Storms mobilize organophosphate esters, bisphenols, PFASs, and vehicle-derived contaminants to San Francisco Bay watersheds†
Received
6th March 2024
, Accepted 10th September 2024
First published on 16th September 2024
Abstract
In urban to peri-urban watersheds such as those surrounding San Francisco Bay, stormwater runoff is a major pathway by which contaminants enter aquatic ecosystems. We evaluated the occurrence of 154 organic contaminants via liquid chromatography coupled to tandem mass spectrometry, including organophosphate esters (OPEs), bisphenols, per- and polyfluoroalkyl substances (PFASs), and a suite of novel urban stormwater tracers (SWCECs; i.e., vehicle-derived chemicals, pesticides, pharmaceuticals/personal care products, benzothiazoles/benzotriazoles). Time-averaged composite sampling focused on storms in highly developed watersheds over four wet seasons, with complementary sampling in less-urban reference watersheds, near-shore estuarine sites, and the open Bay. Of the targeted contaminants, 68 (21 SWCECs, 29 OPEs, 3 bisphenols, 15 PFASs) were detected in ≥10 of 26 urban stormwater samples. Median concentrations exceeded 500 ng L−1 for 1,3-diphenylguanidine, hexa(methoxymethyl)melamine, and caffeine, and exceeded 300 ng L−1 for 2-hydroxy-benzothiazole, 5-methyl-1H-benzotriazole, pentachlorophenol, and tris(2-butoxyethyl) phosphate. Median individual PFAS concentrations were <10 ng L−1, with highest concentrations for PFHxA (180 ng L−1), PFOA (110 ng L−1), and PFOS (81 ng L−1). In six of eight urban stormwater samples analyzed for 6PPD-quinone (a tire rubber-derived transformation product), concentrations exceeded coho salmon acute toxicity thresholds, suggesting (sub)lethal impacts for sensitive species. Observed concentrations were generally significantly higher in highly developed watersheds relative to reference watersheds, but not statistically different in near-shore estuarine sites, suggesting substantial transient exposure potential at stormwater outfalls or creek outflows. Results emphasized the role of stormwater in contaminant transport, the importance of vehicles/roadways as contaminant sources, and the value of monitoring broad multi-analyte contaminant suites to enable comprehensive source and toxicity evaluations.
Environmental significance
Stormwater runoff transports complex contaminant mixtures to receiving waters. However, sampling efforts often focus on limited chemical/use classes and exclude sampling during storms (i.e., during peak mass transport). Notably, vehicle/roadway-derived contaminants have largely undefined toxicity but are globally reported in stormwater (including here) at high concentrations/mass loads. Understanding the occurrence/concentrations of diverse contaminants in stormwater is critical to effective source control and treatment. Data reported here demonstrate that urban stormwater transports significant levels of emerging contaminants to receiving waters, including many classes (e.g., OPEs, bisphenols, PFASs, pharmaceuticals) for which prior studies have focused on wastewater transport. Overall, results inform the extent and scale of future monitoring efforts in San Francisco Bay and other urbanized estuaries, enabling improved stormwater management.
|
Introduction
Stormwater runoff from urban to peri-urban landscapes is increasingly recognized as an important transport pathway for organic contaminants to receiving waters. While many “traditional” stormwater monitoring efforts have focused on metals, polychlorinated biphenyls, polycyclic aromatic hydrocarbons, or legacy pesticides, these efforts exclude the thousands of emerging organic contaminants that are widely used in industrial, commercial, and residential settings. Research that broadens the “analytical net”—by either using targeted analytical methods that include more contaminant classes or non-targeted analyses—is increasingly reporting highly complex contaminant mixtures in stormwater and roadway-impacted receiving waters.1–4
Many classes of emerging contaminants demonstrate characteristics of ubiquitous use, known or suspected toxicity, or reported occurrence in stormwater. For example, the total concentrations of organophosphate ester (OPE) flame retardants and plasticizers5 in stormwater were correlated to transportation-related land use in Hong Kong, China.6 OPEs are associated with a variety of toxic effects, including endocrine disruption and neurotoxicity, with some members of this class also suspected carcinogens.7 Bisphenols, including bisphenol A (BPA) and replacement analogues, widely used in polycarbonate plastics, exhibit endocrine disrupting effects (among other toxic impacts) and are commonly detected in the global environment.8 Per- and polyfluoroalkyl substances (PFASs) used as fire extinguishing agents and to provide water/oil resistance within many consumer products, are now detected ubiquitously in the environment, wildlife, and humans.9–11 PFASs are either highly persistent or degrade to other highly persistent PFASs, and are associated with a wide variety of toxic effects in both wildlife and humans.12 Additionally, there exist many poorly documented contaminants derived from vehicles or roadways, or specifically from tire rubbers, in environmental matrices.13 Examples include 6PPD-quinone (6PPDQ; 2-anilino-5-[(4-methylpentan-2-yl)amino]cyclohexa-2,5-diene-1,4-dione), a transformation product of the rubber antiozonant 6PPD (N-(1,3-dimethylbutyl)-N′-phenyl-p-phenylenediamine) that is acutely toxic to coho salmon,14 the rubber vulcanization accelerator 1,3-diphenylguanidine,15–17 and the corrosion inhibitor 5-methyl-1H-benzotriazole.18,19 Although these compounds have now been globally reported in stormwater and other environmental matrices, the toxicity of most such chemicals remains largely undefined.
Surrounded by a densely populated urban area, San Francisco Bay, California, USA is an important and representative location to evaluate the occurrence of organic contaminants in stormwater runoff and associated receiving waters. Apart from the City of San Francisco, which has a combined sewer system, the region is served by separated stormwater infrastructure that delivers significant legacy contaminant loads to the Bay.20 Past receiving water monitoring efforts reported 13 OPEs21 and >100 pharmaceuticals and personal care products and four alkylphenols22 in Bay water, sediment, and tissue samples. Shimabuku et al. (2022) evaluated the occurrence of and correlations between 22 OPEs and 16 bisphenols in Bay surface water and conducted a screening-level ecological risk assessment.23 Overdahl et al. (2021) applied non-targeted analysis to municipal wastewater effluent and to Bay water samples from sites influenced by different transport pathways, including municipal wastewater, agricultural runoff, and urban stormwater.1 That study documented polar organic pollutants such as pesticide transformation products, polymer additives, and rubber vulcanization agents,1 indicating the role of key and diverse contaminant transport pathways to the estuary. Lindborg et al. (2023) sampled Bay water, stormwater runoff, and municipal wastewater effluent for ethoxylated surfactants.2 However, studies to date did not include robust sampling of stormwater during storm events when the majority of contaminant mass loads are transported to receiving waters. Furthermore, most studies focused on one or two chemical type/use classes, rather than providing a more comprehensive and quantitative contaminant dataset. Understanding the occurrence and concentrations of a broad range of emerging contaminants in stormwater runoff is critical to informing effective stormwater management, source control, and treatment decisions.
To address these data gaps, we applied a comprehensive suite of targeted analytical methods based on liquid chromatography coupled to tandem mass spectrometry (LC-MS/MS) to quantify 154 analytes from four major contaminant groups: 27 polar organic contaminants of emerging concern that serve collectively as a novel stormwater tracer suite (SWCECs; including vehicle-derived chemicals, pesticides, pharmaceuticals/personal care products, and benzothiazoles/benzotriazoles), 34 OPEs, 17 bisphenols, and 76 PFASs. Sample collection primarily targeted storm events in highly urbanized watersheds using time-averaged composite samples to represent an average of an entire storm event, rather than a single snapshot within the contaminant “pollutograph.” Complementary sampling occurred in less urbanized reference watersheds, at near-shore Bay sites with tidal mixing, and in the open Bay to evaluate contaminant occurrence and to advance our understanding of fate and transport within receiving waters. The results can inform the extent and scale of future monitoring efforts (e.g., site quantity and selection criteria, prioritization of contaminants and chemical classes, etc.) in San Francisco Bay and other urbanized estuaries while increasing our understanding of the occurrence of multi-class emerging organic contaminants and enabling evaluation and management of stormwater.
Methods
Chemicals and reagents
Details are provided in Text S1 and Table S1.†
Sampling sites and events
This study primarily focused on sampling in the San Francisco Bay Area (CA, USA) during storm events over four wet seasons (Fall 2018 – Spring 2022) at urban stormwater runoff sites (n = 21 creeks, storm drains, pump stations, and outfalls; n = 10 storm events; 1–3 events per site) and less urban reference sites (n = 4 creeks; n = 4 storm events; 1 event per site) (Fig. 1). Complementary sample collection (leveraging the related Regional Monitoring Program for Water Quality in San Francisco Bay) for select contaminants also occurred (1) at the edge of the Bay within two hours after a storm event (“near-field,” estuarine samples representing tidal mixing with stormwater runoff; n = 3 sites; 1 sampling event) and (2) in the open Bay during the wet season (n = 4 sites; 1 sampling event, ten days after a large storm event). Site locations, sample event dates/times, and key site and storm characteristics are summarized in Text S2 and Tables S2, S3.†
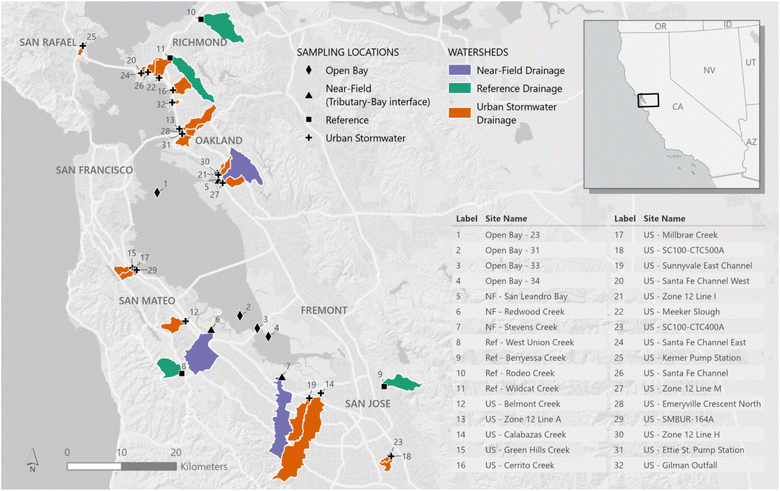 |
| Fig. 1 Map of sampling sites, with inset map providing broader context for the sampling region (San Francisco Bay) within California. The sampling site markers represent the four types of sampling sites—open Bay, near-field (NF), reference (Ref), and urban stormwater (US)—and indicate the specific sampling locations. Site numbers adjacent to site markers on the map correspond to site names provided in the lower right-hand table. Watersheds corresponding to the near-field, reference, and urban stormwater sampling sites are denoted on the map and colored by drainage type. | |
Urban stormwater site selection criteria included watershed land use >80% urban (including residential, commercial, industrial, and transportation) and watershed size generally >1 km2. Selected sites had 69–100% highly developed land uses (average 93%), average 65% impervious area,24 and drainage areas 0.66–50 km2. Site selection was influenced by independent legacy contaminant monitoring efforts, yielding a bias towards higher industrial land use (0–37%, average 10.6%) than the regional average (3.5%). Reference sites had >90% low intensity land use (with average impervious area 4%) and drainage areas 12–23 km2. Although reference watersheds contained less industrial and commercial land use than urban stormwater sites, some contaminant sources do exist (e.g., residential construction, urban product usage), given high overall urbanization of the Bay Area (for detailed land use percentages and imperviousness characteristics by watershed, see Table S2†). Near-field estuarine sites were also selected based on highly urban watershed land use (>92%) and a feasible/safe access point at the Bay's edge. Overall, site selection aimed to avoid any significant hydraulic or contaminant influence of combined sewer systems that are present regionally. Open Bay sites were a subset of existing sites sampled independently each month by the U.S. Geological Survey that were closest in proximity to near-field sites.
Due to the region's Mediterranean climate, most Bay Area waterways are nearly or completely dry during both the dry season (summer) and non-storm conditions within the wet season (autumn – spring). All sampled storms (n = 11) were forecast for >1.3 cm within 6 h. Actual rainfall during sampled storms was 0.2–5.4 cm (average 2.6 cm), with 2 h maximum rainfalls of 0.2–2.3 cm. One first-of-season event (11/26/2019) was sampled at four sites. Among other site/storm event pairs, ∼93% (n = 27/29) had one-week antecedent rainfall >2.54 mm, while ∼55% (n = 16/29) had one-week antecedent rainfall >6.35 mm.
Sample collection
Sample analyses focused on four classes of emerging contaminants that required multiple sampling techniques: a suite of novel stormwater tracers (SWCECs; including 6PPDQ), OPEs, bisphenols, and PFASs. At urban stormwater and reference sites, time-paced composite samples were collected using a variable number of sub-samples (typically 3–7; sub-sample size/quantity selected to ensure sufficient composite sample volume for analysis and to represent most of the storm hydrograph, given estimated storm duration). Sub-samples for PFASs were stored in separate 50 mL polypropylene centrifuge tubes for compositing at the laboratory. Sub-samples for OPEs, bisphenols, and SWCECs were field composited in amber glass containers. We note that many sites were sampled only once, limiting substantive analyses with respect to relationships between observed contaminant concentrations and hydrologic conditions such as storm size, intensity, or antecedent dry period.
All near-field and open Bay samples were single time-point grabs, with salinity measurements also recorded (via salinity refractometer). Additional sample collection details, including class/site-specific sample collection techniques and field blank collection methods, are provided in Text S3 and Table S4.† All samples were refrigerated at 4 °C generally for <12 h before packaging on ice for overnight shipment to each analytical laboratory.
Sample extraction and analysis
Analytical laboratories included University of Washington Tacoma (UWT) for SWCECs (including 6PPDQ), Eurofins West Sacramento (Eurofins) for supplemental 6PPDQ, Southern Illinois University (SIU) for OPEs and bisphenols, Colorado School of Mines (Mines) and Eurofins for PFASs. All reported concentrations describe total water values (i.e., concentrations include both dissolved-phase and particle-bound analytes). Complete sample extraction and analytical method details are in Text S4† and summarized below.
