.30 |
82.07 |
4.42 × 104 |
Warm |
11.46 |
7.64 |
1.62 |
162.38 |
0.00 |
0.96 |
41.07 |
9.16 × 103 |
Alkylate |
Cold |
10.63 |
5.80 |
1.35 |
126.63 |
0.00 |
0.97 |
26.10 |
9.12 × 103 |
Warm |
8.64 |
4.80 |
1.44 |
147.82 |
0.00 |
0.96 |
33.60 |
9.15 × 103 |
Eu6 SI GPF |
EN228 |
Cold |
0.95 |
<DL |
0.66 |
192.03 |
0.00 |
5.68 |
24.60 |
2.17 × 102 |
Warm |
0.97 |
<DL |
0.78 |
137.11 |
0.00 |
10.70 |
10.70 |
2.22 × 102 |
Eu6 DI |
EN590 |
Cold |
0.45 |
<DL |
0.27 |
189.49 |
0.45 |
36.27 |
7.25 |
1.79 × 101 |
Warm |
0.37 |
<DL |
0.26 |
33.96 |
0.37 |
26.87 |
3.36 |
2.45 × 101 |
HVO |
Cold |
0.52 |
<DL |
0.31 |
132.58 |
1.03 |
30.44 |
3.10 |
3.06 × 101 |
Warm |
0.53 |
<DL |
0.37 |
56.42 |
0.53 |
35.86 |
3.69 |
2.73 × 101 |
DPF regen. |
HVO |
Cold |
1.54 |
0.01 |
0.00 |
508.11 |
21.09 |
42.69 |
3.60 |
2.72 × 103 |
CNG |
CNG |
Cold |
3.02 |
<DL |
2.52 |
142.92 |
0.00 |
6.04 |
158.02 |
1.1 × 103 |
Warm |
2.04 |
<DL |
1.73 |
153.80 |
0.00 |
4.07 |
152.78 |
9.13 × 102 |
3.2 Toxicological results
An increase in genotoxicity was observed due to exhaust emissions exposures of EN228 gasoline with both temperatures, alkylate in the cold temperature, EN590 diesel in the cold temperature, CNG in the cold temperature, and DPF regeneration event (Fig. 2). The highest genotoxicity was measured in DPF regeneration exposure and the lowest in HVO diesel at normal temperature. GPF gasoline resulted in lower genotoxicity than a gasoline vehicle without a GPF at both temperatures. The fuel aromatic content increased genotoxicity in both gasoline and diesel vehicle exhaust exposures. A statistically significant increase compared to the clean air control was found in EN228 cold, alkylate cold, and DPF regeneration exposures. Interestingly, HVO warm exposure resulted in a significant decrease compared to the clean air control.
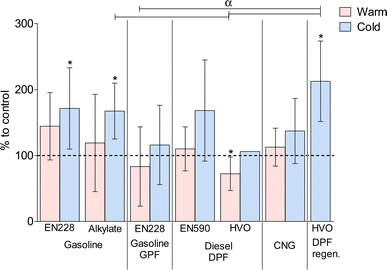 |
| Fig. 2 Percent of genotoxicity after the different vehicle exhaust exposures in two temperatures from an A549 and THP-1 co-culture, compared to the clean air control with a dashed line indicating the control. Results are shown as means with 95% CI. n = 3. *p-value < 0.05 compared to control and α = p-value < 0.05 compared between different exposures. | |
Due to different exhaust exposures, there were distinct concentrations of cytokines CXCL1 and IL-6 secreted from the cell co-cultures (Fig. 3). Decreases in CXCL1 levels were observed in EN228 gasoline at both temperatures and DPF regeneration. For IL-6, cytokine levels were decreased in exposures to gasoline EN228 at both temperatures, EN590 diesel at the cold temperature, and CNG at the warm temperature. Increased cytokine levels were observed for CXCL1 in exposures of alkylate gasoline at the warm temperature and CNG at the cold temperature. For IL-6, an increase in cytokine levels was observed from exhaust exposures of alkylate gasoline at both temperatures and CNG at the cold temperature. A statistically significant decrease compared to the clean air control was found with CXCL1 in exposures of EN228 warm and cold, and DPF regeneration. Alkylate and EN228 exhaust exposures also had statistically significant differences between the warm temperatures. For IL-6, a statistically significant decrease compared to the clean air control was observed with both warm and cold EN228 exhaust, EN590 cold, and CNG warm. A statistically significant increase compared to the clean air control was observed in alkylate warm exhaust exposure. Alkylate warm exhaust exposures displayed a statistically significant difference between both EN228 and EN590 cold, and CNG warm exhaust exposures.
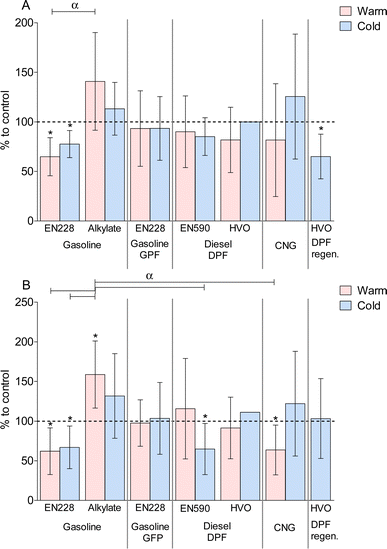 |
| Fig. 3 Percent of CXCL1 (A) and IL-6 (B) cytokines after the different vehicle exhaust exposures at two temperatures from an A549 and THP-1 co-culture, compared to the clean air control with a dashed line indicating the control. Results are shown as means with 95% CI. n = 3. *p-value < 0.05 compared to the control and α = p-value < 0.05 compared between different exposures. | |
3.3 Results from statistical analyses
