Application of psychrotolerant quorum quenching Planococcus versutus sp. L10.15T to membrane bioreactors for biofouling control at low temperatures†
Received
25th September 2023
, Accepted 12th January 2024
First published on 15th January 2024
Abstract
Bacterial quorum quenching (QQ) (e.g., degradation of N-acyl homoserine lactones (AHLs)) has been utilized to mitigate biofouling in membrane bioreactors (MBRs). However, the anti-biofouling efficiency of QQ bacteria may decrease at low temperatures. To enhance the resilience of QQ technology to cold climates, psychrotolerant Planococcus versutus sp. L10.15T was applied to MBRs at 10 °C in the form of polymeric beads entrapping L10.15T. The efficiency of AHL degradation by L10.15T-beads was compared to that by beads entrapping Rhodococcus sp. BH4, a QQ bacterium that has been widely used in MBRs at room temperature. The AHL degradation by the L10.15T-beads was more efficient than that by the BH4-beads for most types of AHLs tested at 10 °C. The application of the L10.15T-beads to a continuous MBR at 10 °C exhibited that the anti-biofouling efficiency of L10.15T was greater than that of BH4 through more effective reduction in extracellular polymeric substances in the biocake on the membrane surface.
Water impact
Bacterial quorum quenching (QQ) is a promising technology to mitigate biofouling in membrane bioreactors (MBRs). However, the antibiofouling efficiency of a QQ bacterium that was applied in several previous studies decreased at low temperatures. Planococcus versutus sp. L10.15T, a psychrotolerant QQ bacterium, was first applied to MBRs, revealing that it effectively alleviated biofouling in MBRs at 10 °C.
|
1. Introduction
Bacteria in the environment often exist as sessile colonies or biofilms rather than as planktonic cells. Biofilm formation is one of the major causes of biofouling in membrane bioreactor (MBR) processes. Biofouling reduces membrane permeability and increases the number of chemical cleaning and membrane replacement, leading to an increase in the operating costs of MBR processes and potential impacts on the environment due to disposal of used chemicals.1–3
Through quorum sensing (QS), which is density-dependent cellular signaling, microorganisms can form biofilms. Cells secrete and sense signaling molecules (e.g., N-acyl homoserine lactones or AHLs) to detect their population density.4–7 When the concentration of signaling molecules exceeds a threshold value, QS controls specific group behaviors, including biofilm formation.8
Previous studies reported some challenges posed by low temperatures in MBRs. Medina-Martinez et al. found that a low temperature of 12 °C did not hinder the production of N-butanoyl-L-homoserine lactones by Aeromonas hydrophila.9 Lee et al. reported that low temperatures intensified biofouling in MBRs because of an increase in the production of extracellular polymeric substances (EPSs).10 Nahm et al. revealed that the elevated potential of biofilm formation in MBRs at low temperatures was due to an increase in EPS production via the increased production of AHLs.11 Tabraiz et al. reported that low temperatures led to an increase in AHL concentrations of biofilm and enhancement in AHL-mediated QS in anaerobic MBRs.12 According to the above studies, biofouling in MBRs at low temperatures increased with increased AHL-mediated QS and EPS production, raising the necessity for biofouling controls at low temperatures.
Quorum quenching (QQ) refers to the inhibition of QS. One QQ approach is the degradation of AHLs.13,14 Oh et al. isolated Rhodococcus sp. BH4 from an MBR plant, which produces a lactonase that degrades the lactone ring of AHLs.15 Since then, many studies have utilized the strain BH4 as a QQ bacterium to effectively inhibit biofouling in MBRs.16–21 However, to operate MBR plants using QQ strategies in various climates, it is necessary to evaluate the effect of temperature on QQ efficiency in MBRs. Lee et al. reported that the anti-biofouling efficiency of MBRs by QQ using BH4 was reduced at low temperatures as the enzymatic reaction rates decreased.10 To overcome the reduction in QQ efficiency at low temperatures, Min et al. developed a modified culture method including low-temperature subcultivation for the adaptation of BH4 to low temperatures and reported that BH4 cultivated by the method mitigated biofouling of MBRs at low temperatures.22 Alternatively, a viable option is to introduce psychrotolerant bacteria naturally possessing QQ activity at low temperatures into MBRs operated in cold climates, a method yet unexplored.