Stormwater tracer CECs
Extraction and quantitative analysis of 25–27 SWCECs including 6PPDQ, other vehicle-derived chemicals (n = 6), benzothiazoles and benzotriazoles (BTH/BTR, n = 4), pharmaceuticals and personal care products (PPCPs, n = 6), and pesticides (n = 8–10; pentachlorophenol and mecoprop analyzed only in 2018–2020, except for two samples in 2021) was based on previously published methods.18,25 Because 6PPDQ was not yet discovered (and therefore lacked an available analytical standard or LC-MS/MS method) at the start of this study, samples collected through 10/24/2021 were first analyzed for other SWCECs, with subsequent re-analysis of the 10/24/2021 event samples with a 6PPDQ-specific LC-MS/MS method. Samples collected 11/4/2021 and later were analyzed with a combined (SWCECs/6PPDQ) LC-MS/MS method. Sample extraction and instrumental analysis details are summarized below, with complete details in Text S4.1 and Tables S5, S6.†
Briefly, samples were split into 1 L triplicates (duplicates for 2021 samples) and spiked with an ISTD mixture (50 μL; n = 14; concentrations 2–20 μg L−1 in final extracts, see Table S7†) and 25 ng (50 μL) 6PPDQ-d5 (the latter spiked only in final extracts for the 10/24/2021 sampling event; not spiked for events before 10/24/2021). Extraction used SPE cartridges (200 mg, 6 mL Oasis HLB, Waters, MA) and elution with methanol (4 × 2.5 mL); after concentration to 1 mL, final extracts were stored at −20 °C until instrumental analysis. Samples with analytes detected at concentrations above the calibration curve range were diluted 10-fold and re-analyzed via the combined LC-MS/MS method. Duplicate method blanks (1 L ultrapure water spiked with ISTDs) were extracted and analyzed in every batch. If enough field water was available, a matrix spike/recovery sample was prepared (50 μL methanolic spike, n = 24 stormwater-derived analytes other than 6PPDQ, 50–500 ng L−1 in water).
LC-MS/MS analyses used an Agilent 1290 Infinity HPLC with reverse-phase C18 analytical and guard columns, coupled to an Agilent G6460A triple-quadrupole MS using electrospray ionization (ESI+/−) and dynamic multiple reaction monitoring, with parameters previously reported.18,25 Data acquisition and sample quantification employed Agilent Mass Hunter Quantitative Analysis software. Two individual ion transitions were monitored where possible, with the higher peak area ion for quantification and the other for qualification. Calibration curves (5–7 points) had R2 > 0.99. The method detection limit (MDL) and method quantification limit (MQL) were determined as the lowest concentrations giving signal to noise (S/N) ratios of 3 and 10, respectively (Tables 1 and S8†).
Table 1 Average 6PPDQ concentrations during storm events in San Francisco Bay Area in 2021–2022. NA indicates a sample was not analyzed by the laboratory
Site Typea |
Sitea |
6PPDQ [ng L−1] (% RPDb) |
UWT |
Eurofins |
Reference, near-field, and urban stormwater sites are listed in order of increasing percent imperviousness in the watershed. All sites were sampled in 2021 except Emeryville Crescent North, which was sampled in 2022.
For open Bay site 34 and Millbrae Creek, the reported relative percent difference (RPD) was calculated from two field replicates (two laboratory replicates were first averaged for each field replicate); for all other UWT analyses, RPD was calculated from two laboratory replicates. Eurofins analyses used a single replicate. UWT MDL 2.5 ng L−1, MQL 5.1 ng L−1; Eurofins MDL 0.54–3.1 ng L−1, MQL 1.7–10 ng L−1 (Table S12).
Results are semi-quantitative.
The reported average is below the MDL (2.5 ng L−1) because it represents the average of duplicate samples, with one result <MDL (considered zero for the calculation) and the other >MDL.
|
Open Bay |
23 |
<MDL |
NA |
31 |
<MDL |
NA |
33 |
<MDL |
NA |
34 |
<MDL |
NA |
Reference |
West Union Creek |
<MDL |
0.85 |
Wildcat Creekc |
1.5 (200)d |
NA |
Near-field |
San Leandro Bayc |
16 (13) |
NA |
Redwood Creekc |
22 (18) |
NA |
Stevens Creekc |
28 (21) |
NA |
Urban stormwater |
Calabazas Creekc |
57 (5.3) |
NA |
Green Hills Creekc |
27 (22) |
NA |
Millbrae Creekc |
25 (4) |
NA |
Sunnyvale East Channelc |
63 (4.8) |
NA |
Meeker Slough |
135 (7.4) |
88 |
Kerner PS |
150 (0) |
84 |
Santa Fe Channel |
130 (0) |
87 |
Emeryville Crescent North |
NA |
240 |
A subset of samples (n = 5) was also analyzed for 6PPDQ by Eurofins (Table 1). Complete sample extraction and instrumental analysis details are in Text S4.2 and Tables S9–S12.† Briefly, field samples and quality control (QC) samples in 250 mL amber glass bottles were fortified with 10 ng (0.5 mL) 13C6-6PPDQ for isotope dilution quantitation. SPE used 200 mg, 6 mL Strata-XL 100 μm polymeric reversed phase cartridges (Phenomenex, 8B-S043-FCH) and elution with acetonitrile (5 mL + 4 mL). Eluents were spiked with 10 ng (0.5 mL) 6PPDQ-d5 as a QC injection ISTD, then adjusted to 10 mL with acetonitrile. Samples were sealed and briefly vortexed before aliquoting a small volume for analysis. LC-MS/MS analyses used a Shimadzu Exion LC with separation on a solid-core C18 column, coupled to a SCIEX 5500+ operating in positive polarity. An initial six-point calibration using an average response factor was injected prior to sample analysis, with percent recovery of the 13C6-6PPDQ standard evaluated for QC. Samples exceeding the calibration range were diluted and reanalyzed.
Organophosphate esters and bisphenols
Extraction and quantitative analysis of 34 OPEs and 17 bisphenols followed a previously published method.23 Complete details of sample processing, extraction, and instrumental analysis are in Text S4.3 and Tables S13–S15.† Briefly, samples (∼1 L) were filtered (0.45 μm, nylon membrane filter; Whatman) to separate dissolved and particulate phases for independent extractions, then spiked with ISTDs for isotope dilution quantitation (10 ng each for OPEs, 20 ng for bisphenols; see Table S15†). Filtered water samples were liquid–liquid extracted with DCM (25 mL, three times); dried particulates were extracted by ultrasonication with HEX
:
DCM, 1
:
1 v/v (5 min, twice, supernatant removed between rounds). For a given matrix, resulting extracts were combined, concentrated, and split into two equal volume aliquots. The aliquot for OPE analysis was solvent-exchanged to hexane, then cleaned further using SPE cartridges (1 g ammonium silica; Biotage, Charlotte, NC). The aliquot for bisphenol analysis was concentrated to near-dryness and reconstituted in methanol. Final extracts (∼200 μL) were spiked with an injection ISTD to evaluate instrumental performance (10 ng 13C18-TPhP for OPEs; 10 ng d16-BPA for bisphenols).
LC-MS/MS analyses used a Shimadzu HPLC coupled to an AB SCIEX Q Trap 5500 MS, using ESI+ for OPEs, ESI− for bisphenols, and MRM, with quantitative and qualitative ion pairs for each analyte (Table S15†). Calibration curves (n = 5–7 points; 0–200 ng mL−1 for OPEs and 0–50 ng mL−1 for bisphenols) had R2 > 0.99. Results for field samples were corrected for observed blank contamination. Final concentrations from dissolved water and particulate fractions were summed to produce total water concentrations (used in all analyses in this study). MDLs were defined as three times the standard deviation of the noise when injecting a low concentration mixture of target analytes. MQLs were either assessed by multiplying the standard deviation of replicate analyses (n = 8) of matrix spikes from each matrix type (Optima water or clean filter) by a Student's t-value designated for a 99% confidence level (analytes without detections in laboratory blanks) or calculated as the average concentration in blanks plus three times the standard deviation in blanks (analytes detectable in laboratory blanks) (Table S16†).
PFASs
Stormwater sample preparation occurred at both Mines (first three wet seasons) and Eurofins (last wet season), with composited samples from the second and third wet seasons archived for analysis by Eurofins in 2022. Samples from the first wet season were analyzed for 45 PFASs at Mines by established methods,26 with subsequent samples analyzed for 73 PFASs at Eurofins using a modified version of USEPA Method 1633.27 Complete details of sample processing, extraction, and instrumental analysis are in Texts S4.4, S4.5 and Tables S17–S19.†
For samples from the first three wet seasons, triplicate composite total water samples were prepared by combining replicate sub-samples. Based on a previously published method,26 composite samples were sub-sampled (2 mL) and prepared for analysis by adding 0.528 mL methanol, 0.292 mL isopropyl alcohol, 0.101 mL basic water (0.01% ammonium hydroxide in water), 0.303 mL 2,2,2-trifluoroethanol, and 0.112 mL PFAS ISTD mixture (2000 ng L−1; Table S17†). Samples were vortexed (60 s) then sonicated (1 h, 50–55 °C). For samples from the fourth wet season (Fall 2021 – Spring 2022), single composite total water samples were prepared by combining up to five storm event sub-samples (each fortified with PFAS ISTDs, total concentration 1.25–2.5 ng mL−1 in final sample extract; Table S19†) in an HDPE bottle. Composite samples were extracted using multi-phase SPE (weak-anion exchange/graphite carbon black). Elution used 8 mL of 0.4% ammonium acetate in methanol (first used to serially rinse sub-sample tubes and the HDPE bottle). Eluents were adjusted to 80
:
20 methanol
:
water, briefly vortexed, and stored at 2–6 °C prior to analysis.
Sample analysis at Mines used HPLC coupled to a SCIEX X500R QTOF-MS system, with calibration curves to quantify each analyte (regression fit 1/x2; analyzed every 24 h; ≥5 points, r2 > 0.98, concentration ± 30% of known).26 Lower and upper limits of quantitation were determined as the lowest and highest calibration standards calculated to be within 30% of the expected value, respectively. If PFASs were detected in any blanks, the MQL was set at the concentration of the next calibration standard that was three times greater than the concentration of that PFAS in the blank (Table S18†). Sample analysis at Eurofins used an Exion LC system and a C18 analytical column coupled to a SCIEX 5500 mass spectrometer with ESI− and MRM; MDLs and MQLs are provided in Table S18.†
Quality assurance review
Excepting 5% of PFAS field sample results, which were rejected by the laboratory for errors or anomalies in the analysis/data recording, all other field sample data (for all compound classes) were included in an independent post hoc quality assurance (QA) review of all data, conducted by San Francisco Estuary Institute. Specific measurement quality objectives for the compounds analyzed in this study were not previously assigned. Thus, QA review used the approach described in the Quality Assurance Program Plan for the Regional Monitoring Program for Water Quality in San Francisco Bay, with acceptance ranges for other trace organic compound groups adapted for compounds analyzed in this study.28 QA parameters and results are summarized below, with additional details provided in Text S5.†
Data quality and method performance were assessed using QC samples (spiked recovery samples and lab duplicates). “Quantitative” concentrations (average recovery 50–200%, average duplicate relative percent difference (RPD) <65%) are known within a factor of two and are provided without further comment. “Semi-quantitative” concentrations (average recovery 25–50% or 200–400%, RPD <120%) have uncertainties within a factor of four and can support order-of-magnitude comparisons. Data for compounds with performance outside of the semi-quantitative range were not considered sufficiently quantitative for inclusion (three SWCECs, five PFASs from Eurofins analyses; quantitative data from Mines was available for one of these five PFASs; see Text S5†).
If laboratory and field blank samples indicated potential influence of background contamination on reported concentrations, and if results were not already blank-corrected or LOQ-corrected, relevant measurements were flagged (QA codes IP, VIP, VIPF, VIPND; Table S20†). This applied to ∼1% of individual measurements (46 of 4034). Comparison of results for compounds analyzed by both PFAS laboratories indicated that reporting limits were in the same order of magnitude for all analytes except PFPeA (Mines 22 ng L−1, Eurofins 2.0 ng L−1).
Statistical analyses
Where sample replicates were available, reported concentrations reflect first averaging laboratory replicates, then field replicates. For sums, averages, and bar charts, non-detects (NDs) were considered zero; for box-whisker plots and other statistical tests, NDs were assigned a value of half their respective MDLs. The non-parametric Spearman's rank correlation test (statistical significance level α = 0.05; only including analytes detected in ≥50% of all available samples; Python 3.11.0, Python Software Foundation, 2022) was selected to analyze pairwise correlations among individual contaminants due to its limited sensitivity to outliers and its ability to handle non-normal datasets (Table S24†). The Wilcoxon rank-sum test (statistical significance level α = 0.05; R 1.2.1335, R Development Core Team, 2019) was chosen to assess differences between urban stormwater samples and near-field and open Bay samples (for contaminants with available data) because it does not require normality.
Results and discussion
6PPDQ
After discovery of 6PPDQ as a transformation product of the tire rubber antiozonant 6PPD and its identification as the toxicant responsible for decades of stormwater-linked coho salmon mortality events in Pacific Northwest watersheds,14 6PPDQ analysis was included for sampling events in autumn 2021 and later. Detections were <MDL – 1.5 ng L−1 at the two reference sites included (West Union and Wildcat Creeks), reflecting limited roadway runoff impacts, and 25–240 ng L−1 at the urban stormwater sites (n = 8, Tables 1 and S20†). Noting that these were time-composited samples that inherently dilute peak runoff concentrations, these data align with the low end of previously documented 6PPDQ concentrations in stormwater and runoff-impacted surface waters in China (210–2430 ng L−1),29 Canada (<MDL – 2300 ng L−1),16,30,31 Australia (0.38–88 ng L−1),17 and the U.S. (36–2300 ng L−1),14,25 including prior reports of Bay Area creeks impacted by stormwater (n = 4/10, 120–420 ng L−1).14,25 Among urban sites in the current study, 75% (n = 6/8) exceeded the lowest reported coho salmon LC50 (41 ng L−1),25,32,33 even with potential dilution, highlighting the potential for lethal and sublethal impacts to sensitive species.34,35 Although coho salmon are now absent from San Francisco Bay watersheds, the estuary supports other sensitive salmonids, including an Endangered Species Act listed population of steelhead trout.35 Furthermore, noting previous observations of 6PPDQ concentration profiles that correspond to or are broader than storm hydrograph peaks,17,30 peak 6PPDQ concentrations during these storm events were likely considerably higher than the time-averaged composite values reported here.