The MMM analysis revealed a statistically significant increase of PM mass in genotoxicity when other measured parameters, such as NOx and NO2, were added to the model and data was divided by the vehicle types (Table 3). However, NO2 resulted in a higher increase in genotoxicity with a similar mass unit increase. Similarly, for PN a statistically significant increase in genotoxicity per unit increase was seen. For cytokines CXCL1 and IL-6, statistically significant effects were found with BC, PM, and organic matter masses, with a unit increase of PM and organic mass raising the cytokine levels and BC mass decreasing. Moreover, both PN and NOx displayed decreasing effects for both cytokine percentages. When data were further divided by the DPF regeneration effect, the association of NO2 and DPF regeneration event was emphasised in genotoxicity. The unit increases in NOx and PM mass together with DPF regeneration still induced a statistically significant increase in genotoxicity. For cytokines, no statistically significant effect was found when the DPF regeneration event was taken into account. Full results of the MMM analysis are displayed in ESI S2.†
Table 3 Results from the MMM analysis. PM, NOx, organic matter (Org), and NO2 correspond to one mass unit increase (μg m−3) and PN an increase of 10 # cm−3. Estimates indicate the percentage effect on toxicological responses and a p-value of <0.05 is considered statistically significant and marked with an asterisk
Data divided by |
Toxicological endpoint |
Parameter |
Estimate |
Std. error |
p-Value |
95% CI lower |
95% CI upper |
Vehicle |
Genotoxicity |
PM |
2.72 |
0.98 |
0.006*
|
0.79 |
4.66 |
NOx |
0.16 |
0.04 |
0.000*
|
0.08 |
0.23 |
PM |
2.52 |
0.98 |
0.012*
|
0.56 |
4.47 |
NO2 |
2.99 |
0.88 |
0.001*
|
1.22 |
4.71 |
PN |
0.02 |
0.01 |
0.006*
|
0.001 |
0.04 |
NOx |
0.17 |
0.04 |
0.000*
|
0.09 |
0.25 |
PM |
6.5 |
2.68 |
0.105 |
−2.7 |
15.6 |
Org |
−29.1 |
16.5 |
0.166 |
−78.5 |
20.3 |
CXCL1 |
PM |
26.2 |
8.94 |
0.004*
|
8.42 |
44.1 |
BC |
−48.2 |
15.4 |
0.003*
|
−77.9 |
−17.5 |
PM |
−3.7 |
1.94 |
0.097 |
−8.2 |
0.83 |
Org |
19.8 |
12.0 |
0.131 |
−0.12 |
0.02 |
IL-6 |
PM |
34.6 |
11.8 |
0.005*
|
11.0 |
58.2 |
BC |
−64.6 |
20.3 |
0.002*
|
−105.3 |
−23.9 |
BC |
−33.3 |
8.83 |
0.000*
|
−50.9 |
−15.6 |
Org |
95.2 |
29.2 |
0.002*
|
36.9 |
153.5 |
Vehicle + DPF reg. |
Genotoxicity |
PM |
1.25 |
4.73 |
0.792 |
−8.1 |
10.6 |
PN |
0.001 |
0.04 |
0.793 |
−0.06 |
0.08 |
DPF reg. |
93.8 |
28.2 |
0.001*
|
36.6 |
148.1 |
PM |
2.8 |
0.98 |
0.005*
|
0.87 |
4.78 |
NOx |
0.23 |
0.11 |
0.023*
|
0.0.4 |
0.48 |
DPF reg. |
−65.7 |
73.9 |
0.375 |
−211.6 |
80.1 |
PM |
2.3 |
1.06 |
0.028*
|
0.25 |
4.4 |
NO2 |
−5.8 |
18.2 |
0.750 |
−41.8 |
30.1 |
DPF reg. |
270.7 |
560.7 |
0.630 |
−836.3 |
1377 |
CXCL1 |
PM |
4.18 |
3.6 |
0.241 |
−2.84 |
11.2 |
PN |
−0.04 |
0.03 |
0.164 |
−0.09 |
0.02 |
DPF reg. |
−35.4 |
22.8 |
0.123 |
−80.4 |
9.67 |
IL-6 |
PM |
4.92 |
4.4 |
0.266 |
−3.8 |
13.6 |
PN |
−0.05 |
0.04 |
0.132 |
−0.12 |
0.02 |
DPF reg. |
−1.12 |
29.1 |
0.969 |
−58.6 |
56.3 |
PM |
−1.37 |
0.87 |
0.135 |
−3.22 |
0.5 |
NOx |
−0.19 |
0.09 |
0.052 |
−0.4 |
0.002 |
DPF reg. |
118.8 |
64.9 |
0.069 |
−9.3 |
246.9 |
4 Discussion
In the present study, an in vitro co-culture of A549 and THP-1 cells was exposed to the exhaust emissions of different light-duty vehicles using a thermophoresis-based ALI system. Concerning the fuel, for diesel fuels the main distinctions were in cetane number and aromatic content, and for gasoline fuels, distinctions were observed in addition to aromatic content, sulphur, and olefin content. Previous studies have linked the aromatic content to the increase in emissions, whereas the role of olefins for example is negligible.21,23,45,46,63 Therefore, the difference in the emissions between the fuels is probably due to the aromatic content. Differences in the emissions are analysed in more detail in the study of Saarikoski et al., 2023.36 But briefly, with both diesel and gasoline fuels, the higher aromatic hydrocarbon content increased the exhaust emissions. The difference was especially high within the gasoline fuels at cold temperatures, with close to a five-fold difference between the EN228 and alkylate in PM, BC, organic matter, and PN concentrations. There were also higher emissions from aromatic fuel for the diesel vehicle, which was seen as an increase in PN and PM mass, but only in the EN590 at cold operating temperature. Similarly to the present study, the aromatic content of the fuel has been associated with higher exhaust emissions in previous studies.21–24,47 The aromatic hydrocarbons in fuel work as precursors of PM emissions due to the polymerisation, higher double-bond equivalent values, higher boiling point and lower vapour pressure.48–50 In addition to PM concentrations, in our previous study by Hakkarainen et al., 2023 (ref. 24) there was a substantial increase in PM-bound PAH components from aromatic diesel fuel exhaust compared to the non-aromatic. The increase in exhaust emissions is a collective consequence of imperfect fuel mixing and a decrease in combustion efficiency.