Therefore, in this study, Planococcus versutus sp. L10.15T was applied to MBRs to improve the QQ efficiency at low temperatures. The QQ efficiency of L10.15T was compared to that of BH4. Batch biofilm formation tests at 10 °C were conducted by varying the types and concentrations of AHLs. Polymeric beads entrapping L10.15T or BH4 were then utilized to evaluate the degradation of the AHLs at 10 °C. Finally, the beads of L10.15T or BH4 were tested in MBRs to compare their respective anti-biofouling efficiencies at 10 °C.
2. Materials and methods
For the batch evaluation of biofilm formation using AHLs, activated sludge samples were taken from laboratory-scale MBRs and cultivated separately. L10.15T and BH4 were cultivated and used to fabricate polymeric beads entrapping either of the two QQ bacteria. AHL degradation by L10.15T- and BH4-beads was analyzed using a luminometer. Finally, the two kinds of beads were separately injected into MBRs operated continuously. The details of materials and methods are described in the subsequent sections.
2.1. Chemicals
Luria–Bertani (LB) broth was purchased from BD (Becton, Dickinson and Company, Franklin Lakes, NJ, USA) for the cultivation of bacteria and activated sludge samples. For the cultivation of Agrobacterium tumefaciens strain A136, spectinomycin dihydrochloride pentahydrate (>98.0%) and tetracycline (>98.8%) were purchased from Sigma-Aldrich (St. Louis, MO, USA). For the biofilm formation assay, crystal violet and ethanol were purchased from Junsei Chemical (Tokyo, Japan) and Duksan Company (Ansan, Republic of Korea), respectively. The AHLs used in this study were N-hexanoyl-DL-homoserine lactone (C6-HSL), N-octanoyl-DL-homoserine lactone (C8-HSL), N-dodecanoyl-DL-homoserine lactone (C12-HSL), N-(3-oxo-hexanoyl)-L-homoserine lactone (3-oxo-C6-HSL), N-3-oxo-octanoyl-L-homoserine lactone (3-oxo-C8-HSL), and N-3-oxo-dodecanoyl-L-homoserine lactone (3-oxo-C12-HSL), which were purchased from Sigma-Aldrich (St. Louis, MO, USA). A β-galactosidase assay kit (Beta-Glo® Assay System) purchased from Promega (Madison, WI, USA) was used to analyze the concentration of AHLs.
2.2. Bacterial strains and culture conditions
The sludge samples taken from laboratory-scale MBRs were incubated in LB broth at 10 °C and stored at −80 °C in a 12.5% glycerol solution.
Planococcus versutus sp. L10.15T (KACC No. 18918), isolated from a soil sample in Antarctica, was obtained from the Korean Agricultural Culture Collection (Wanju-gun, Republic of Korea).23 The strain L10.15T is a psychrotolerant QQ bacterium that exhibits high AHL-degradation activity at low temperatures but has never been applied to MBRs.24Rhodococcus sp. BH4 was isolated from a real MBR plant (Okcheon, Republic of Korea).15 L10.15T and BH4 inoculated into LB broth were incubated at 26 and 30 °C, respectively, for 48 hours.
2.3. Batch tests for biofilm formation induced by various AHLs
Biofilm formation tests with injection of the six AHLs were performed in polystyrene 12-well plates (SPL, Pocheon, Republic of Korea). The optical density at 600 nm (OD600) of the activated sludge cultured in LB broth was measured and adjusted to 0.05 with fresh LB broth. The activated sludge in LB broth was loaded into each well of a 12-well plate together with each type of the AHLs at a concentration of 0, 0.1, 0.5, or 1.0 nM. The plate was incubated at 10 °C for 12 h and then washed thrice with deionized (DI) water. The biofilm formed on the plate was stained with 1% crystal violet for 15 min and destained with 95% ethanol for 2 h. The absorbance of the destaining ethanol solution was measured at 570 nm to compare the relative amount of biofilm.25–27
2.4. Preparation of polymeric beads entrapping QQ bacteria
For the application of QQ bacteria to MBRs, polymeric beads entrapping either L10.15T or BH4 were prepared. The culture of L10.15T or BH4 in LB broth was centrifuged at 8000 × g and 4 °C for 15 min to obtain a cell pellet, which was resuspended in DI water. The bacterial resuspension of L10.15T or BH4 was mixed with a sterilized polymer solution of 10% w/v PVA and 1% w/v sodium alginate. The bacterial concentration in the final solution was adjusted to 5 mg of bacteria per g solution. The solution was used to prepare the polymeric beads, as described previously.22 In this study, beads entrapping L10.15T and BH4 were named L10.15T-beads and BH4-beads, respectively. Polymeric beads with no bacteria (named NB-beads) were also prepared using DI water instead of QQ bacterial resuspension. The average diameter of the beads (L10.15T-beads, BH4-beads, and NB-beads) was 4.0 (±0.2) mm.