At near-field estuarine sites along the Bay shore, observed 6PPDQ concentrations were 16–28 ng L−1 (Table 1). Near-field estuarine sampling reflected a variable amount of mixing of creek outflow (primarily composed of stormwater runoff) with near-shore tidal water (sample salinity range 0.16–11 g L−1). As the first report, to our knowledge, of 6PPDQ detection in near-shore estuarine and marine environments, these data highlight the potential exposure of near-shore aquatic organisms to 6PPDQ concentrations similar to those observed in urban creeks. This concentration similarity, also observed for several other SWCECs (discussed below), indicated the potential for more limited mixing as well as transient episodes of high concentration, minimally diluted stormwater runoff in near-shore marine and estuarine environments. However, in open Bay samples collected 10 days after a storm event, dilution and/or other fate processes reduced concentrations below detection limits.
Other SWCECs
Beyond 6PPDQ, all SWCECs were detected in at least three urban stormwater samples (DF 14–100%, median 86%; see Tables 2, S21;†Fig. 2 and S1†). Individual compound classes are discussed below. Overall, we note no consistent positive pairwise correlations were observed for any individual SWCECs with respect to analytes from other contaminant classes included here (i.e., OPEs, bisphenols, PFASs). This finding is consistent with the hypothesis that these compounds represent distinct sources and transport processes, and thus should be individually considered important components of future monitoring efforts.
Table 2 Concentrations of stormwater tracer CECs in San Francisco Bay Area in 2018–2021. Units are ng L−1. Detection frequency (DF) reported as a percentage of samples analyzed. NA indicates a sample was not analyzed by the laboratory. 6PPDQ: 6PPD-quinone; DCU: 1,3-dicyclohexylurea; DPG: 1,3-diphenylguanidine; HMMM: hexa(methoxymethyl)melamine; NCBA: N-cyclohexyl-2-benzothiazolamine; 2,4-MoBT: 2-(4-morpholinyl)benzothiazole; 2-NH2-BTH: 2-amino-benzothiazole; 2-OH-BTH: 2-hydroxy-benzothiazole; BTR: benzotriazole; 5-methyl-1H-BTR: 5-methyl-1H-benzotriazole
Analyte class |
Analyte |
Open Bay (n = 4) |
Near-field (n = 3) |
Reference (n = 4) |
Urban stormwater (n = 21) |
Min |
Max |
Min |
Max |
Min |
Max |
Min |
Median |
Max |
DF [%] |
6PPDQ data collected only in 2021 (n = 4 open Bay, n = 3 near-field, n = 2 reference sites, n = 8 urban stormwater; highest concentration detection at a given site considered to calculate median).
Mecoprop and PCP data collected at n = 14 urban stormwater sites (analyzed in only one 2021 sampling event, 1/26–27/2021).
|
Vehicle-derived |
6PPDQa |
<MDL |
<MDL |
16 |
28 |
<MDL |
1.5 |
25 |
97 |
240 |
100 |
DCU |
<MDL |
12 |
36 |
60 |
<MDL |
100 |
36 |
120 |
1100 |
100 |
DPG |
14 |
90 |
180 |
660 |
5.6 |
780 |
290 |
1200 |
6700 |
100 |
HMMM |
11 |
57 |
130 |
2700 |
0.84 |
44 |
290 |
990 |
7400 |
100 |
NCBA |
<MDL |
<MDL |
<MDL |
5.5 |
<MDL |
4.2 |
<MDL |
31 |
100 |
86 |
2,4-MoBT |
<MDL |
<MDL |
<MDL |
<MDL |
<MDL |
5.9 |
<MDL |
39 |
88 |
81 |
BTH/BTR |
2-NH2-BTH |
<MDL |
<MDL |
<MDL |
<MDL |
<MDL |
15 |
<MDL |
22 |
58 |
81 |
2-OH-BTH |
<MDL |
96 |
19 |
1100 |
<MDL |
250 |
<MDL |
310 |
3400 |
86 |
5-Methyl-1H-BTR |
56 |
3200 |
<MDL |
170 |
<MDL |
18 |
<MDL |
430 |
7400 |
86 |
BTR |
250 |
4700 |
<MDL |
1500 |
<MDL |
110 |
<MDL |
290 |
2200 |
86 |
PPCPs |
Caffeine |
22 |
38 |
790 |
1300 |
2.6 |
55 |
120 |
700 |
8500 |
100 |
Cetirizine |
<MDL |
21 |
1.1 |
2.8 |
<MDL |
1.5 |
<MDL |
1.3 |
13 |
81 |
Cotinine |
5.2 |
15 |
13 |
16 |
<MDL |
5.6 |
8.2 |
24 |
130 |
100 |
Ibuprofen |
<MDL |
35 |
<MDL |
<MDL |
<MDL |
<MDL |
<MDL |
130 |
4100 |
62 |
Triclosan |
<MDL |
1.1 |
<MDL |
<MDL |
<MDL |
<MDL |
<MDL |
<MDL |
5.7 |
24 |
Pesticides |
Carbendazim |
7.0 |
31 |
43 |
110 |
<MDL |
23 |
39 |
81 |
530 |
100 |
DEET |
<MDL |
9 |
9.9 |
18 |
<MDL |
10 |
6.5 |
25 |
110 |
100 |
Diuron |
7.4 |
33 |
130 |
2000 |
0.69 |
150 |
100 |
280 |
1400 |
100 |
Prometon |
<MDL |
<MDL |
<MDL |
8.2 |
<MDL |
<MDL |
<MDL |
0.38 |
18 |
57 |
Imidacloprid |
<MDL |
<MDL |
<MDL |
95 |
<MDL |
36 |
<MDL |
27 |
410 |
90 |
Thiamethoxam |
<MDL |
<MDL |
<MDL |
<MDL |
<MDL |
<MDL |
<MDL |
<MDL |
12 |
14 |
Clothianidin |
<MDL |
<MDL |
<MDL |
7.5 |
<MDL |
24 |
<MDL |
<MDL |
20 |
19 |
Mecopropb |
NA |
NA |
<MDL |
18 |
<MDL |
19 |
260 |
79 |
PCPb |
NA |
NA |
7.4 |
25 |
94 |
300 |
450 |
100 |
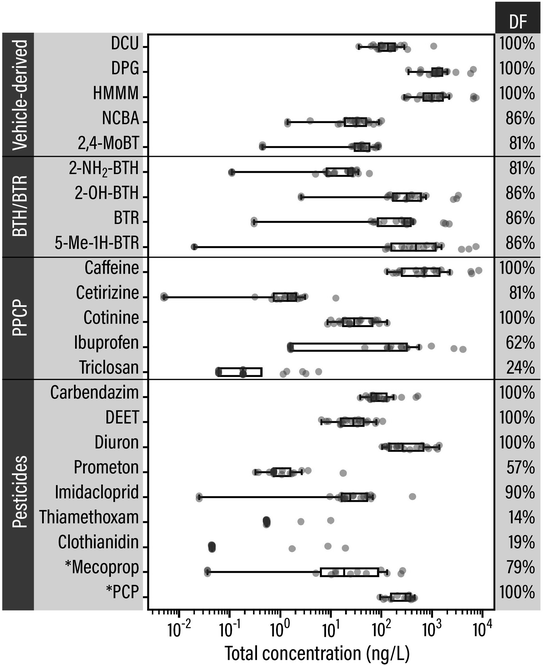 |
| Fig. 2 Box-and-whisker plot summarizing stormwater CEC concentrations in urban stormwater samples, for vehicle-derived compounds, benzothiazoles/benzotriazoles (BTH/BTR), pharmaceuticals and personal care products (PPCP), and pesticides. Detection frequencies (DF) are based on 21 urban stormwater samples, except for analytes denoted by asterisk (*): mecoprop and pentachlorophenol (PCP) (n = 14). Boxes indicate 25th–75th percentile, whiskers indicate 10th–90th percentile, and a point is plotted for every sampling event, with all non-detects (i.e., concentrations <MDL) plotted as 0.5*MDL (see Table S8† for MDL values). 6PPDQ: 6PPD-quinone; DCU: 1,3-dicyclohexylurea; DPG: 1,3-diphenylguanidine; HMMM: hexa(methoxymethyl)melamine; NCBA: N-cyclohexyl-2-benzothiazolamine; 2,4-MoBT: 2-(4-morpholinyl)benzothiazole; 2-NH2-BTH: 2-amino-benzothiazole; 2-OH-BTH: 2-hydroxy-benzothiazole; BTR: benzotriazole; 5-Me-1H-BTR: 5-methyl-1H-benzotriazole. | |
Vehicle-derived contaminants
Two tire rubber-derived contaminants exhibited median concentrations ∼8–40-fold higher than other vehicle-derived SWCECs in urban stormwater samples, with 100% detection frequencies: 1,3-diphenylguanidine (DPG; 290–6700 ng L−1, median 1200 ng L−1) and hexa(methoxymethyl)melamine (HMMM; 290–7400 ng L−1, median 990 ng L−1) (Table 2). These data align with previous reports of highly elevated, ubiquitous DPG and HMMM detections in urban runoff across the US and globally.15,17,30,31,36,37 The industrial compound 1,3-dicyclohexylurea (DCU), also reported in tire leachates and urban runoff,15–17,38 was detected in 100% of urban stormwater samples at 36–1100 ng L−1 (median 120 ng L−1; Table 2). Likewise, N-cyclohexyl-2-benzothiazolamine (NCBA) and 2-(4-morpholinyl)benzothiazole (2,4-MoBT), which are impurities of vulcanization accelerators and were previously identified as markers of tire tread wear particles in urban sediment and dust,39–41 were detected in >80% of urban stormwater samples with median concentrations of 31 and 39 ng L−1, respectively (Table 2). Although these data theoretically reflect total water concentrations, SPE is optimized for extraction from the dissolved phase; lower concentrations of NCBA and 2,4-MoBT relative to other tire-derived chemicals may thus be indicative of their occurrence primarily sorbed to sediment or particulate fractions, which may not be fully extracted during SPE. Limited to no aquatic toxicity information is available for these compounds, highlighting an important data gap that limits any effective risk assessment for most vehicle and rubber derived contaminants.
Notably, HMMM, DPG, and DCU were detected frequently in reference sites, near-field Bay sites, and the open Bay (DF 100% for all compound/site type pairs, except DF 75% for DCU in reference sites and the open Bay), emphasizing the environmental ubiquity of these tire-derived chemicals. However, significantly lower concentrations of DPG and DCU were detected in near-field Bay sites, the open Bay, and reference sites relative to concentrations in urban stormwater sites (Wilcoxon rank-sum test, p < 0.05). Likewise, NCBA and 2,4-MoBT concentrations were <MDL – 6 ng L−1 in all near-field, open Bay, and reference site samples (vs. <MDL – 100 ng L−1 in urban stormwater sites; DF 81–86%). In contrast, although HMMM concentrations were lower in reference sites and the open Bay relative to observations in urban stormwater sites (Wilcoxon rank-sum test, p < 0.05), HMMM concentrations in near-field sites (130–2700 ng L−1) were not significantly different from those in urban stormwater (Wilcoxon rank-sum test, p = 0.81). This difference indicated different transport processes for HMMM relative to other tire-derived contaminants between urban stormwater sites (e.g., creeks, storm drains/outfalls) and near-shore estuarine environments. This difference also aligned with highly positive correlations between all pairs of tire-derived chemicals (r > 0.75) besides HMMM, and moderately positive correlations between HMMM and all other tire-derived chemicals (r = 0.56–0.66) (Table S24†). Additional field sampling and laboratory studies are needed to systematically evaluate distinctions in contaminant sources, transport, and fate in these types of locations.
Benzothiazoles & benzotriazoles
Benzothiazoles (BTHs) and benzotriazoles (BTRs) are commonly used as corrosion inhibitors (e.g., in metals, antifreeze);19 BTHs are also used as vulcanization accelerators in tire rubber production.42 Both classes are commonly detected in urban matrices, including tire wear particles, road dust, sediment, wastewater, and stormwater runoff.19,43–46 Both classes have also been associated with a variety of adverse effects in aquatic organisms and humans, including endocrine disruption, hepatotoxicity, neurotoxicity, and carcinogenicity,47,48 with predicted no effect concentrations (PNECs) for freshwater ecosystems of 20
000 ng L−1 for BTR and 5-methyl-1H-BTR49 and 33
000 ng L−1 for 2-OH-BTH.47 All BTHs/BTRs measured here were detected in >80% of urban stormwater samples, with median concentrations >290 ng L−1 for all except 2-NH2-BTH (median 22 ng L−1) (Table 2). Maximum urban stormwater concentrations of 2-OH-BTH, BTR, and 5-methyl-1H-BTR were >2000 ng L−1, indicating the combined impact of a few significant sources, or contributions from many sources in stormwater.
Both BTR and 5-methyl-1H-BTR were detected in the open Bay at concentrations >3000 ng L−1 (i.e., ∼0.4 to 2-fold of maximum urban stormwater concentrations and ∼3 to 19-fold higher than maximum near-field concentrations), underscoring the role of municipal wastewater effluent as another major pathway for discharge of benzotriazoles to the Bay.1,50,51 BTR and 5-methyl-1H-BTR were positively correlated across all sites (r = 0.85; Table S24†), but were not correlated to any vehicle-derived contaminants or benzothiazoles (r < 0.40; Table S24†), further emphasizing the role of distinct source inputs. In contrast, 2-NH2-BTH and 2-OH-BTH were both at least moderately positively correlated to all vehicle-derived chemicals except HMMM and, for 2-OH-BTH, DPG (r = 0.53–0.73; Table S24†). Finally, we note elevated concentrations of 2-OH-BTH (250 ng L−1) and BTR (110 ng L−1) at the Wildcat Creek reference site, a distinct occurrence relative to concentrations observed for other analytes. Although further sampling is needed to evaluate the reproducibility of this observation and the potential impact of localized sources, such detections indicate how widespread some of these SWCECs are and that this site may not be an appropriate regional reference location for future BTH/BTR sampling.