Cold operation temperatures have been associated with higher emissions in multiple studies previously.12,25,26,51,52 Similar results were observed in the present study, with all the vehicles having a higher concentration of PM emissions in cold operation temperature measurements. Additionally, for the diesel vehicle, there were observed significant increases in NOx concentrations at cold running temperatures, indicating the effect of temperature on the increase in gaseous emissions such as NOx. The reason for emissions increases is connected to poor fuel atomisation, decreased thermal efficiency, and incomplete combustion due to cold temperature.53 For example, the increased PM formation in the cold engine is associated with the cooling effect of engine walls in a combination of inadequate evaporation of fuel droplets and poor fuel and gas mixtures.54 Furthermore, PM size distribution can be affected by colder temperatures, with increased emissions of larger particles, speculated to be connected to the evaporation of oil droplets, leading to more incomplete combustion and a higher size fraction of PM emissions. The concentration of toxic air pollution components, such as PAH compounds, also increases due to cold operating temperatures.52 Moreover, cold operation temperatures have been shown to affect the after-treatment systems, decreasing their efficiency, and leading for example to increases in NOx emissions.25,55 The increase in NOx emissions from the diesel vehicle might be due to the decreased efficiency of the SCR system in the cold temperature, similarly, as has been observed in the previous studies.25
Both the higher aromatic hydrocarbon content of the fuel and cold running temperatures also affected the toxicity of the emissions. Genotoxicity was higher in all the cold temperature exposures except the gasoline vehicle fuelled with EN228, indicating that overall cold temperature increases the genotoxicity of the emissions. The increase in genotoxicity and increase in PM emissions are associated, as the exhaust PM has been connected to genotoxicity in previous studies.24,56,57 The MMM analysis also demonstrated a statistically significant increase in genotoxicity due to the PN and PM mass concentration increases, supporting the genotoxicity of exhaust PM. Gaseous components such as NOx and NO2 also displayed a statistically significant increase in genotoxicity, highlighting the potential toxicity of these as well. Similarly, the aromatic content of the fuel has been shown to increase the toxicological responses to the exhaust PM in several studies.58–63 Moreover, the previous study by Hakkarainen et al., 2023 (ref. 24) shows that higher concentrations of PM-bound PAH compounds resulted in an increase in the toxicological potential of diesel exhaust. This could play a role in the genotoxicity as the PM-bound PAH compounds can be transported to intracellular spaces, increasing their toxicological potential. The ability of PAH compounds to form DNA adducts affects the DNA's structural integrity, resulting in unfunctional DNA, genotoxicity, and apoptosis.57 This could also explain the difference between as aromatic and non-aromatic gasoline exposures; the exhaust from higher aromatic fuel resulted in primary genotoxicity with an absence of inflammation, whereas the observed genotoxicity from alkylate gasoline exhaust exposures was secondary, including the inflammatory response.64 The effect of after-treatment systems of modern light-duty vehicles has been shown to significantly decrease the PAH compounds in exhaust PM, subsequently affecting the toxicity.12 In the present study, in addition to the clear effect of the DPF, the GPF was also shown to decrease the toxicity of exhaust exposures, compared to the gasoline vehicle without a GPF. These results support the recommendations to increasingly include a GPF in modern gasoline vehicles.65
Cytokine results display an increase in IL-6 levels due to exhaust exposures of a GPF-equipped gasoline vehicle in the cold operating temperature, indicating an inflammatory response from the cells. The CNG exhaust also increased both cytokine levels in exhaust exposure at cold running temperatures. Both results suggest that the cold temperature affects exhaust toxicity with low PM concentrations, indicating the important role of cold temperature in the toxicity of gaseous compounds. Interestingly, the cold temperature increased the levels of IL-6 in EN228 gasoline exhaust exposures, whereas with the diesel exhaust there was a decrease in cytokine levels at cold running temperature from exposures to higher aromatic fuel. The decrease in cytokine levels observed from the EN228 exhaust supports that the increased PM emissions also induce toxicity via cytokine level alterations. The MMM analysis also showed that higher BC concentration in emissions leads to a decrease in cytokine levels. This is likely due to the high BC concentrations in emissions of the gasoline vehicle without a GPF. Moreover, the toxicity of BC has been estimated to be higher than the mass concentration in the PM mixture, due to the small particle size and increased surface area for toxic compounds to absorb.66 The decrease in cytokine levels indicates most likely immunological responses, such as immunosuppression, likewise observed in our previous study with diesel exhaust exposures.24 Immunosuppressive effects have been associated with air pollution exposure previously.41,42,67
Interestingly, the cold temperature had an effect on the toxicological responses of CNG vehicle exhaust, with increases in genotoxicity and inflammation. The vehicle used in the present study utilises gasoline in specific moments, such as cold operating temperatures and engine start.68 Therefore, this results in a surge in PM emissions and a subsequent increase in toxicity might be due to the cold temperature resulting in increased gasoline combustion in the vehicle. The lubrication oils may also play a role in the increase in PM emissions as indicated previously.69 Overall, emissions from CNG-fuelled vehicles have also been shown previously to be less toxic compared to diesel and gasoline vehicles.70–72 However, in the vehicle type used in the present study, the cold operation temperature increased the toxicity of the exhaust, especially the genotoxicity, emphasising the need to study the effects of operating temperature on gas-fuelled vehicles further. Moreover, the cold start in the cycle produces high emissions and its share in the observed effects is probably significant.
DPF regeneration is a method of removing the accumulated PM from the filter material.73 The present study demonstrated an apparent effect in the emissions and the toxicological endpoints with the active DPF regeneration event. During the active regeneration, the accumulated soot particulates are burned from the filter by adding excess fuel in the exhaust gas system, increasing the temperature to 600 °C, followed by a multi-fold increase in PM emissions. The active regeneration event has been calculated to increase the overall particulate emissions from modern diesel vehicles and may lead to an underestimation of real-world PM emissions.73 DPF regeneration events have also been associated with an increase in sulphur emissions,74 , as seen in the results of Saarikoski et al., 2023.36 Moreover, the recent light-duty vehicle emissions regulations do not include the emissions spikes during filter regeneration.75 In the present study, a substantial increase in genotoxicity was seen from the exhaust exposures including the active regeneration of the DPF, indicating the genotoxic potential of diesel particulate emissions. In addition to the rise in PM emissions, gaseous compounds such as oxidative NO2 may play an important role in the increase of toxicity and NO2 has been suggested to be one of the main toxic components of modern diesel exhaust.76 Therefore, the increase in toxicological potential with DPF regeneration event exposure might be due to the NO2 emissions. The MMM analysis further emphasised the association of NO2 emissions and the DPF regeneration event, indicating the genotoxicity due to NO2. Consequently, the results of the present study indicate that the DPF regeneration event increases the toxicity of modern light-duty diesel vehicle emissions, implying that after-treatment events such as the DPF regeneration should be included in the discussions regarding the real-life emissions of modern vehicles. In addition, GPF regeneration events have also been associated with an increase in especially UFP emissions.77 However, the possible effect on emission toxicity requires further studies.