Because the beads entrapped the same mass of L10.15T or BH4, the AHL degradation efficiency may depend on the relative amount of live and dead cells inside the beads. Therefore, the viability of L10.15T and BH4 inside the beads was investigated using confocal laser scanning microscopy (CLSM, LSM 710, Carl Zeiss, Oberkochen, Germany) by observing live and dead cells inside the L10.15T-beads and BH4-beads. For the CLSM analysis, the beads were stained with SYTO9 and propidium iodide (PI) enclosed in a bacterial viability kit (L7012, LIVE/DEAD BacLight, Invitrogen, Waltham, MA, USA). SYTO9 and PI were put into a microtube at a volume ratio of 1
:
1 to make a dye mixture. Then, beads were stained in the microtube at 25 °C for 15 min. Finally, the stained beads were washed with DI water. The magnification of CLSM was set to 100 times, and the green and red fluorescence intensities of SYTO9 and PI were analyzed at 500 and 635 nm of wavelength, respectively. CLSM images were converted into binary (black and white) images using ImageJ software (version 1.52a, National Institutes of Health, Bethesda, MD, USA). The live/dead cell ratio was calculated by counting the number of white pixels with level 255 of the binary images and averaging the numbers of the pixels in the images of three different bead samples, corresponding to biological triplicates.
2.5. Assessment of AHL degradation
The QQ efficiencies of the L10.15T- and BH4-beads were analyzed in terms of the degradation rates of the six types of AHLs at 10 °C. Fifty beads were added to 20 mL of 1 μM AHLs (C6-HSL, C8-HSL, C12-HSL, 3-oxo-C6-HSL, 3-oxo-C8-HSL, or 3-oxo-C12-HSL) dissolved in phosphate buffered saline solution (PBS, 0.05 M, pH 7) and allowed to degrade AHLs in a shaking incubator (ISS-3075R, JEIO Tech, Daejeon, Republic of Korea) at 100 rpm and 10 °C for 120 min. Then, a sample (1.0 mL) was taken from the AHL solution and filtered using a 0.45 μm syringe filter (SV13P045NL, Hyundai Micro, Seoul, Republic of Korea). The concentration of AHLs was measured via a bioassay using the reporter strain A. tumefaciens A136(Ti−)(pCF218)(pCF372) and a luminometer (Synergy®, BioTek, Winooski, VT, USA), and more detailed information on the method was provided in previous studies.11,20,21 The strain A136 is a genetically engineered microorganism that lacks the Ti plasmid controlling AHL synthesis and contains two plasmids, pCF218 and pCF372, encoding traR and traI-lacZ fusion genes, respectively.15,28,29 As a result, A136 is unable to produce AHLs independently, and relevant metabolic activities occur only when exogenous AHL signal molecules diffuse into the interior of A136. Exogenous AHL molecules entering the A136 are bound to the AHL receptor protein TraR from the pCF218, forming the TraR-AHL complex. It activates the traI-lacZ (β-galactosidase gene) in pCF372, leading to the production of β-galactosidase. The β-galactosidase is involved in the reactions of the β-galactosidase assay kit, synthesizing oxyluciferin with light emission (luminescence). Consequently, the light intensity measured using a luminometer depends on the concentration of AHLs.