PPCPs
Although PPCPs are historically considered markers of wastewater impacts,52,53 their utility is substantially confounded by growing recognition of substantial PPCP occurrence and transport to receiving waters by stormwater runoff.15,54 The data collected here further support substantial PPCP mass contributions from stormwater pathways. Four of five PPCPs analyzed (caffeine, cetirizine, cotinine, and ibuprofen) were detected in >60% of urban stormwater samples, with median concentrations >100 ng L−1 for both ibuprofen (<MDL – 4100 ng L−1, median 130 ng L−1) and caffeine (120–8500 ng L−1, median 700 ng L−1) (Table 2). While ibuprofen was below detection limits at almost every other site type (reference, near-field, open Bay), caffeine was detected ubiquitously, exhibiting near-field concentrations (n = 3; 790–1300 ng L−1) that were not significantly different from urban stormwater concentrations (n = 21; Wilcoxon rank-sum test, p = 0.45). In less-urban reference sites (2.6–55 ng L−1) and in the open Bay (22–38 ng L−1), caffeine was present at levels ∼1–2 orders of magnitude lower than in urban stormwater/near-field samples.
Based on measured concentrations, we observed moderate to highly positive pair-wise correlations for caffeine with cotinine, all vehicle-derived chemicals, 2-OH-BTH, carbendazim, and diuron (r = 0.60–0.89; Table S24†), likely reflecting common urban transport pathways (i.e., road/roadside surfaces) and the potential for caffeine to act as a chemical surrogate for other urban contaminants. These data also agree well with our previous identification of caffeine within a chemical profile for which occurrence and abundance corresponded to a watershed-scale urbanization gradient.4
Pesticides
At urban stormwater sites, the fungicide carbendazim, the pesticide/wood preservative pentachlorophenol (PCP), the herbicide diuron, and the insecticides N,N-diethyl-meta-toluamide (DEET) and imidacloprid were detected in ≥90% of samples (noting the potential impact of analytical interferences on ubiquitous environmental DEET detections55) (Table 2). PCP, which has a freshwater aquatic life benchmark56 of 6900 ng L−1 and is being phased out in the U.S. over 2022–2027,57 was present at 94–450 ng L−1 in urban stormwater sites (median 300 ng L−1; DF 100%, n = 14) and 7.4–25 ng L−1 at reference sites (DF 100%, n = 4) (Table 2). Throughout these data and other datasets we have collected, PCP is surprisingly commonly detected, potentially due to widespread use in telephone poles and/or in railroad ties used for railways or in landscaping. Most pesticide detections at reference sites and in the open Bay were <MDL to 40 ng L−1. Diuron was an exception, with concentrations of 57 and 150 ng L−1 observed at two reference sites (Wildcat and Rodeo Creeks, respectively), with implications for careful selection of reference sites with respect to painted structures, agricultural areas, or areas treated for weed control. Maximum detected near-field concentrations of carbendazim, diuron, and imidacloprid exceeded lowest available US EPA Office of Pesticide Programs' Aquatic Life Benchmarks for freshwater species of 99 ng L−1 (vertebrate), 130 ng L−1 (invertebrate), and 10 ng L−1 (invertebrate), respectively.56 However, these values are for chronic (long-term) exposure; no in-Bay concentrations exceeded acute toxicity thresholds. Maximum stormwater concentrations exceeded acute Aquatic Life Benchmarks only for imidacloprid (freshwater invertebrate), highlighting the potential for imidacloprid to impact urban creek invertebrates.
Concentrations of carbendazim, DEET, diuron, and imidacloprid at urban stormwater sites and near-field sites were not significantly different (Wilcoxon rank-sum test, p = 0.62, 0.20, 0.45, and 0.73 respectively; PCP not analyzed in near-field samples). This comparison again emphasized that near-shore exposures best reflect water quality conditions and compositions in urban creeks for a potentially extended time after storm events, depending on tidal and estuarine mixing conditions. Among pesticides, only carbendazim and diuron were positively correlated (r = 0.92; Table S24†), which might reflect the use of both compounds as biocides in outdoor paints.58 Both carbendazim and diuron were previously identified as components of an urban pesticide signature, with concentration and occurrence related to degree of urbanization at the watershed level.59
OPEs
Of 34 OPEs monitored, 29 were detected in ≥96% of urban stormwater samples, three were detected in a single urban stormwater sample (RBDPP, TPrP, TTBNPP), and two were not detected in any samples (CrDPP, TDBPP) (Tables 3, S22;†Fig. 3b–d and S2–S4†). The data indicate substantial OPE loads transport through the environment, including via stormwater pathways. In urban stormwater samples, summed OPE concentrations ranged from 134–14
400 ng L−1 (median 1300 ng L−1). At reference sites, sums of OPE concentrations were 72–1980 ng L−1. Notably, three of four reference site samples exhibited total OPE concentrations within a factor of three of the median total concentration in urban stormwater samples. This observation emphasized both the ubiquity of OPE contamination and the continued challenge of identifying appropriate reference sites, especially for analytes like OPEs that can exhibit atmospheric transport. Studies elsewhere have detected OPEs in atmospheric particles60–62 and rainfall.63–66
Table 3 Concentrations of bisphenols and OPEs in San Francisco Bay Area in 2018–2022. Units are ng L−1. Detection frequency (DF) reported as a percentage of samples analyzed. Analytes not listed (13 bisphenols – BPA-diglycidyl ether, BPAF, BPAP, BPB, BPBP, BPC, BPC-dichloride, BPE, BPG, BPM, BPP, BPPH, BPTMC; and 2 OPEs – CrDPP, TDBPP) were <MDL at all sites
Analyte group |
Analyte class |
Analyte |
Reference (n = 4) |
Urban stormwater (n = 26) |
Min |
Max |
Min |
Median |
Max |
DF [%] |
Semi-quantitative analytes.
|
Bisphenols |
— |
BPA |
<MDL |
34 |
1.7 |
18 |
740 |
100 |
BPF |
<MDL |
9.2 |
<MDL |
6.3 |
96 |
92 |
BPS |
<MDL |
<MDL |
<MDL |
1.4 |
20 |
54 |
BPZ |
<MDL |
4.3 |
<MDL |
<MDL |
3.3 |
4 |
Organophosphate esters (OPEs) |
Aryl OPEs |
BPA-BDPP |
<MDL |
4.1 |
<MDL |
1.3 |
29 |
96 |
EHDPP |
<MDL |
83 |
2.0 |
24 |
360 |
100 |
IDDPP |
<MDL |
4.2 |
1.1 |
6.7 |
68 |
100 |
RBDPPa |
<MDL |
<MDL |
<MDL |
<MDL |
2 |
4 |
T35DMPP |
<MDL |
9.9 |
0.6 |
2.8 |
74 |
100 |
TPhPa |
0.8 |
62 |
2.3 |
23 |
110 |
100 |
TMPP |
<MDL |
61 |
5.2 |
21 |
510 |
100 |
Alkyl OPEs |
TBOEP |
14 |
360 |
24 |
320 |
5900 |
100 |
TEHP |
<MDL |
140 |
6.7 |
53 |
580 |
100 |
TEP |
1.0 |
28 |
2.2 |
15 |
390 |
100 |
TPrP |
<MDL |
<MDL |
<MDL |
<MDL |
12 |
4 |
TiBP |
0.9 |
21 |
1.1 |
6.1 |
33 |
100 |
TnBP |
9.9 |
140 |
14 |
69 |
300 |
100 |
Br OPEs |
TTBNPP |
<MDL |
<MDL |
<MDL |
<MDL |
1.6 |
4 |
Cl OPEs |
TCEP |
1.3 |
86 |
1.6 |
27 |
710 |
100 |
TCIPP |
11 |
400 |
18 |
210 |
1700 |
100 |
TDCIPP |
9.3 |
380 |
7.2 |
110 |
9300 |
100 |
V6 |
<MDL |
13 |
<MDL |
16 |
330 |
96 |
ITPs |
2IPPDPP |
<MDL |
37 |
0.77 |
14 |
130 |
100 |
4IPPDPP |
0.48 |
32 |
0.46 |
8.4 |
130 |
100 |
24DIPPDPP |
<MDL |
41 |
2.2 |
12 |
62 |
100 |
B2IPPP |
<MDL |
23 |
1.5 |
9.6 |
49 |
100 |
B4IPPP |
<MDL |
21 |
<MDL |
4.1 |
76 |
96 |
B24DIPPP |
<MDL |
19 |
0.93 |
5.3 |
24 |
100 |
T2IPPP |
<MDL |
4.5 |
1.2 |
6.4 |
54 |
100 |
T3IPPP |
<MDL |
8.3 |
0.85 |
5.0 |
33 |
100 |
T4IPPP |
<MDL |
7.2 |
1.0 |
4.5 |
23 |
100 |
TBPPs |
2tBPDPP |
1.5 |
21 |
2.5 |
15 |
100 |
100 |
4tBPDPP |
<MDL |
23 |
5.1 |
20 |
140 |
100 |
B2tBPPP |
<MDL |
43 |
3.2 |
18 |
150 |
100 |
B4tBPPP |
<MDL |
74 |
5.3 |
23 |
170 |
100 |
T4tBPP |
<MDL |
50 |
1.4 |
7.8 |
72 |
100 |
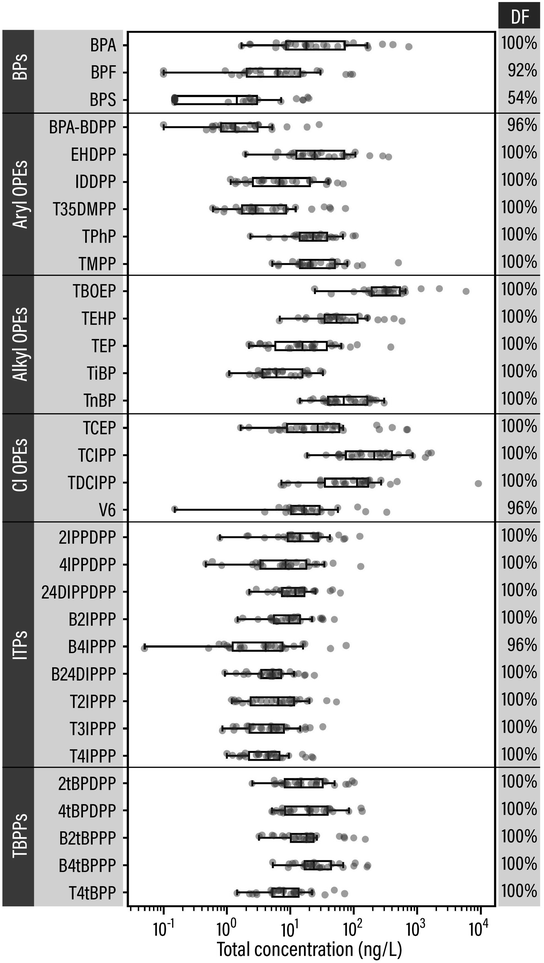 |
| Fig. 3 Box-and-whisker plot summarizing bisphenol (BP) and organophosphate ester (OPE) concentrations (including aryl OPEs, alkyl OPEs, chlorinated [Cl] OPEs, ITPs [isopropylated triarylphosphate esters], and TBPPs [tert-butylated triarylphosphate esters]) in urban stormwater samples for analytes with detection frequencies (DF) > 15%, based on 26 urban stormwater samples. Boxes indicate 25th–75th percentile, whiskers indicate 10th–90th percentile, and a point is plotted for every sampling event, with all non-detects (i.e., concentrations <MDL) plotted as 0.5*MDL (see Table S16† for MDL values). | |
Three analytes—tris(2-butoxyethyl) phosphate (TBOEP), tris(1-chloro-2-propyl) phosphate (TCIPP), and tris(1,3-dichloroisopropyl) phosphate (TDCIPP)—accounted for 29–80% (median 58%) of the summed concentrations of OPEs in urban stormwater samples, with median concentrations of 320 ng L−1 (range 24–5900 ng L−1), 210 ng L−1 (range 18–2700 ng L−1), and 110 ng L−1 (7.2–9300 ng L−1), respectively (Tables 3 and S22†). These values are similar to those reported previously in urban stormwater,3,67–70 including a prior study of grab samples collected from two sites in the Bay Area.21
While a fraction of these three OPEs are likely derived from rainfall, mean precipitation concentrations reported in the literature are generally an order of magnitude lower than mean concentrations in urban stormwater samples observed here.63,64 This suggests that other regional sources are significantly contributing to OPE distributions on surfaces within watersheds, leading to their transport into streams during storm events. These compounds and other OPEs are widely used as additive flame retardants, plasticizers, and for other functions in many outdoor products relevant to the stormwater pathway, including construction materials such as paints, coatings, vinyl resins, polyurethane foams, rubbers, and other plastics; vehicles; textiles; and plastic food packaging.71–76 Stormwater concentrations of TCIPP and TDCIPP were highly positively correlated (r = 0.81; Table S24†). In contrast, correlations of these two OPEs with TBOEP were moderately positive (r = 0.57–0.59; Table S24†), a difference that might be related to different use patterns or varying transport processes.
Many other OPEs exhibited positive pairwise correlations. In particular, OPEs used as flame retardants were also moderately or highly positively correlated with TCIPP and TDCIPP, including TCEP, V6, TPhP, and several members of two newly identified subclasses of OPEs, iso-propylated and tert-butylated triaryl phosphate esters (ITPs and TBPPs; Table S24†). Almost no environmental monitoring data are available for ITPs and TBPPs; this study provided some of the first observations for aqueous samples. The sums of ITPs and TBPPs in urban stormwater represented 6% (range 2–15%) and 8% (range 2–22%), respectively, of the sum OPE concentrations observed in this study.