The utilisation of non-aromatic fuels in traffic is a possible method to decrease the hazardous emissions from the energy sector, especially when the non-aromatic fuels are made from more sustainable source materials, as the non-aromatic diesel in the present study. The non-aromatic gasoline fuel is made by alkylation, in which the lighter hydrocarbons are combined to form longer high-octane-rate gasoline using a catalyst.78 The alkylate components in the non-aromatic gasoline also have high-octane numbers.79 HVO is manufactured from renewable and sustained sources of fats and oils, resulting in diesel fuel free from oxygen, sulphur, and aromatic compounds.80 Therefore, the production of carbon-neutral biofuels would also result in the reduction of the aromatic content in fuels. Thus, increasing the availability of these fuels in addition to a better after-treatment system could be an efficient way to decrease emissions further. Moreover, as one of the main PM components of the exhaust is black carbon, which is also a relatively significant climate force, especially in the Arctic region, the potential of distinct filter systems and fuels to decrease BC emissions would further contribute to climate change mitigation. The study provides more representative in vitro results from the possible health effects of traffic emissions, as the ALI systems represent biological systems better than more traditional submerged exposures. The additional advantage of the distinct ALI systems is to include the whole aerosol within the exposure, including the gaseous compounds.81,82
The present study displayed novel results using a unique ALI exposure system. Despite this, the study had some limitations. These include a low number of exposure repetitions, which decreased the statistical power, especially with the non-aromatic diesel fuel at cold temperatures, as the DPF regeneration event took place during the two exposure cycles. Moreover, the bi-fuel approach of the CNG vehicle raises uncertainty if the higher toxicological responses with the cold running temperature were mainly because of the use of gasoline fuel in the vehicle. Furthermore, the toxicological analysis battery was a bit restricted, as the measurements were conducted at the dynamometer laboratory several hundreds of km from the toxicological laboratory. Consequently, the toxicological analyses were limited to those we could conduct from the collected medium and cell samples. Despite these limitations, the present study demonstrates how fuel components such as aromatics affect exhaust emissions toxicity in vitro and how environmental factors such as cold operating temperature have an important role. Moreover, after-treatment systems such as the DPF and GPF significantly decrease the toxicity of the emissions, but active filter regeneration events should be increasingly included in the upcoming emissions regulations.
5 Conclusions
The present study utilised a thermophoresis-based ALI system to analyse the exhaust toxicity of different light-duty vehicles and how the difference in fuel aromatic content, after-treatment systems, and environmental factors, such as operation temperature, impacts the toxicity of the emissions. Results indicate that the fuel aromatics increased the emissions, leading to an increase in toxicological responses, especially with gasoline. The after-treatment systems resulted in a substantial decrease in PM emissions, subsequently leading to a decreased toxic effect compared to the gasoline vehicle without particulate filters. The role of increased PM and NOx concentrations in genotoxicity was further accentuated with statistical analysis. The cold temperature increased the inflammatory response in multiple exhaust exposures, especially with the CNG-fuelled vehicle. However, with the CNG vehicle, this might be due to the vehicle using gasoline at cold temperatures, thus increasing the emissions and their toxicity. The active regeneration event of the DPF in diesel vehicles displayed a unique situation in the toxicological study. Regeneration events led to a substantial increase in emissions especially with NO2, subsequently observed as a rise in toxicity of emissions. Overall, the present study demonstrates how the aromatic content of the fuel increases toxicity, further emphasising our results from the previous studies. Furthermore, decreasing PM emissions from modern gasoline vehicles is highly important and will also decrease the toxicity of the exhaust emissions. Factors such as cold operating temperature and filter regeneration events should be included in the upcoming light-duty vehicle regulations to establish increased approximations of real-life exhaust emissions, resulting in further decreases in exhaust emissions and cleaning the traffic sector.
Abbreviations
A549 | Human lung type II epithelial cells |
ALI | Air–liquid interface |
BC | Black carbon |
CNG | Compressed natural gas |
CPC | Condensation particle counter |
DPF | Diesel particulate filter |
DMEM | Dulbecco's modified Eagle's medium |
DR | Dilution ratio |
ELISA | Enzyme-linked immunosorbent assay |
ELPI | Electrical low-pressure impactor |
FBS | Fetal bovine serum |
GPF | Gasoline particulate filter |
HEPES | 4-(2-Hydroxyethyl)-1-piperazineethanesulfonic acid |
PAH | Polyaromatic hydrocarbons |
PM | Particulate matter |
PMA | Phorbol-12-myristate-13-acetate |
PN | Particle number |
SP-AMS | Soot particle aerosol mass spectrometer |
SMPS | Scanning mobility particle sizer |
THP-1 | Human monocyte |
UFP | Ultra-fine particles |
Author contributions
All authors contributed to writing the manuscript and approved the present version. Performed experimental work: Henri Hakkarainen, Anssi Järvinen, Teemu Lepistö, Niina Kuittinen, Lassi Markkula, Tuukka Ihantola, Mo Yang, Maria-Viola Martikainen, Sanna Saarikoski, Minna Aurela, Luis Barreira, Pasi Jalava. Evaluated data: Henri Hakkarainen, Anssi Järvinen, Teemu Lepistö, Niina Kuittinen, Lassi Markkula, Mo Yang, Maria-Viola Martikainen, Santtu Mikkonen, Sanna Saarikoski, Minna Aurela, Luis Barreira, Mika Ihalainen, Hilkka Timonen, Topi Rönkkö, Päivi-Aakko-Saksa, Pasi Jalava. Provided experimental and instrumental infrastructure: Sanna Saarikoski, Hilkka Timonen, Topi Rönkkö, Päivi-Aakko-Saksa, Pasi Jalava. Wrote and edited the manuscript: Henri Hakkarainen, Anssi Järvinen, Teemu Lepistö, Niina Kuittinen, Lassi Markkula, Tuukka Ihantola, Mo Yang, Maria-Viola Martikainen, Santtu Mikkonen, Sanna Saarikoski, Minna Aurela, Luis Barreira, Mika Ihalainen, Hilkka Timonen, Topi Rönkkö, Päivi-Aakko-Saksa, Pasi Jalava.
Conflicts of interest
The authors declare that they have no known competing financial interests or personal relationships that could have appeared to influence the work reported in this paper.
Acknowledgements
This project has received funding from the European Union's Horizon 2020 research and innovation programme under grant agreements no. 814978 (TUBE) and no. 955390 (ULTRHAS). Financial support was provided by the Black Carbon Footprint project funded by Business Finland (grant no. 528/31/2019, 530/31/2019), participating companies, and municipal actors. The Academy of Finland Flagship Programme “ACCC” (grant numbers 337551, 337552) as well as University of Eastern Finland doctoral school EPHB funding are gratefully acknowledged. We thank Hanne Vainikainen for her invaluable assistance with the Comet analysis and Petteri Marjanen, Jussi Hoivala, and Iida Okkonen for their valuable work during the measurement campaign.
References
- S. Heydari, M. Tainio, J. Woodcock and A. de Nazelle, Estimating traffic contribution to particulate matter concentration in urban areas using a multilevel Bayesian meta-regression approach, Environ. Int., 2020, 141, 105800, DOI:10.1016/j.envint.2020.105800.
-
European Environment Agency (EEA), Transport and Environment Report 2021 Decarbonising Road Transport — the Role of Vehicles, Fuels and Transport Demand, 2022, p. 87, DOI:10.2800/68902.
-
EPA, Inventory of U.S. Greenhouse Gas Emissions and Sinks: 1990-2021, U.S. Environmental Protection Agency, 2023, EPA 430-D-23-001, https://www.epa.gov/ghgemissions/draft-inventory-us-greenhouse-gas-emissions-and-sinks-1990-2021 Search PubMed.
-
World Health Organization, WHO Global Air Quality Guidelines: Particulate Matter (PM2.5 and PM10), Ozone, Nitrogen Dioxide, Sulfur Dioxide and Carbon Monoxide, World Health Organization, 2021, https://apps.who.int/iris/handle/10665/345329 Search PubMed.
-
ICCT, International Council on Clean Transportation, China, 2020, accessed August 19, 2020, https://theicct.org/china.
-
ICCT, International Council on Clean Transportation, Latin America, 2020, accessed August 19, 2020, https://theicct.org/latin-america.
-
ICCT, International Council on Clean Transportation, United States, 2020, accessed August 19, 2020, https://theicct.org/united-states.
- N. Hooftman, M. Messagie, J. Van Mierlo and T. Coosemans, A review of the European passenger car regulations – real driving emissions vs local air quality, Renewable Sustainable Energy Rev., 2018, 86, 1–21, DOI:10.1016/j.rser.2018.01.012.
- Y. Wu, S. Zhang, J. Hao, H. Liu, X. Wu, J. Hu, M. P. Walsh, T. J. Wallington, K. M. Zhang and S. Stevanovic, On-road vehicle emissions and their control in China: A review and outlook, Sci. Total Environ., 2017, 574, 332–349, DOI:10.1016/j.scitotenv.2016.09.040.
- P. Bielaczyc, IOP Conf. Ser.: Earth Environ. Sci., 2019, 214, 012139 CrossRef.
- C. B. Ribeiro, F. H. C. Rodella and L. Hoinaski, Regulating light-duty vehicle emissions: an overview of US, EU, China and Brazil programs and its effect on air quality, Clean Technol. Environ. Policy, 2022, 24(3), 851–862, DOI:10.1007/s10098-021-02238-1.
- H. Hakkarainen, P. Aakko-Saksa, M. Sainio, T. Ihantola, T. J. Rönkkö, P. Koponen, T. Rönkkö and P. I. Jalava, Toxicological evaluation of exhaust emissions from light-duty vehicles using different fuel alternatives in sub-freezing conditions, Part. Fibre Toxicol., 2020, 17, 17 CrossRef CAS PubMed.
- A. Kęska, A. Janicka, M. Zawiślak, J. Molska, R. Włostowski, A. Włóka, J. Świeściak and K. Ostrowski, Assessment of the Actual Toxicity of Engine Exhaust Gas Emissions from Euro 3 and Euro 6 Compliant Vehicles with the BAT-CELL Method Using In vitro Tests, Int. J. Environ. Res. Publ. Health, 2022, 19(21), 14138, DOI:10.3390/ijerph192114138.
- D. Sofia, F. Gioiella and N. Lotrecchiano,
et al., Mitigation strategies for reducing air pollution, Environ. Sci. Pollut. Res., 2020, 27, 19226–19235, DOI:10.1007/s11356-020-08647-x.
- B. Apicella, E. Mancaruso, C. Russo, A. Tregrossi, M. M. Oliano, A. Ciajolo and B. M. Vaglieco, Effect of after-treatment systems on particulate matter emissions in diesel engine exhaust, Exp. Therm. Fluid Sci., 2020, 116, 110107 CrossRef CAS.
- E. Kritsanaviparkporn, F. M. Baena-Moreno and T. R. Reina, Catalytic Converters for Vehicle Exhaust: Fundamental Aspects and Technology Overview for Newcomers to the Field, Chemistry, 2021, 3(2), 630–646, DOI:10.3390/chemistry3020044.
- H. S. Kwon, M. H. Ryu and C. Carlsten, Ultrafine particles: unique physicochemical properties relevant to health and disease, Exp. Mol. Med., 2020, 52, 318–328, DOI:10.1038/s12276-020-0405-1.
-
M. Amann, M. Holland, R. Maas, T. Vandyck and B. Saveyn, Costs, Benefits and Economic Impacts of the EU Clean Air Strategy and Their Implications on Innovation and Competitiveness, International Institute for Applied Systems Analysis, Laxenburg, 2017, https://ec.europa.eu/environment/air/pdf/clean_air_outlook_economic_impact_report.pdf Search PubMed.
- H. Timonen, P. Karjalainen, E. Saukko, S. Saarikoski, P. Aakko-Saksa, P. Simonen, T. Murtonen, M. Dal Maso, H. Kuuluvainen, M. Bloss, E. Ahlberg, B. Svenningsson, J. Pagels, W. H. Brune, J. Keskinen, D. R. Worsnop, R. Hillamo and T. Rönkkö, Influence of fuel ethanol content on primary emissions and secondary aerosol formation potential for a modern flex-fuel gasoline vehicle, Atmos. Chem. Phys., 2017, 17, 5311–5329 CrossRef CAS.
- J. Yang, P. Roth, T. Durbin, C. J. Kent, A. Asa-Awuku, D. Cocker and G. Karavalakis, Investigation of the effect of mid- and high-level ethanol blends on the particulate and the mobile source air toxic (MSAT) emissions from a GDI flex fuel vehicle, Energy Fuels, 2019, 33, 429–440 CrossRef CAS.
- G. Karavalakis, D. Short, D. Vu, R. Russell, M. Hajbabaei, A. Asa-Awuku and T. D. Durbin, Evaluating the effects of aromatics content in gasoline on gaseous and particulate matter emissions from SI-PFI and SIDI vehicles, Environ. Sci. Technol., 2015, 49, 7021–7031, DOI:10.1021/es5061726.
- J. Peng, M. Hu, Z. Du, Y. Wang, J. Zheng, W. Zhang, Y. Yang, Y. Qin, R. Zheng, Y. Xiao, Y. Wu, S. Lu, Z. Wu, S. Guo, H. Mao and S. Shuai, Gasoline aromatics: A critical determinant of urban secondary organic aerosol formation, Atmos. Chem. Phys., 2017, 17(17), 10743–10752, DOI:10.5194/acp-17-10743-2017.
- J. Yang, P. Roth, T. Durbin and G. Karavalakis, Impacts of gasoline aromatic and ethanol levels on the emissions from GDI vehicles: Part 1. Influence on regulated and gaseous toxic pollutants, Fuel, 2019, 252, 799–811, DOI:10.1016/j.fuel.2019.04.143.