OPEs were generally not correlated with SWCECs, including those derived from vehicle tires (Table S24†). This is of particular interest because some OPEs are used in other vehicle components and have been observed in vehicle interiors72 and vehicle exhaust.77 While vehicles may be an important source of OPEs to the outdoor environment and to stormwater runoff, the lack of correlation with tire-derived contaminants suggests differences in sources and/or transport processes. OPEs were also generally not correlated with PFASs, but many OPEs exhibited at least moderately positive correlations with BPA and BPF (Table S24†), discussed below. Positive pairwise correlations were previously observed between OPEs and bisphenols in ambient Bay water, suggesting similarities in sources, transport, and/or environmental fate.23
Bisphenols
Four of 17 bisphenols analyzed in this study were detected, including BPA and three common “BPA alternatives”: BPF, BPS, and BPZ (Table 3, S22;†Fig. 3a and S5†). Sum bisphenol concentrations were <MDL – 47 ng L−1 at reference sites and 1.7–850 ng L−1 (median 23 ng L−1) in urban stormwater samples. At lower concentrations, BPS (median 1.4 ng L−1) and BPZ (median <MDL) were detected in 54% and 4% of urban stormwater samples, respectively. In contrast, higher concentrations of BPA (1.7–740 ng L−1, median 18 ng L−1) and BPF (<MDL – 96 ng L−1, median 6.3 ng L−1) were detected in >90% of urban stormwater samples, with detections positively correlated across urban stormwater samples (r = 0.91; Table S24†).
Concentrations of BPA and BPF in this study were in the lower range of values reported in a broader US study of urban stormwater (BPA: <MDL – 2770 ng L−1, median 263 ng L−1; BPF: <MDL – 141 ng L−1, median 11 ng L−1).3 We could find only one other report of BPS, BPF, or other BPA alternatives in the stormwater literature,78 suggesting the data reported here are among the first observations of broader bisphenol concentrations in stormwater. Continued monitoring is needed to understand the contribution of BPA alternatives to overall bisphenol loads and associated toxicity risks in stormwater and San Francisco Bay.
BPA concentrations exceeding 160 ng L−1 were observed in five of 26 urban stormwater samples, with all other samples <100 ng L−1. Sources of bisphenols to urban watersheds include construction materials such as concrete, asphalt, paint, products treated with anti-corrosion epoxy resins, and plastic pipes and gutters; plastic and paper-based litter; and vehicle bodywork and other parts.79–84 BPA and other bisphenols were also observed in vehicle tire and crumb rubber leachate.83,85 BPA has been quantified globally in outdoor air and is generally particle-associated;86 this suggests the potential role of deposition via precipitation to the landscape, though few measurements in rainfall are available. Additionally, BPA and BPF detections at two of four reference sites were within 0.8–1.8-fold of median urban stormwater concentrations, indicating these may not be appropriate reference sites for future bisphenol-focused sampling efforts.
BPA and BPF, the only bisphenols observed with detection frequencies ≥50%, were not correlated to tire-derived contaminants, other SWCECs, or any PFASs (Table S24†). As noted previously, moderately to highly positive correlations of BPA and BPF with some OPEs suggested potential similarities in sources, transport, and/or fate in stormwater.
PFASs
We focused this discussion on perfluorocarboxylic acids (PFCAs), perfluorosulfonic acids (PFSAs), and polyfluoroalkyl phosphate diesters (diPAPs) data because analytes from all other PFAS classes (some of which degrade to PFCAs or PFSAs) exhibited median concentrations <MDL in urban stormwater samples (Table S23†). PFHxA, PFOA, and PFOS accounted for 17%, 12%, and 15% (respective medians) of total detected PFAS concentrations in urban stormwater samples and exhibited the highest maximum concentrations across all PFASs in a given sample. Most PFCAs (chain lengths C3–C10, C12) were detected in ≥69% of urban stormwater samples, with the short-chain compounds (PFPrA, PFBA, PFPeA, PFHxA, PFHpA) representing 38–86% (median 63%) of the total PFCA concentration in any given sample. Median concentrations for all PFCAs ranged from <MDL – 10 ng L−1; the highest measured concentrations for PFCAs in urban stormwater samples were 180 ng L−1 for PFHxA and 110 ng L−1 for PFOA (Table 4; Fig. 4 and S6–S7†). The maximum observed concentration for almost all PFCAs (except PFPrA and PFTrDA) occurred at a single site (Emeryville Crescent North), although specific source(s) could not be identified. Three PFSAs (PFBS, PFHxS, and PFOS) were detected in ≥81% of urban stormwater samples (all other PFSAs detected in ≤12% of samples), with median concentrations of 3.0 ng L−1, 0.97 ng L−1, and 8.5 ng L−1, respectively. The highest measured concentrations for PFSAs in urban stormwater samples were 81 ng L−1 for PFOS and 41 ng L−1 for PFBS. Overall, these observations indicated generally higher concentrations than previously observed in urban stormwater for PFHxA, and similar concentrations for PFOA and PFOS.87–92 Chemical-specific patterns were similar to a previous monitoring study of stormwater in the San Francisco Bay Area, which analyzed samples collected in 2010–2011.93
Table 4 Concentrations of PFCAs, PFSAs, and diPAPs in the San Francisco Bay Area in 2018–2022. Units are ng L−1. Detection frequency (DF) reported as a percentage of samples analyzed. NA indicates a sample was not analyzed by the laboratory
Analyte class |
Analyte |
Reference (n = 4) |
Urban stormwater (n = 26) |
Min |
Max |
Min |
Median |
Max |
DF [%] |
Semi-quantitative analytes.
Sample counts differ from those stated in table headers: reference n = 2, urban stormwater n = 18.
Sample counts differ from those stated in table headers: reference n = 2, urban stormwater n = 8.
Sample counts differ from those stated in table headers: reference n = 0, urban stormwater n = 10.
|
PFCAs |
PFPrAb |
4.0 |
5.9 |
<MDL |
5.2 |
37 |
83 |
PFBAb |
3.5 |
16 |
3.9 |
7.4 |
32 |
100 |
PFPeA |
<MDL |
7.9 |
<MDL |
5.9 |
41 |
69 |
PFHxA |
<MDL |
8.2 |
4 |
10 |
180 |
100 |
PFHpA |
<MDL |
3.6 |
<MDL |
3.9 |
51 |
88 |
PFOA |
1.5 |
6.8 |
2.9 |
7.7 |
110 |
100 |
PFNA |
<MDL |
1.8 |
<MDL |
1.9 |
22 |
69 |
PFDA |
0.41 |
1.6 |
<MDL |
4.2 |
54 |
96 |
PFUnDA |
<MDL |
<MDL |
<MDL |
<MDL |
7.5 |
42 |
PFDoDAa |
<MDL |
<MDL |
<MDL |
1.3 |
9.7 |
69 |
PFTrDA |
<MDL |
<MDL |
<MDL |
<MDL |
4.1 |
8 |
PFTeDA |
<MDL |
<MDL |
<MDL |
<MDL |
1.5 |
12 |
PFHxDA |
<MDL |
<MDL |
<MDL |
<MDL |
0.35 |
4 |
PFSAs |
PFPrS |
<MDL |
<MDL |
<MDL |
<MDL |
0.53 |
4 |
PFBS |
0.87 |
4.1 |
1.4 |
3.0 |
41 |
100 |
PFPeS |
<MDL |
<MDL |
<MDL |
<MDL |
1.8 |
12 |
PFHxS |
<MDL |
1.1 |
<MDL |
0.97 |
15 |
81 |
PFHpS |
<MDL |
<MDL |
<MDL |
<MDL |
<MDL |
0 |
PFOS |
<MDL |
11 |
<MDL |
8.5 |
81 |
88 |
PFNS |
<MDL |
<MDL |
<MDL |
<MDL |
0.325 |
4 |
PFDSc |
<MDL |
<MDL |
<MDL |
<MDL |
<MDL |
0 |
PFDoDS |
<MDL |
<MDL |
<MDL |
<MDL |
0.35 |
4 |
diPAPs |
6:2-diPAPa,d |
NA |
0.81 |
1.2 |
3.2 |
100 |
8:2-diPAPa,d |
NA |
<MDL |
0.25 |
0.99 |
90 |
10:2-diPAPd |
NA |
<MDL |
<MDL |
0.32 |
10 |
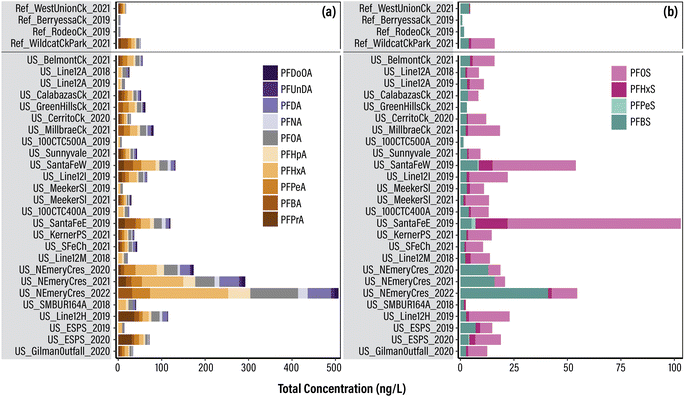 |
| Fig. 4 Bar charts summarizing concentrations of: (a) perfluorocarboxylic acids (PFCAs) and (b) perfluorosulfonic acids (PFSAs) with detection frequencies (DF) >15% in urban stormwater samples. Non-detects (i.e., concentrations <MDL) are not plotted (i.e., represented as a concentration of zero) (see Table S18† for MDL values). Sample names indicate SiteType_SiteName_Year, where Ref = reference sites and US = urban stormwater sites (site types are also separated by a black line). Site order from top to bottom is based on (1) the percent of impervious landcover in the watershed that corresponds to each sampling site (see Table S2†), and (2) chronological order for repeat sampling at a given site (see Table S3†). | |
Moderately to strongly positive statistically significant pairwise correlations (r = 0.55–0.92) were observed for PFHxA, PFHpA, and PFOA with most C5–C11 PFCAs and PFBS, and for PFBS with PFHxS (r = 0.63) (Table S24†). These correlations were consistent with anticipated similarity in sources, transport processes, and/or fate in urban stormwater for these individual PFASs. Apart from well-known industrial uses such as fire-fighting foams and metal-plating mist suppressants, PFAS sources particularly relevant to outdoor urban environments include construction materials such as paints and coatings, roofing materials, outdoor textiles, solar panels, and synthetic turf; paper-based litter; and automotive parts and fluids.94–96 We note that the specific PFASs used in many of these products likely need to undergo transformation to form the intermediate and terminal products (PFCAs and PFSAs) analyzed in this study. PFASs also occur in precipitation, which may contribute to observed concentrations in stormwater.97,98 However, rainwater concentrations reported elsewhere are typically in the lower range of values reported here, and the distribution of PFASs in rainfall is generally skewed towards higher concentrations of the short- or ultra-short-chain PFASs,97,98 which is inconsistent with the distribution observed in these stormwater samples. This comparison suggests other PFAS sources or transport processes contribute more significantly to concentrations observed in stormwater.
To evaluate polyfluoroalkyl precursors to PFCAs that are used directly as components of consumer products, a pilot analysis of fluorotelomer-based diPAPs was performed on a subset of ten urban stormwater samples. DiPAPs are commercial surfactants used in paper products including food packaging,99 among others. They have been previously detected in indoor dust,100 sediment,101–103 and benthic organisms,104 and are known to transform to PFCAs105 in the environment. Detection frequencies for 6:2-diPAP and 8:2-diPAP in our urban stormwater samples were ≥90% (median concentrations 1.2 ng L−1 and 0.25 ng L−1, respectively). The highest measured concentration for diPAPs was 3.2 ng L−1 for 6:2-diPAP (Table 4). This pilot effort suggests these consumer goods-derived PFCA precursors may be widely distributed in environmental matrices, albeit at low concentrations, and are relevant to stormwater transport.
This study also provided insights about PFASs exhibiting few or no detections in these urban stormwater samples. For example, more recently identified PFASs of interest without a significant presence in these stormwater samples included HFPO-DA, ADONA, PFECA (A, B, F, G), and F53B minor (all DF 0%), and F53B major (DF 3%, detected in single sample). Fluorotelomer sulfonates (FTS) were observed sporadically (median DF 0–19%) at 5 ng L−1 or less. Similarly, fluorotelomer carboxylic acids (FTCAs) and fluorotelomer unsaturated carboxylic acids (FTUCAs) exhibited low detection frequencies (DF 0–42% for individual analytes), albeit with infrequent higher concentrations of individual analytes (5–63 ng L−1) observed at four sites and consistent detection of multiple analytes (>2–3 individual FTCA/FTUCAs) only at one site sampled in both 2021 and 2022. Ultimately, PFAS sources, transformation, and transport processes will need more detailed studies to inform management of this complex class, including for stormwater pathways.
Conclusions
Using an especially wide-ranging suite of analytical methods, we characterized the occurrence and concentrations of 154 emerging organic contaminants in stormwater from highly developed watersheds surrounding an urban estuary. These data again emphasize that urban stormwater is an important and often underappreciated transport pathway to receiving waters for many diverse emerging contaminants,3 with major implications for water quality and ecosystem health. For many of the contaminant classes explored here (e.g., OPEs, bisphenols, PFASs, PPCPs, BTH/BTRs), prior studies have often focused on the wastewater pathway,8,106–111 which likely neglects additional loads entering receiving waters in systems with separate stormwater infrastructure. Importantly, water-scarce regions may consider urban stormwater capture to provide a drinking water source. However, due to human health concerns, PFASs and other industrial chemicals lacking thorough toxicological evaluations, but with toxic properties and bioactive functional groups, may require focused management of contaminant sources to the stormwater pathway. In either case, improved characterization of emerging contaminants across stormwater and other diffuse pathways would be needed to fully define exposures, risks, and source contributions in complex urban and near-urban systems.