- H. Hakkarainen, A. Järvinen, T. Lepistö, L. Salo, N. Kuittinen, E. Laakkonen, M. Yang, M.-V. Martikainen, S. Saarikoski, M. Aurela, L. Barreira, K. Teinilä, M. Ihalainen, P. Aakko-Saksa, H. Timonen, T. Rönkkö and P. I. Jalava, Toxicity of exhaust emissions from high aromatic and non-aromatic diesel fuels using in vitro ALI exposure system, Sci. Total Environ., 2023, 890, 164215 CrossRef CAS.
- R. Suarez-Bertoa and C. Astorga, Impact of cold temperature on euro 6 passenger car emissions, Environ. Pollut., 2018, 234, 318–329, DOI:10.1016/j.envpol.2017.10.096.
- C. Weber, I. Sundvor and E. Figenbaum, Comparison of regulated emission factors of Euro 6 LDV in Nordic temperatures and cold start conditions: Diesel- and gasoline direct-injection, Atmos. Environ., 2019, 206, 208–217, DOI:10.1016/j.atmosenv.2019.02.031.
- M. Park, H. S. Joo and K. Lee,
et al., Differential toxicities of fine particulate matters from various sources, Sci. Rep., 2018, 8, 17007 CrossRef PubMed.
- J. J. West, A. Cohen, F. Dentener, B. Brunekreef, T. Zhu, B. Armstrong, M. L. Bell, M. Brauer, G. Carmichael and D. L. Costa,
et al., What We Breathe Impacts Our Health: Improving Understanding of the Link between Air Pollution and Health, Environ. Sci. Technol., 2016, 50, 4895–4904, DOI:10.1021/acs.est.5b03827.
- M. Ihalainen, P. Jalava, T. Ihantola, S. Kasurinen, O. Uski, O. Sippula, A. Hartikainen, J. Tissari, K. Kuuspalo, A. Lähde, M.-R. Hirvonen and J. Jokiniemi, Design and validation of an air-liquid interface (ALI) exposure device based on thermophoresis, Aerosol Sci. Technol., 2019, 53(2), 133–145, DOI:10.1080/02786826.2018.1556775.
- K. Kaur, R. Mohammadpour, A. Sturrock, H. Ghandehari, C. Reilly, R. Paine III and K. E. Kelly, Comparison of biological responses between submerged, pseudo-air-liquid interface, and air-liquid interface exposure of A549 and differentiated THP-1 co-cultures to combustion-derived particles, J. Environ. Sci. Health, Part A, 2022, 57(7), 540–551, DOI:10.1080/10934529.2022.2083429.
- A. M. Speen, J. R. Murray, Q. T. Krantz, D. Davies, P. Evansky, J. A. Harrill, L. J. Everett, J. L. Bundy, L. A. Dailey and J. Hill,
et al., Benchmark Dose Modeling Approaches for Volatile Organic Chemicals Using a Novel Air-Liquid Interface In vitro Exposure System, Toxicol. Sci., 2022, 188, 88–107 CrossRef CAS PubMed.
- S. Binder, N. Rastak, E. Karg, A. Huber, E. Kuhn, G. C. Dragan, C. Monsé, D. Breuer, S. Di Bucchianico, M. N. Delaval, S. Oeder, M. Sklorz and R. Zimmermann, Construction of an In vitro Air–Liquid Interface Exposure System to Assess the Toxicological Impact of Gas and Particle Phase of Semi-Volatile Organic Compounds, Toxics, 2022, 10(12), 730, DOI:10.3390/toxics10120730.
- R. Bedford, E. Perkins, J. Clements and M. Hollings, Recent advancements and application of in vitro models for predicting inhalation toxicity in humans, Toxicol. in Vitro, 2022, 79, 105299, DOI:10.1016/j.tiv.2021.105299.
- M. Shiraiwa, K. Ueda, A. Pozzer, G. Lammel, C. J. Kampf, A. Fushimi, S. Enami, A. M. Arangio, J. Fröhlich-Nowoisky, Y. Fujitani, A. Furuyama, P. S. J. Lakey, J. Lelieveld, K. Lucas, Y. Morino, U. Pöschl, S. Takahama, A. Takami, H. Tong, B. Weber, A. Yoshino and K. Sato, Aerosol Health Effects from Molecular to Global Scales, Environ. Sci. Technol., 2017, 51(23), 13545–13567, DOI:10.1021/acs.est.7b04417.
- S. M. Platt, I. El Haddad, S. M. Pieber, A. A. Zardini, R. Suarez-Bertoa, M. Clairotte and A. S. H. Prévôt, Gasoline cars produce more carbonaceous particulate matter than modern filter-equipped diesel cars, Sci. Rep., 2017, 7(1), 4926–4929, DOI:10.1038/s41598-017-03714-9.
- S. Saarikoski, A. Järvinen, L. Markkula, M. Aurela, N. Kuittinen, J. Hoivala, L. M. F. Barreira, P. Aakko-Saksa, T. Lepistö, P. Marjanen, H. Timonen, H. Hakkarainen, P. Jalava and T. Rönkkö, Towards zero pollution vehicles by advanced fuels and exhaust after treatment technologies, Environ. Pollut., 2024, 347, 123665 CrossRef CAS PubMed.
- T. Rönkkö, A. Virtanen, K. Vaaraslahti, J. Keskinen, L. Pirjola and M. Lappi, Effect of dilution conditions and driving parameters on nucleation mode particles in diesel exhaust: laboratory and on-road study, Atmos. Environ., 2006, 40, 2893–2901 CrossRef.
- J. Keskinen and T. Rönkkö, Can Real-World Diesel Exhaust Particle Size Distribution be Reproduced in the Laboratory? A Critical Review, J. Air Waste Manag. Assoc., 2010, 60, 1245–1255 CrossRef CAS PubMed.
- L. Drinovec, G. Močnik, P. Zotter, A. S. H. Prévôt, C. Ruckstuhl, E. Coz, M. Rupakheti, J. Sciare, T. Müller, A. Wiedensohler and A. D. A. Hansen, The “dual-spot” Aethalometer: an improved measurement of aerosol black carbon with real-time loading compensation, Atmos. Meas. Tech., 2015, 8, 1965–1979, DOI:10.5194/amt-8-1965-2015.
- H. Hakkarainen, L. Salo, S. Mikkonen, S. Saarikoski, M. Aurela, K. Teinilä, M. Ihalainen, S. Martikainen, P. Marjanen, T. Lepistö, N. Kuittinen, K. Saarnio, P. Aakko-Saksa, T. V. Pfeiffer, H. Timonen, T. Rönkkö and P. I. Jalava, Black carbon toxicity dependence on particle coating: Measurements with a novel cell exposure method, Sci. Total Environ., 2022, 838(4), 156543, DOI:10.1016/j.scitotenv.2022.156543.