Across urban stormwater sites, wide concentration ranges were evident for many contaminants, suggesting that sampling at higher temporal and spatial resolution will be required to better characterize concentrations and estimate loads to San Francisco Bay (and other urban estuaries). Such a sampling campaign would also enable improved evaluation of contaminant sources and transport dynamics, including the influence of key watershed and storm characteristics. A major data gap relevant to both future study design and pollution prevention is the limited information available regarding the consumer products and other commercial applications releasing emerging contaminants into outdoor urban environments. This is especially true with respect to industrial chemicals and other understudied contaminants with often surprisingly high concentrations and/or detection frequencies. For each chemical and/or class, a comprehensive conceptual model of runoff-relevant sources and transport processes is needed to inform stormwater sampling site selection. Similarly, although evaluation of toxicity risks was outside the scope of the present study, toxicity is a key factor in selection of contaminants for future monitoring programs. Given that toxicity depends on both contaminant concentration and exposure duration, additional focused sampling would also inform improved transport models that connect stormwater hydrographs and contaminant concentration profiles. Collectively, these models, informed by available product testing data and toxicity information, could also guide prioritization of analytes for stormwater monitoring, including identification of suitable tracers that represent specific sources, classes of contaminants, or toxicity impacts. Data generated through this approach would better support broader chemical, product, and stormwater management actions that protect ecological and human health.
Data availability
The data supporting this article have been included as part of the ESI.†
Author contributions
KTP: formal analysis, visualization, writing – original draft, review & editing; AG: conceptualization, investigation, supervision, writing – review & editing; MG: methodology, investigation; ZT: methodology; AW: data curation; DY: data curation, validation; ELM: investigation, formal analysis, writing – review & editing; PA: formal analysis, visualization; DC: methodology, resources, writing – review & editing; AP: methodology, resources, writing – review & editing; NF: methodology, investigation; CPH: methodology, resources, writing – review & editing; EPK: conceptualization, methodology, resources, supervision, writing – review & editing; RS: conceptualization, funding acquisition, project administration, supervision, writing – review & editing.
Conflicts of interest
There are no conflicts to declare.
Acknowledgements
This work (San Francisco Estuary Institute Contribution No. 1156) was supported by the Regional Monitoring Program for Water Quality in San Francisco Bay (RMP). The authors thank SFEI staff including the stormwater monitoring team for support in sample collection, J. Dougherty, K. Paterson, and D. Peterson for assistance with data compilation, K. Palermo for GIS expertise, and R. Askevold for graphical assistance. We also thank Chunjie Xia of Southern Illinois University Carbondale for assistance with analysis of OPEs and bisphenols. The authors are grateful to J. Davis, K. Moran, and L. McKee with SFEI for their constructive feedback, as well as S. Corsi, D. Muir, T. Mumley, H. Stapleton, and A. Ventura. We would like to acknowledge the Indigenous Peoples whose unceded lands surround San Francisco Bay, including the Him-R^n Ohlone Jalquin, Saclan Tribe, the Villages of Lisjan, the Karkin, Muwekma, Ramaytush, Tamien, and Yokuts Ohlone, the Coast and Bay Miwok, Patwin, and the Amah Mutsun Tribal Band.
References
- K. E. Overdahl, R. Sutton, J. Sun, N. J. DeStefano, G. J. Getzinger and P. L. Ferguson, Assessment of Emerging Polar Organic Pollutants Linked to Contaminant Pathways within an Urban Estuary Using Non-Targeted Analysis, Environ. Sci.: Processes Impacts, 2021, 23(3), 429–445, 10.1039/D0EM00463D.
- A. R. Lindborg, K. E. Overdahl, B. Vogler, D. Lin, R. Sutton and P. L. Ferguson, Assessment of Long-Chain Polyethoxylate Surfactants in Wastewater Effluent, Stormwater Runoff, and Ambient Water of San Francisco Bay, CA, ACS ES&T Water, 2023, 3(4), 1233–1242, DOI:10.1021/acsestwater.3c00024.
- J. R. Masoner, D. W. Kolpin, I. M. Cozzarelli, L. B. Barber, D. S. Burden, W. T. Foreman, K. J. Forshay, E. T. Furlong, J. F. Groves, M. L. Hladik, M. E. Hopton, J. B. Jaeschke, S. H. Keefe, D. P. Krabbenhoft, R. Lowrance, K. M. Romanok, D. L. Rus, W. R. Selbig, B. H. Williams and P. M. Bradley, Urban Stormwater: An Overlooked Pathway of Extensive Mixed Contaminants to Surface and Groundwaters in the United States, Environ. Sci. Technol., 2019, 53(17), 10070–10081, DOI:10.1021/acs.est.9b02867.
- K. T. Peter, J. I. Lundin, C. Wu, B. E. Feist, Z. Tian, J. R. Cameron, N. L. Scholz and E. P. Kolodziej, Characterizing the Chemical Profile of Biological Decline in Stormwater-Impacted Urban Watersheds, Environ. Sci. Technol., 2022, 56(5), 3159–3169, DOI:10.1021/acs.est.1c08274.
- G.-L. Wei, D.-Q. Li, M.-N. Zhuo, Y.-S. Liao, Z.-Y. Xie, T.-L. Guo, J.-J. Li, S.-Y. Zhang and Z.-Q. Liang, Organophosphorus Flame Retardants and Plasticizers: Sources, Occurrence, Toxicity and Human Exposure, Environ. Pollut., 2015, 196, 29–46, DOI:10.1016/j.envpol.2014.09.012.
- J.-Y. Lao, S. Xu, K. Zhang, H. Lin, Y. Cao, R. Wu, D. Tao, Y. Ruan, K. M. Yee Leung and P. K. S. Lam, New Perspective to Understand and Prioritize the Ecological Impacts of Organophosphate Esters and Transformation Products in Urban Stormwater and Sewage Effluents, Environ. Sci. Technol., 2023, 57(31), 11656–11665, DOI:10.1021/acs.est.3c04159.
- A. K. Greaves and R. J. Letcher, A Review of Organophosphate Esters in the Environment from Biological Effects to Distribution and Fate, Bull. Environ. Contam. Toxicol., 2017, 98(1), 2–7, DOI:10.1007/s00128-016-1898-0.
- D. Chen, K. Kannan, H. Tan, Z. Zheng, Y.-L. Feng, Y. Wu and M. Widelka, Bisphenol Analogues Other Than BPA: Environmental Occurrence, Human Exposure, and Toxicity—A Review, Environ. Sci. Technol., 2016, 50(11), 5438–5453, DOI:10.1021/acs.est.5b05387.
- A. Podder, A. H. M. A. Sadmani, D. Reinhart, N.-B. Chang and R. Goel, Per and Poly-Fluoroalkyl Substances (PFAS) as a Contaminant of Emerging Concern in Surface Water: A Transboundary Review of Their Occurrences and Toxicity Effects, J. Hazard. Mater., 2021, 419, 126361, DOI:10.1016/j.jhazmat.2021.126361.
- G. M. Sinclair, S. M. Long and O. A. H. Jones, What Are the Effects of PFAS Exposure at Environmentally Relevant Concentrations?, Chemosphere, 2020, 258, 127340, DOI:10.1016/j.chemosphere.2020.127340.
- A. O. De Silva, J. M. Armitage, T. A. Bruton, C. Dassuncao, W. Heiger-Bernays, X. C. Hu, A. Kärrman, B. Kelly, C. Ng, A. Robuck, M. Sun, T. F. Webster and E. M. Sunderland, PFAS Exposure Pathways for Humans and Wildlife: A Synthesis of Current Knowledge and Key Gaps in Understanding, Environ. Toxicol. Chem., 2021, 40(3), 631–657, DOI:10.1002/etc.4935.
-
US EPA, O, Our Current Understanding of the
Human Health and Environmental Risks of PFAS, https://www.epa.gov/pfas/our-current-understanding-human-health-and-environmental-risks-pfas, accessed 2023-12-11.
- P. M. Mayer, K. D. Moran, E. L. Miller, S. M. Brander, S. Harper, M. Garcia-Jaramillo, V. Carrasco-Navarro, K. T. Ho, R. M. Burgess, L. M. Thornton Hampton, E. F. Granek, M. McCauley, J. K. McIntyre, E. P. Kolodziej, X. Hu, A. J. Williams, B. A. Beckingham, M. E. Jackson, R. D. Sanders-Smith, C. L. Fender, G. A. King, M. Bollman, S. S. Kaushal, B. E. Cunningham, S. J. Hutton, J. Lang, H. V. Goss, S. Siddiqui, R. Sutton, D. Lin and M. Mendez, Where the Rubber Meets the Road: Emerging Environmental Impacts of Tire Wear Particles and Their Chemical Cocktails, Sci. Total Environ., 2024, 927, 171153, DOI:10.1016/j.scitotenv.2024.171153.
- Z. Tian, H. Zhao, K. T. Peter, M. Gonzalez, J. Wetzel, C. Wu, X. Hu, J. Prat, E. Mudrock, R. Hettinger, A. E. Cortina, R. G. Biswas, F. V. C. Kock, R. Soong, A. Jenne, B. Du, F. Hou, H. He, R. Lundeen, A. Gilbreath, R. Sutton, N. L. Scholz, J. W. Davis, M. C. Dodd, A. Simpson, J. K. McIntyre and E. P. Kolodziej, A Ubiquitous Tire Rubber–Derived Chemical Induces Acute Mortality in Coho Salmon, Science, 2021, 371(6525), 185–189, DOI:10.1126/science.abd6951.
- K. T. Peter, F. Hou, Z. Tian, C. Wu, M. Goehring, F. Liu and E. P. Kolodziej, More Than a First Flush: Urban Creek Storm Hydrographs Demonstrate Broad Contaminant Pollutographs, Environ. Sci. Technol., 2020, 54(10), 6152–6165, DOI:10.1021/acs.est.0c00872.
- J. K. Challis, H. Popick, S. Prajapati, P. Harder, J. P. Giesy, K. McPhedran and M. Brinkmann, Occurrences of Tire Rubber-Derived Contaminants in Cold-Climate Urban Runoff, Environ. Sci. Technol. Lett., 2021, 8(11), 961–967, DOI:10.1021/acs.estlett.1c00682.
- C. Rauert, N. Charlton, E. D. Okoffo, R. S. Stanton, A. R. Agua, M. C. Pirrung and K. V. Thomas, Concentrations of Tire Additive Chemicals and Tire Road Wear Particles in an Australian Urban Tributary, Environ. Sci. Technol., 2022, 56(4), 2421–2431, DOI:10.1021/acs.est.1c07451.
- F. Hou, Z. Tian, K. T. Peter, C. Wu, A. D. Gipe, H. Zhao, E. A. Alegria, F. Liu and E. P. Kolodziej, Quantification of Organic Contaminants in Urban Stormwater by Isotope Dilution and Liquid Chromatography-Tandem Mass Spectrometry, Anal. Bioanal. Chem., 2019, 411(29), 7791–7806, DOI:10.1007/s00216-019-02177-3.
- J. Asheim, K. Vike-Jonas, S. V. Gonzalez, S. Lierhagen, V. Venkatraman, I.-L. S. Veivåg, B. Snilsberg, T. P. Flaten and A. G. B. Asimakopoulos, Benzothiazoles and Trace Elements in an Urban Road Setting in Trondheim, Norway: Re-Visiting the Chemical Markers of Traffic Pollution, Sci. Total Environ., 2019, 649, 703–711, DOI:10.1016/j.scitotenv.2018.08.299.
- J. A. Davis, F. Hetzel, J. J. Oram and L. J. McKee, Polychlorinated Biphenyls (PCBs) in San Francisco Bay, Environ. Res., 2007, 105(1), 67–86, DOI:10.1016/j.envres.2007.01.013.
- R. Sutton, D. Chen, J. Sun, D. J. Greig and Y. Wu, Characterization of Brominated, Chlorinated, and Phosphate
Flame Retardants in San Francisco Bay, an Urban Estuary, Sci. Total Environ., 2019, 652, 212–223, DOI:10.1016/j.scitotenv.2018.10.096.
- S. L. Klosterhaus, R. Grace, M. C. Hamilton and D. Yee, Method Validation and Reconnaissance of Pharmaceuticals, Personal Care Products, and Alkylphenols in Surface Waters, Sediments, and Mussels in an Urban Estuary, Environ. Int., 2013, 54, 92–99, DOI:10.1016/j.envint.2013.01.009.
- I. Shimabuku, D. Chen, Y. Wu, E. Miller, J. Sun and R. Sutton, Occurrence and Risk Assessment of Organophosphate Esters and Bisphenols in San Francisco Bay, California, USA, Sci. Total Environ., 2022, 813, 152287, DOI:10.1016/j.scitotenv.2021.152287.
-
J. Dewitz, National Land Cover Database (NLCD) 2019 Products, 2021, DOI:10.5066/P9KZCM54.
- Z. Tian, M. Gonzalez, C. A. Rideout, H. N. Zhao, X. Hu, J. Wetzel, E. Mudrock, C. A. James, J. K. McIntyre and E. P. Kolodziej, 6PPD-Quinone: Revised Toxicity Assessment and Quantification with a Commercial Standard, Environ. Sci. Technol. Lett., 2022, 9(2), 140–146, DOI:10.1021/acs.estlett.1c00910.
- C. C. Murray, H. Vatankhah, C. A. McDonough, A. Nickerson, T. T. Hedtke, T. Y. Cath, C. P. Higgins and C. L. Bellona, Removal of Per- and Polyfluoroalkyl Substances Using Super-Fine Powder Activated Carbon and Ceramic Membrane Filtration, J. Hazard. Mater., 2019, 366, 160–168, DOI:10.1016/j.jhazmat.2018.11.050.
-
Method 1633: Analysis of Per- and Polyfluoroalkyl Substances (PFAS) in Aqueous, Solid, Biosolids, and Tissue Samples by LC-MS/MS, 2023.
-
D. Yee, A. Wong and M. Weaver, 2021 Quality Assurance Program Plan for the Regional Monitoring Program for Water Quality in San Francisco Bay; SFEI Contribution No. 1048, San Francisco Estuary Institute, Richmond, CA, 2021 Search PubMed.
- G. Cao, W. Wang, J. Zhang, P. Wu, X. Zhao, Z. Yang, D. Hu and Z. Cai, New Evidence of Rubber-Derived Quinones in Water, Air, and Soil, Environ. Sci. Technol., 2022, 56(7), 4142–4150, DOI:10.1021/acs.est.1c07376.
- C. Johannessen, P. Helm, B. Lashuk, V. Yargeau and C. D. Metcalfe, The Tire Wear Compounds 6PPD-Quinone and 1,3-Diphenylguanidine in an Urban Watershed, Arch. Environ. Contam. Toxicol., 2022, 82, 171–179, DOI:10.1007/s00244-021-00878-4.