- T. Ihantola, M. R. Hirvonen and M. Mika Ihalainen,
et al., Genotoxic and inflammatory effects of spruce and brown coal briquettes combustion aerosols on lung cells at the air-liquid interface, Sci. Total Environ., 2022, 806(1), 150489, DOI:10.1016/j.scitotenv.2021.150489.
- T. Ihantola, S. Di Bucchianico and M. Happo,
et al., Influence of wood species on toxicity of log-wood stove combustion aerosols: a parallel animal and air-liquid interface cell exposure study on spruce and pine smoke, Part. Fibre Toxicol., 2020, 17, 27, DOI:10.1186/s12989-020-00355-1.
-
L. Mehtätalo and J. Lappi, Biometry for Forestry and Environmental Data: with Examples in R, CRC Press, LLC, Boca Raton, FL, 2020 Search PubMed.
- S. Mikkonen, H. Korhonen, S. Romakkaniemi, J. N. Smith, J. Joutsensaari, K. E. J. Lehtinen, A. Hamed, T. J. Breider, W. Birmili, G. Spindler, C. Plass-Duelmer, M. C. Facchini and A. Laaksonen, Meteorological and trace gas factors affecting the number concentration of atmospheric Aitken (Dp=50 nm) particles in the continental boundary layer: parameterization using a multivariate mixed effects model, Geosci. Model Dev., 2011, 4, 1–13, DOI:10.5194/gmd-4-1-2011.
- M. Hajbabaei, G. Karavalakis, J. W. Miller, M. Villela, K. Huaying Xu and T. D. Durbin, Impact of olefin content on criteria and toxic emissions from modern gasoline vehicles, Fuel, 2013, 107, 671–679, DOI:10.1016/j.fuel.2012.12.031.
- T. Lu, Z. Huang, C. S. Cheung and M. J. Jing, Size distribution of EC, OC and particle-phase PAHs emissions from a diesel engine fueled with three fuels, Sci. Total Environ., 2012, 438, 33–41, DOI:10.1016/j.scitotenv.2012.08.026.
- T. Chen, Y. Liu, C. Liu, J. Liu, B. Chu and H. He, Important role of aromatic hydrocarbons in SOA formation from unburned gasoline vapor, Atmos. Environ., 2019, 201, 101–109, DOI:10.1016/j.atmosenv.2019.01.001.
- H. Richter and J. B. Howard, Formation of polycyclic aromatic hydrocarbons and their growth to soot: a review of chemical reaction pathways, Prog. Energy Combust. Sci., 2000, 26, 565–608 CrossRef CAS.
- K. Aikawa and J. J. Jetter, Impact of gasoline composition on particulate matter emissions from a direct-injection gasoline engine: Applicability of the particulate matter index, Int. J. Engine Res., 2014, 15(3), 298–306, DOI:10.1177/1468087413481216.
- P. Hellier, N. Ladommatos, R. Allan and J. Rogerson, Combustion and emissions characteristics of toluene/n-heptane and 1-octene/n-octane binary mixtures in a direct injection compression ignition engine, Combust. Flame, 2013, 160(10), 2141–2158, DOI:10.1016/j.combustflame.2013.04.016.
-
P. Aakko and N. Nylund, Particle Emissions at Moderate and Cold Temperatures Using Different Fuels, SAE Technical Paper 2003-01-3285, 2003, DOI:10.4271/2003-01-3285.
- R. Zhu, Y. Fu, L. Wang, J. Hu, L. He, M. Wang, Y. Lai and S. Su, Effects of a start-stop system for gasoline direct injection vehicles on fuel consumption and particulate emissions in hot and cold environments, Environ. Pollut., 2022, 308, 119689, DOI:10.1016/j.envpol.2022.119689.
- Y. Choi, H. Yi, Y. Oh and S. Sungwook Park, Effects of engine restart strategy on particle number emissions from a hybrid electric vehicle equipped with a gasoline direct injection engine, Atmos. Environ., 2021, 253, 118359, DOI:10.1016/j.atmosenv.2021.118359.
- S. Yu, G. Dong and L. Li, Transient characteristics of emissions during engine start/stop operation employing a conventional gasoline engine for HEV application, Int. J. Automot. Technol., 2008, 9, 543–549, DOI:10.1007/s12239-008-0064-z.
- A. A. Yusuf and F. L. Inambao, Effect of cold start emissions from gasoline-fueled engines of light-duty vehicles at low and high ambient temperatures: Recent trends, Case Stud. Therm. Eng., 2019, 14, 100417, DOI:10.1016/j.csite.2019.100417.
- J. Topinka, A. Milcova, J. Schmuczerova, M. Mazac, M. Pechout and M. V. Lom, Genotoxic potential of organic extracts from particle emissions of diesel and rapeseed oil powered engines, Toxicol. Lett., 2012, 212, 11–17 CrossRef CAS PubMed.
- P. M. Yang,
et al., Development of novel alternative biodiesel fuels for reducing PM emissions and PM-related genotoxicity, Environ. Res., 2017, 156, 512–518 CrossRef CAS PubMed.
- T. Lu, Z. Huang, C. S. Cheung and J. Jing Ma, Size distribution of EC, OC and particle-phase PAHs emissions from a diesel engine fueled with three fuels, Sci. Total Environ., 2012, 438, 33–41, DOI:10.1016/j.scitotenv.2012.08.026.
- B. L. Valle-Hernández, O. Amador-Muñoz, A. Jazcilevich-Diamant, A. E. Hernández-López, R. Villalobos-Pietrini and R. González-Oropeza, Polycyclic Aromatic Hydrocarbons in Particulate Matter Emitted by the Combustion of Diesel and Biodiesel, Combust. Sci. Technol., 2013, 185(3), 420–434, DOI:10.1080/00102202.2012.726665.
- I. M. Kooter, M. J. Alblas, A. D. Jedynska, M. Steenhof, M. M. G. Houtzager and M. van Ras, Alveolar epithelial cells (A549) exposed at the air–liquid interface to diesel exhaust: First study in TNO's powertrain test center, Toxicol. in Vitro, 2013, 27(8), 2342–2349, DOI:10.1016/j.tiv.2013.10.007.
- S. Steiner, J. Czerwinski, P. Comte, O. Popovicheva, E. Kireeva, L. Müller, N. Heeb, A. Mayer, A. Fink and B. Rothen-Rutishauser, Comparison of the toxicity of diesel exhaust produced by bio- and fossil diesel combustion in human lung cells in vitro, Atmos. Environ., 2013, 81, 380–388, DOI:10.1016/j.atmosenv.2013.08.059.
- L. Malorni, V. Guida, M. Sirignano, G. Genovese, C. Petrarca and P. Pedata, Exposure to sub-10nm particles emitted from a biodiesel-fueled diesel engine: In vitro toxicity and inflammatory potential, Toxicol. Lett., 2017, 270, 51–61, DOI:10.1016/j.toxlet.2017.02.009.
- N. Yilmaz and B. Donaldson, Combined effects of engine characteristics and fuel aromatic content on polycyclic aromatic hydrocarbons and toxicity, Energy Sources, Part A, 2022, 44(4), 9156–9171, DOI:10.1080/15567036.2022.2129880.
- R. P. Schins and A. M. Knaapen, Genotoxicity of poorly soluble particles, Inhalation Toxicol., 2007, 19(Suppl 1), 189–198, DOI:10.1080/08958370701496202.
- O. I. Awad, X. Ma, M. Kamil, O. M. Ali, Z. Zhang and S. Shuai, Particulate emissions from gasoline direct injection engines: A review of how current emission regulations are being met by automobile manufacturers, Sci. Total Environ., 2020, 718, 137302 CrossRef CAS PubMed.
- N. A. Janssen, G. Hoek, M. Simic-Lawson, P. Fischer, L. van Bree, H. ten Brink, M. Keuken, R. W. Atkinson, H. R. Anderson, B. Brunekreef and F. R. Cassee, Black carbon as an additional indicator of the adverse health effects of airborne particles compared with PM10 and PM2.5, Environ. Health Perspect., 2011, 119(12), 1691–1699, DOI:10.1289/ehp.1003369.
- M.-V. Martikainen, T. J. Rönkkö and B. Schaub,
et al., Integrating farm and air pollution studies in search for immunoregulatory mechanisms operating in protective and high-risk environments, Pediatr. Allergy Immunol., 2018, 29, 815–822, DOI:10.1111/pai.12975.
- Z. Samaras, A. Kontses, A. Dimaratos and D. Kontses,
et al., A European Regulatory Perspective towards a Euro 7 Proposal, SAE Int. J. Adv. Curr. Prac. Mobil., 2023, 5(3), 998–1011, DOI:10.4271/2022-37-0032.
- A. P. Singh, A. Pal and A. K. Agarwal, Comparative particulate characteristics of hydrogen, CNG, HCNG, gasoline and diesel fueled engines, Fuel, 2016, 185(1), 491–499 CrossRef CAS.
- A. K. Agarwal, B. Ateeq, T. Gupta, A. P. Singh, S. K. Pandey, N. Sharma, R. A. Agarwal, N. K. Gupta, H. Sharma, A. Jain and P. C. Shukla, Toxicity and mutagenicity of exhaust from compressed natural gas: could this be a clean solution for megacities with mixed-traffic conditions?, Environ. Pollut., 2018, 239, 1–13 CrossRef PubMed.
- J. Seagrave, A. Gigliotti, J. D. McDonald, S. K. Seilkop, K. A. Whitney, B. Zielinska and J. L. Mauderly, Composition, toxicity, and mutagenicity of particulate and semi volatile emissions from heavy-duty compressed natural gas-powered vehicles, Toxicol. Sci., 2005, 87, 232–241 CrossRef CAS PubMed.
- L. Turio-Baldassarri, C. L. Battistelli, L. Conti, R. Crebelli, B. De Berardis, A. L. Iamiceli, M. Gambino and S. Iannaccone, Evaluation of emission toxicity of urban bus engines: compressed natural gas and comparison with liquid fuels, Sci. Total Environ., 2006, 355, 64–77 CrossRef PubMed.
- Z. Zhang, R. Dong, G. Lan, T. Yuan and D. Tan, Diesel particulate filter regeneration mechanism of modern automobile engines and methods of reducing PM emissions: A review, Environ. Sci. Pollut. Res., 2023, 30(14), 39338–39376 CrossRef CAS PubMed.
-
T. Hirano, I. Goto, K. Kitano, T. Kojima and M. Ikeda, Analysis of Sulfur-Related White Smoke Emissions from DPF System, SAE Technical Paper 2015-01-2023, 2015, DOI:10.4271/2015-01-2023.
- B. Giechaskiel and M. Clairotte, Fourier Transform Infrared (FTIR) Spectroscopy for Measurements of Vehicle Exhaust Emissions: A Review, Appl. Sci., 2021, 11(16), 7416, DOI:10.3390/app11167416.
- C. A. Weitekamp, L. B. Kerr, L. Dishaw, J. Nichols, M. Lein and M. J. Stewart, A systematic review of the health effects associated with the inhalation of particle-filtered and whole diesel exhaust, Inhal. Toxicol., 2020, 32(1), 1–13, DOI:10.1080/08958378.2020.
- T. W. Chan, M. Saffaripour and F. Liu,
et al., Characterization of Real-Time Particle Emissions from a Gasoline Direct Injection Vehicle Equipped with a Catalyzed Gasoline Particulate Filter During Filter Regeneration, Emiss. Control Sci. Technol., 2016, 2, 75–88, DOI:10.1007/s40825-016-0033-3.
- H. D. Velázquez, R. Cerón-Camacho and M. L. Mosqueira-Mondragón,
et al., Recent progress on catalyst technologies for high quality gasoline production, Catal. Rev., 2022, 1–221, DOI:10.1080/01614940.2021.2003084.
-
J. Perander, L. Rantanen, J. Pentikäinen, P. Aakko, et al., No Major Backsliding in Air Quality when Replacing MTBE with Isooctane in CARB Gasoline, SAE Technical Paper 2001-01-3588, 2001, DOI:10.4271/2001-01-3588.
- A. Dimitriadis, I. Natsios, A. Dimaratos, D. Katsaounis, Z. Samaras, S. Bezergianni and K. Lehto, Evaluation of a hydrotreated vegetable oil (HVO) and effects on emissions of a passenger car diesel engine, Front. Mech. Eng., 2018, 4, 7 CrossRef.
- S. C. Faber and S. D. McCullough, Through the Looking Glass: In vitro Models for Inhalation Toxicology and Interindividual Variability in the Airway, Appl. In Vitro Toxicol., 2018, 4(2), 115–128, DOI:10.1089/aivt.2018.0002.
- S. Upadhyay and L. Palmberg, Air-Liquid Interface: Relevant In Vitro Models for Investigating Air Pollutant-Induced Pulmonary Toxicity, Toxicol. Sci., 2018, 164(1), 21–30, DOI:10.1093/toxsci/kfy053.
|
This journal is © The Royal Society of Chemistry 2024 |
Click here to see how this site uses Cookies. View our privacy policy here.