- C. Johannessen, P. Helm and C. D. Metcalfe, Detection of Selected Tire Wear Compounds in Urban Receiving Waters, Environ. Pollut., 2021, 287, 117659, DOI:10.1016/j.envpol.2021.117659.
- J. B. Greer, E. M. Dalsky, R. F. Lane and J. D. Hansen, Establishing an In Vitro Model to Assess the Toxicity of 6PPD-Quinone and Other Tire Wear Transformation Products, Environ. Sci. Technol. Lett., 2023, 10(6), 533–537, DOI:10.1021/acs.estlett.3c00196.
- B. P. Lo, V. L. Marlatt, X. Liao, S. Reger, C. Gallilee, A. R. S. Ross and T. M. Brown, Acute Toxicity of 6PPD-Quinone to Early Life Stage Juvenile Chinook (Oncorhynchus Tshawytscha) and Coho (Oncorhynchus Kisutch) Salmon, Environ. Toxicol. Chem., 2023, 42(4), 815–822, DOI:10.1002/etc.5568.
- X. Chen, T. He, X. Yang, Y. Gan, X. Qing, J. Wang and Y. Huang, Analysis, Environmental Occurrence, Fate and Potential Toxicity of Tire Wear Compounds 6PPD and 6PPD-Quinone, J. Hazard. Mater., 2023, 452, 131245, DOI:10.1016/j.jhazmat.2023.131245.
- M. Brinkmann, D. Montgomery, S. Selinger, J. G. P. Miller, E. Stock, A. J. Alcaraz, J. K. Challis, L. Weber, D. Janz, M. Hecker and S. Wiseman, Acute Toxicity of the Tire Rubber-Derived Chemical 6PPD-Quinone to Four Fishes of Commercial, Cultural, and Ecological Importance, Environ. Sci. Technol. Lett., 2022, 9(4), 333–338, DOI:10.1021/acs.estlett.2c00050.
- C. Johannessen, P. Helm and C. D. Metcalfe, Runoff of the Tire-Wear Compound, Hexamethoxymethyl-Melamine into Urban Watersheds, Arch. Environ. Contam. Toxicol., 2022, 82(2), 162–170, DOI:10.1007/s00244-021-00815-5.
- C. Rauert, S. L. Kaserzon, C. Veal, R. Y. Yeh, J. F. Mueller and K. V. Thomas, The First Environmental Assessment of Hexa(Methoxymethyl)Melamine and Co-Occurring Cyclic Amines in Australian Waterways, Sci. Total Environ., 2020, 743, 140834, DOI:10.1016/j.scitotenv.2020.140834.
- K. T. Peter, Z. Tian, C. Wu, P. Lin, S. White, B. Du, J. K. McIntyre, N. L. Scholz and E. P. Kolodziej, Using High-Resolution Mass Spectrometry to Identify Organic Contaminants Linked to Urban Stormwater Mortality Syndrome in Coho Salmon, Environ. Sci. Technol., 2018, 52(18), 10317–10327, DOI:10.1021/acs.est.8b03287.
- H. Kumata, J. Yamada, K. Masuda, H. Takada, Y. Sato, T. Sakurai and K. Fujiwara, Benzothiazolamines as Tire-Derived Molecular Markers: Sorptive Behavior in Street Runoff and Application to Source Apportioning, Environ. Sci. Technol., 2002, 36(4), 702–708, DOI:10.1021/es0155229.
- H. Kumata, Y. Sanada, H. Takada and T. Ueno, Historical Trends of N -Cyclohexyl-2-Benzothiazolamine, 2-(4-Morpholinyl)Benzothiazole, and Other Anthropogenic Contaminants in the Urban Reservoir Sediment Core, Environ. Sci. Technol., 2000, 34(2), 246–253, DOI:10.1021/es990738k.
- K. M. Unice, M. L. Kreider and J. M. Panko, Comparison of Tire and Road Wear Particle Concentrations in Sediment for Watersheds in France, Japan, and the United States by Quantitative Pyrolysis GC/MS Analysis, Environ. Sci. Technol., 2013, 47, 8138–8147, DOI:10.1021/es400871j.
- J. Zhang, X. Zhang, L. Wu, T. Wang, J. Zhao, Y. Zhang, Z. Men and H. Mao, Occurrence of Benzothiazole and Its Derivates in Tire Wear, Road Dust, and Roadside Soil, Chemosphere, 2018, 201, 310–317, DOI:10.1016/j.chemosphere.2018.03.007.
- B. Seiwert, P. Klöckner, S. Wagner and T. Reemtsma, Source-Related Smart Suspect Screening in the Aqueous Environment: Search for Tire-Derived Persistent and Mobile Trace Organic Contaminants in Surface Waters, Anal. Bioanal. Chem., 2020, 412(20), 4909–4919, DOI:10.1007/s00216-020-02653-1.
- A. Kloepfer, M. Jekel and T. Reemtsma, Determination of Benzothiazoles from Complex Aqueous Samples by Liquid Chromatography–Mass Spectrometry Following Solid-Phase Extraction, J. Chromatogr. A, 2004, 1058(1–2), 81–88, DOI:10.1016/j.chroma.2004.08.081.
- A. Kloepfer, M. Jekel and T. Reemtsma, Occurrence, Sources, and Fate of Benzothiazoles in Municipal Wastewater Treatment Plants, Environ. Sci. Technol., 2005, 39(10), 3792–3798, DOI:10.1021/es048141e.
- R. B. Spies, B. D. Andresen and Jr D. W. Rice, Benzthiazoles in Estuarine Sediments as Indicators of Street Runoff, Nature, 1987, 327(6124), 697–699, DOI:10.1038/327697a0.
- C. Liao, U.-J. Kim and K. Kannan, A Review of Environmental Occurrence, Fate, Exposure, and Toxicity of Benzothiazoles, Environ. Sci. Technol., 2018, 52(9), 5007–5026, DOI:10.1021/acs.est.7b05493.
- Z. Q. Shi, Y. S. Liu, Q. Xiong, W. W. Cai and G. G. Ying, Occurrence, Toxicity and Transformation of Six Typical Benzotriazoles in the Environment: A Review, Sci. Total Environ., 2019, 661, 407–421, DOI:10.1016/j.scitotenv.2019.01.138.
- A. Seeland, M. Oetken, A. Kiss, E. Fries and J. Oehlmann, Acute and Chronic Toxicity of Benzotriazoles to Aquatic Organisms, Environ. Sci. Pollut. Res., 2012, 19(5), 1781–1790, DOI:10.1007/s11356-011-0705-z.
- S. Huntscha, T. B. Hofstetter, E. L. Schymanski, S. Spahr and J. Hollender, Biotransformation of Benzotriazoles: Insights from Transformation Product Identification and Compound-Specific Isotope Analysis, Environ. Sci. Technol., 2014, 48(8), 4435–4443, DOI:10.1021/es405694z.
- S. Weiss, J. Jakobs and T. Reemtsma, Discharge of Three Benzotriazole Corrosion Inhibitors with Municipal Wastewater and Improvements by Membrane Bioreactor Treatment and Ozonation‚Ä, Environ. Sci. Technol., 2006, 40(23), 7193–7199, DOI:10.1021/es061434i.
- I. J. Buerge, T. Poiger, M. D. Müller and H.-R. Buser, Caffeine, an Anthropogenic Marker for Wastewater Contamination of Surface Waters, Environ. Sci. Technol., 2003, 37(4), 691–700, DOI:10.1021/es020125z.
- H.-R. Buser, T. Poiger and M. D. Müller, Occurrence and Environmental Behavior of the Chiral Pharmaceutical Drug Ibuprofen in Surface Waters and in Wastewater, Environ. Sci. Technol., 1999, 33(15), 2529–2535, DOI:10.1021/es981014w.
- P. M. Bradley, C. A. Journey, K. M. Romanok, L. B. Barber, H. T. Buxton, W. T. Foreman, E. T. Furlong, S. T. Glassmeyer, M. L. Hladik, L. R. Iwanowicz, D. K. Jones, D. W. Kolpin, K. M. Kuivila, K. A. Loftin, M. A. Mills, M. T. Meyer, J. L. Orlando, T. J. Reilly, K. L. Smalling and D. L. Villeneuve, Expanded Target-Chemical Analysis Reveals Extensive Mixed-Organic-Contaminant Exposure in U.S. Streams, Environ. Sci. Technol., 2017, 51(9), 4792–4802, DOI:10.1021/acs.est.7b00012.
- S. Merel, A. I. Nikiforov and S. A. Snyder, Potential Analytical Interferences and Seasonal Variability in Diethyltoluamide Environmental Monitoring Programs, Chemosphere, 2015, 127, 238–245, DOI:10.1016/j.chemosphere.2015.02.025.
-
US EPA, Aquatic Life Benchmarks and Ecological Risk Assessments for Registered Pesticides, US EPA, https://www.epa.gov/pesticide-science-and-assessing-pesticide-risks/aquatic-life-benchmarks-and-ecological-risk, accessed 2023-12-11 Search PubMed.
-
U.S. EPA, Pentachlorophenol, https://www.epa.gov/ingredients-used-pesticide-products/pentachlorophenol#:%7E:text=UseofPCPisbeing,transitionfromPCPtoalternatives, accessed 2023-06-30.
- U. Schoknecht, J. Gruycheva, H. Mathies, H. Bergmann and M. Burkhardt, Leaching of Biocides Used in Façade Coatings under Laboratory Test Conditions, Environ. Sci. Technol., 2009, 43(24), 9321–9328, DOI:10.1021/es9019832.
- L. H. Nowell, P. W. Moran, L. M. Bexfield, B. J. Mahler, P. C. Van Metre, P. M. Bradley, T. S. Schmidt, D. T. Button and S. L. Qi, Is There an Urban Pesticide Signature? Urban Streams in Five U.S. Regions Share Common Dissolved-Phase Pesticides but Differ in Predicted Aquatic Toxicity, Sci. Total Environ., 2021, 793, 148453, DOI:10.1016/j.scitotenv.2021.148453.
- A. Salamova, Y. Ma, M. Venier and R. A. Hites, High Levels of Organophosphate Flame Retardants in the Great Lakes Atmosphere, Environ. Sci. Technol. Lett., 2014, 1(1), 8–14, DOI:10.1021/ez400034n.
- K. Yang, Q. Li, M. Yuan, M. Guo, Y. Wang, S. Li, C. Tian, J. Tang, J. Sun, J. Li and G. Zhang, Temporal Variations and Potential Sources of Organophosphate Esters in PM2.5 in Xinxiang, North China, Chemosphere, 2019, 215, 500–506, DOI:10.1016/j.chemosphere.2018.10.063.
- S. Lai, Z. Xie, T. Song, J. Tang, Y. Zhang, W. Mi, J. Peng, Y. Zhao, S. Zou and R. Ebinghaus, Occurrence and Dry Deposition of Organophosphate Esters in Atmospheric Particles over the Northern South China Sea, Chemosphere, 2015, 127, 195–200, DOI:10.1016/j.chemosphere.2015.02.015.
- J. Regnery and W. Püttmann, Seasonal Fluctuations of Organophosphate Concentrations in Precipitation and Storm Water Runoff, Chemosphere, 2010, 78(8), 958–964, DOI:10.1016/j.chemosphere.2009.12.027.
- U.-J. Kim and K. Kannan, Occurrence and Distribution of Organophosphate Flame Retardants/Plasticizers in Surface Waters, Tap Water, and Rainwater: Implications for Human Exposure, Environ.
Sci. Technol., 2018, 52(10), 5625–5633, DOI:10.1021/acs.est.8b00727.
- T. F. M. Rodgers, J. W. Truong, L. M. Jantunen, P. A. Helm and M. L. Diamond, Organophosphate Ester Transport, Fate, and Emissions in Toronto, Canada, Estimated Using an Updated Multimedia Urban Model, Environ. Sci. Technol., 2018, 52(21), 12465–12474, DOI:10.1021/acs.est.8b02576.
- Y. Shi, L. Gao, W. Li, Y. Wang, J. Liu and Y. Cai, Occurrence, Distribution and Seasonal Variation of Organophosphate Flame Retardants and Plasticizers in Urban Surface Water in Beijing, China, Environ. Pollut., 2016, 209, 1–10, DOI:10.1016/j.envpol.2015.11.008.
- A. Burant, W. Selbig, E. T. Furlong and C. P. Higgins, Trace Organic Contaminants in Urban Runoff: Associations with Urban Land-Use, Environ. Pollut., 2018, 242, 2068–2077, DOI:10.1016/j.envpol.2018.06.066.
- D. J. Fairbairn, S. M. Elliott, R. L. Kiesling, H. L. Schoenfuss, M. L. Ferrey and B. M. Westerhoff, Contaminants of Emerging Concern in Urban Stormwater: Spatiotemporal Patterns and Removal by Iron-Enhanced Sand Filters (IESFs), Water Res., 2018, 145, 332–345, DOI:10.1016/j.watres.2018.08.020.
- B. Awonaike, Y. D. Lei, A. Parajulee, C. P. J. Mitchell and F. Wania, Polycyclic Aromatic Hydrocarbons and Quinones in Urban and Rural Stormwater Runoff: Effects of Land Use and Storm Characteristics, ACS ES&T Water, 2021, 1(5), 1209–1219, DOI:10.1021/acsestwater.0c00287.
- D. Wicke, A. Matzinger, H. Sonnenberg, N. Caradot, R.-L. Schubert, R. Dick, B. Heinzmann, U. Dünnbier, D. Von Seggern and P. Rouault, Micropollutants in Urban Stormwater Runoff of Different Land Uses, Water, 2021, 13(9), 1312, DOI:10.3390/w13091312.
- H. Zhu, M. M. Al-Bazi, T. A. Kumosani and K. Kannan, Occurrence and Profiles of Organophosphate Esters in Infant Clothing and Raw Textiles Collected from the United States, Environ. Sci. Technol. Lett., 2020, 7(6), 415–420, DOI:10.1021/acs.estlett.0c00221.
- J. Lexén, M. Bernander, I. Cotgreave and P. L. Andersson, Assessing Exposure of Semi-Volatile Organic Compounds (SVOCs) in Car Cabins: Current Understanding and Future Challenges in Developing a Standardized Methodology, Environ. Int., 2021, 157, 106847, DOI:10.1016/j.envint.2021.106847.
- R. Bi and G. Su, Dietary Intake Assessment of Known and Unknown Organophosphate Esters (OPEs) in Foodstuffs via High-Resolution Mass Spectrometry, Sci. Total Environ., 2023, 854, 158452, DOI:10.1016/j.scitotenv.2022.158452.
- S. Harrad, M. Sharkey, W. A. Stubbings, M. Alghamdi, H. Berresheim, M. Coggins, A. H. Rosa and D. Drage, Chlorinated Organophosphate Esters in Irish Waste Foams and Fabrics: Concentrations, Preliminary Assessment of Temporal Trends and Evaluation of the Impact of a Concentration Limit Value, Sci. Total Environ., 2023, 859, 160250, DOI:10.1016/j.scitotenv.2022.160250.
- J. Fernández-Arribas, T. Moreno and E. Eljarrat, Human Exposure to Organophosphate Esters in Water and Packed Beverages, Environ. Int., 2023, 175, 107936, DOI:10.1016/j.envint.2023.107936.
- Y. Wang, M. Hou, Q. Zhang, X. Wu, H. Zhao, Q. Xie and J. Chen, Organophosphorus Flame Retardants and Plasticizers in Building and Decoration Materials and Their Potential Burdens in Newly Decorated Houses in China, Environ. Sci. Technol., 2017, 51(19), 10991–10999, DOI:10.1021/acs.est.7b03367.
- M. J. Fabiańska, B. Kozielska, J. Konieczyński and P. Bielaczyc, Occurrence of Organic Phosphates in Particulate Matter of the Vehicle Exhausts and Outdoor Environment – A Case Study, Environ. Pollut., 2019, 244, 351–360, DOI:10.1016/j.envpol.2018.10.060.
- Z. Huang, J.-L. Zhao, Y.-Y. Yang, Y.-W. Jia, Q.-Q. Zhang, C.-E. Chen, Y.-S. Liu, B. Yang, L. Xie and G.-G. Ying, Occurrence, Mass Loads and Risks of Bisphenol Analogues in the Pearl River Delta Region, South China: Urban Rainfall Runoff as a Potential Source for Receiving Rivers, Environ. Pollut., 2020, 263, 114361, DOI:10.1016/j.envpol.2020.114361.
- C. Liao and K. Kannan, Widespread Occurrence of Bisphenol A in Paper and Paper Products: Implications for Human Exposure, Environ. Sci. Technol., 2011, 45(21), 9372–9379, DOI:10.1021/es202507f.
- M. K. Björnsdotter, J. De Boer and A. Ballesteros-Gómez, Bisphenol A and Replacements in Thermal Paper: A Review, Chemosphere, 2017, 182, 691–706, DOI:10.1016/j.chemosphere.2017.05.070.
- A. Markiewicz, K. Björklund, E. Eriksson, Y. Kalmykova, A.-M. Strömvall and A. Siopi, Emissions of Organic Pollutants from Traffic and Roads: Priority Pollutants Selection and Substance Flow Analysis, Sci. Total Environ., 2017, 580, 1162–1174, DOI:10.1016/j.scitotenv.2016.12.074.
- E. L. M. Vermeirssen, C. Dietschweiler, I. Werner and M. Burkhardt, Corrosion Protection Products as a Source of Bisphenol A and Toxicity to the Aquatic Environment, Water Res., 2017, 123, 586–593, DOI:10.1016/j.watres.2017.07.006.
- K. Lamprea, A. Bressy, C. Mirande-Bret, E. Caupos and M.-C. Gromaire, Alkylphenol and Bisphenol A Contamination of Urban Runoff: An Evaluation of the Emission Potentials of Various Construction Materials and Automotive Supplies, Environ. Sci. Pollut. Res., 2018, 25(22), 21887–21900, DOI:10.1007/s11356-018-2272-z.
- D. Jiang, W.-Q. Chen, X. Zeng and L. Tang, Dynamic Stocks and Flows Analysis of Bisphenol A (BPA) in China: 2000–2014, Environ. Sci. Technol., 2018, 52(6), 3706–3715, DOI:10.1021/acs.est.7b05709.
- C. Halsband, L. Sørensen, A. M. Booth and D. Herzke, Car Tire Crumb Rubber: Does Leaching Produce a Toxic Chemical Cocktail in Coastal Marine Systems?, Front. Environ. Sci., 2020, 8, 125, DOI:10.3389/fenvs.2020.00125.
- T. Vasiljevic and T. Harner, Bisphenol A and Its Analogues in Outdoor and Indoor Air: Properties, Sources and Global Levels, Sci. Total Environ., 2021, 789, 148013, DOI:10.1016/j.scitotenv.2021.148013.
- V. T. Nguyen, M. Reinhard and G. Y.-H. Karina, Occurrence and Source Characterization of Perfluorochemicals in an Urban Watershed, Chemosphere, 2011, 82(9), 1277–1285, DOI:10.1016/j.chemosphere.2010.12.030.
- N. A. Procopio, R. Karl, S. M. Goodrow, J. Maggio, J. B. Louis and T. B. Atherholt, Occurrence and Source Identification of Perfluoroalkyl Acids (PFAAs) in the Metedeconk River Watershed, New Jersey, Environ. Sci. Pollut. Res., 2017, 24(35), 27125–27135, DOI:10.1007/s11356-017-0309-3.
- J. R. Masoner, D. W. Kolpin, I. M. Cozzarelli, P. M. Bradley, B. B. Arnall, K. J. Forshay, J. L. Gray, J. F. Groves, M. L. Hladik, L. E. Hubbard, L. R. Iwanowicz, J. B. Jaeschke, R. F. Lane, R. B. McCleskey, B. F. Polite, D. A. Roth, M. B. Pettijohn and M. C. Wilson, Contaminant Exposure and Transport from Three Potential Reuse Waters within a Single Watershed, Environ. Sci. Technol., 2023, 57(3), 1353–1365, DOI:10.1021/acs.est.2c07372.
- F. Xiao, M. F. Simcik and J. S. Gulliver, Perfluoroalkyl Acids in Urban Stormwater Runoff: Influence of Land Use, Water Res., 2012, 46(20), 6601–6608, DOI:10.1016/j.watres.2011.11.029.
- L. Zhao, M. Zhou, T. Zhang and H. Sun, Polyfluorinated and Perfluorinated Chemicals in Precipitation and Runoff from Cities Across Eastern and Central China, Arch. Environ. Contam. Toxicol., 2013, 64(2), 198–207, DOI:10.1007/s00244-012-9832-x.
- J. L. Wilkinson, J. Swinden, P. S. Hooda, J. Barker and S. Barton, Markers of Anthropogenic Contamination: A Validated Method for Quantification of Pharmaceuticals, Illicit Drug Metabolites, Perfluorinated Compounds, and Plasticisers in Sewage Treatment Effluent and Rain Runoff, Chemosphere, 2016, 159, 638–646, DOI:10.1016/j.chemosphere.2016.06.039.
- E. F. Houtz and D. L. Sedlak, Oxidative Conversion as a Means of Detecting Precursors to Perfluoroalkyl Acids in Urban Runoff, Environ. Sci. Technol., 2012, 46(17), 9342–9349, DOI:10.1021/es302274g.
- J. Glüge, M. Scheringer, I. T. Cousins, J. C. DeWitt, G. Goldenman, D. Herzke, R. Lohmann, C. A. Ng, X. Trier and Z. Wang, An Overview of the Uses of Per- and Polyfluoroalkyl Substances (PFAS), Environ. Sci.: Processes Impacts, 2020, 22(12), 2345–2373, 10.1039/D0EM00291G.
-
S. R. Fernandez, C. Kwiatkowski and T. Bruton, Building a Better World: Eliminating Unnecessary PFAS in Building Materials, 2021, https://greensciencepolicy.org/docs/pfas-building-materials-2021.pdf.
- H. Zhu and K. Kannan, A Pilot Study of Per- and Polyfluoroalkyl Substances in Automotive Lubricant Oils from the United States, Environ. Technol. Innovation, 2020, 19, 100943, DOI:10.1016/j.eti.2020.100943.
- S. B. Gewurtz, L. E. Bradley, S. Backus, A. Dove, D. McGoldrick, H. Hung and H. Dryfhout-Clark, Perfluoroalkyl Acids in Great Lakes Precipitation and Surface Water (2006–2018) Indicate Response to Phase-Outs, Regulatory Action, and Variability in Fate and Transport Processes, Environ. Sci. Technol., 2019, 53(15), 8543–8552, DOI:10.1021/acs.est.9b01337.
- K. A. Pike, P. L. Edmiston, J. J. Morrison and J. A. Faust, Correlation Analysis of Perfluoroalkyl Substances in Regional U.S. Precipitation Events, Water Res., 2021, 190, 116685, DOI:10.1016/j.watres.2020.116685.
- L. Minet, Z. Wang, A. Shalin, T. A. Bruton, A. Blum, G. F. Peaslee, H. Schwartz-Narbonne, M. Venier, H. Whitehead, Y. Wu and M. L. Diamond, Use and Release of Per- and Polyfluoroalkyl Substances (PFASs) in Consumer Food Packaging in U.S. and Canada, Environ. Sci.: Processes Impacts, 2022, 24(11), 2032–2042, 10.1039/D2EM00166G.
- U. Eriksson and A. Kärrman, World-Wide Indoor Exposure to Polyfluoroalkyl Phosphate Esters (PAPs) and Other PFASs in Household Dust, Environ. Sci. Technol., 2015, 49(24), 14503–14511, DOI:10.1021/acs.est.5b00679.
- E. I. H. Loi, L. W. Y. Yeung, S. A. Mabury and P. K. S. Lam, Detections of Commercial Fluorosurfactants in Hong Kong Marine Environment and Human Blood: A Pilot Study, Environ. Sci. Technol., 2013, 47(9), 4677–4685, DOI:10.1021/es303805k.
- R. Guo, D. Megson, A. L. Myers, P. A. Helm, C. Marvin, P. Crozier, S. Mabury, S. P. Bhavsar, G. Tomy, M. Simcik, B. McCarry and E. J. Reiner, Application of a Comprehensive Extraction Technique for the Determination of Poly- and Perfluoroalkyl Substances (PFASs) in Great Lakes Region Sediments, Chemosphere, 2016, 164, 535–546, DOI:10.1016/j.chemosphere.2016.08.126.
- M. D. Sedlak, J. P. Benskin, A. Wong, R. Grace and D. J. Greig, Per- and Polyfluoroalkyl Substances (PFASs) in San Francisco Bay Wildlife: Temporal Trends, Exposure Pathways, and Notable Presence of Precursor Compounds, Chemosphere, 2017, 185, 1217–1226, DOI:10.1016/j.chemosphere.2017.04.096.
- M. Chen, Q. Wang, Y. Zhu, L. Zhu, B. Xiao, M. Liu and L. Yang, Species Dependent Accumulation and Transformation of 8:2 Polyfluoroalkyl Phosphate Esters in Sediment by Three Benthic Organisms, Environ. Int., 2019, 133, 105171, DOI:10.1016/j.envint.2019.105171.
- A. A. Rand and S. A. Mabury, Is There a Human Health Risk Associated with Indirect Exposure to Perfluoroalkyl Carboxylates (PFCAs)?, Toxicology, 2017, 375, 28–36, DOI:10.1016/j.tox.2016.11.011.
- E. R. V. Dickenson, S. A. Snyder, D. L. Sedlak and J. E. Drewes, Indicator Compounds for Assessment of Wastewater Effluent Contributions to Flow and Water Quality, Water Res., 2011, 45(3), 1199–1212, DOI:10.1016/j.watres.2010.11.012.
- R. Loos, R. Carvalho, D. C. António, S. Comero, G. Locoro, S. Tavazzi, B. Paracchini, M. Ghiani, T. Lettieri, L. Blaha, B. Jarosova, S. Voorspoels, K. Servaes, P. Haglund, J. Fick, R. H. Lindberg, D. Schwesig and B. M. Gawlik, EU-Wide Monitoring Survey on Emerging Polar Organic Contaminants in Wastewater Treatment Plant Effluents, Water Res., 2013, 47(17), 6475–6487, DOI:10.1016/j.watres.2013.08.024.
- D. J. Fairbairn, W. A. Arnold, B. L. Barber, E. F. Kaufenberg, W. C. Koskinen, P. J. Novak, P. J. Rice and D. L. Swackhamer, Contaminants of Emerging Concern: Mass Balance and Comparison of Wastewater Effluent and Upstream Sources in a Mixed-Use Watershed, Environ. Sci. Technol., 2016, 50(1), 36–45, DOI:10.1021/acs.est.5b03109.
- X. Zeng, Z. Liu, L. He, S. Cao, H. Song, Z. Yu, G. Sheng and J. Fu, The Occurrence and Removal of Organophosphate Ester Flame Retardants/Plasticizers in a Municipal Wastewater Treatment Plant in the Pearl River Delta, China, J. Environ. Sci. Health, Part A, 2015, 50(12), 1291–1297, DOI:10.1080/10934529.2015.1055158.
- J. Meyer and K. Bester, Organophosphate Flame Retardants and Plasticisers in Wastewater Treatment Plants, J. Environ. Monit., 2004, 6(7), 599, 10.1039/b403206c.
- S. P. Lenka, M. Kah and L. P. Padhye, A Review of the Occurrence, Transformation, and Removal of Poly- and Perfluoroalkyl Substances (PFAS) in Wastewater Treatment Plants, Water Res., 2021, 199, 117187, DOI:10.1016/j.watres.2021.117187.
Footnote |
† Electronic supplementary information (ESI) available: PDF and excel files that include additional detail about chemicals/reagents, sampling sites, analytical methods, and QA/QC, as well as supporting tables and figures. See DOI: https://doi.org/10.1039/d4em00117f |
|
This journal is © The Royal Society of Chemistry 2024 |
Click here to see how this site uses Cookies. View our privacy policy here.