2.6. MBR operation
The beads of L10.15T or BH4 were injected into the MBRs to compare their respective anti-biofouling efficiencies at 10 °C by analyzing the transmembrane pressure (TMP) profiles and the concentration of EPSs in the biocake on the membrane surface. Two laboratory-scale MBRs with the same working volume (2.5 L) were operated continuously in parallel (Fig. 1). Synthetic wastewater was fed into the MBRs. The operating conditions of the MBRs and the composition of the synthetic wastewater are shown in Tables S1 and S2 of the ESI,† respectively. The two MBRs were operated in a water bath to maintain a reactor temperature of 10 °C. One contained either L10.15T-beads (L10.15T-MBR) or BH4-beads (BH4-MBR), and the other contained NB-beads (NB-MBR). The dosage of beads in each reactor was 0.5% v/v (volume of beads per volume of a reactor).
 |
| Fig. 1 Schematic diagram of two identical MBRs: one is L10.15T- or BH4-MBR and the other is NB-MBR (indicating L10.15T- or BH4 bead and NB-bead (no bacteria-bead) injected MBRs, respectively). | |
Membrane modules with an effective membrane surface area of 310 cm2 were prepared using hollow-fiber membranes with 0.04 μm pore size (Zeeweed 10, Veolia Water Technologies & Solutions, Trevose, PA, USA). The water flux through the module was controlled at 20 LMH (L m−2 h−1). The TMP was continuously measured during MBR operations, and the average time required for one TMP jump to 30 kPa (TTMP) was evaluated to quantitatively compare membrane fouling.
2.7. Analysis of EPS concentrations
The bound EPS of the biocake formed on the membrane surface was extracted using a heat extraction method.30 When TMP reached 30 kPa, MBR operation was terminated, and the fouled membrane module was removed from each reactor. The biocake layer formed on the membrane surface was sampled by detaching it using a sonicator (CPX5800H-E, Branson Ultrasonics, Brookfield, CT, USA) at 160 W and 40 kHz in 0.05 M PBS solution for 30 min. A cell pellet was obtained by centrifuging the biocake in PBS at 8000 × g for 15 min and was resuspended in fresh PBS and heated to 95 °C in a water bath for 30 min. Bound EPS samples were obtained by filtering the heated suspension using a syringe filter. The amounts of polysaccharides and proteins in the EPS samples were determined using the phenol-sulfuric acid method and Bradford assay, respectively.31,32
2.8. Statistical methods
All experiments, denoted as n = 3 in the tables and figures, were performed three times independently, and the average values were reported. Error bars in the figures indicate standard deviations. The t-test or analysis of variance (ANOVA) was utilized to determine whether the averages of two or three independent groups were significantly different. For instance, p < 0.05 indicates that the difference in average values is statistically significant at a confidence level of 95%.
3. Results and discussion
3.1. Biofilm formation induced by various AHLs at 10 °C
Fig. 2 shows the effects of the AHL types and concentrations on the biofilm formation at 10 °C in the batch tests using MBR sludge samples. Biofilm formation increased with an increase in the concentration of C6-HSL, C8-HSL, and 3-oxo-C6-HSL at most of the concentration ranges. For 3-oxo-C8-HSL and 3-oxo-C12-HSL, injection of the AHLs at concentrations of up to 0.1 nM enhanced biofilm formation. However, in the case of C12-HSL, the amount of biofilm formed did not change significantly. Overall, the tendency of biofilm formation had a positive correlation with the concentration of five AHLs, except that of C12-HSL. AHL signal molecules synthesized by LuxI proteins in Gram negative bacteria are utilized via QS signaling pathways in which LuxR proteins sense the AHL concentration and initiate a coordinated response by bacteria when a threshold AHL concentration is reached. LuxR-homologue regulatory proteins initiate transcription of selected genes.33 Various genes regulated by the LuxR-homologue in bacteria are known to be related to biofilm formation. Therefore, the injection of AHLs enhanced biofilm formation.
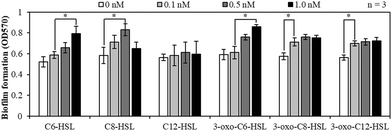 |
| Fig. 2 Biofilm formation of MBR-activated sludge samples at 10 °C using 12-well plates with six AHLs at a concentration of 0, 0.1, 0.5, or 1.0 nM (*: p < 0.05). | |
3.2. AHL degradation by L10.15T and BH4 at 10 °C
The degradation of C8-HSL by L10.15T-beads was investigated at 10, 17, and 25 °C for 120 min (Fig. 3). The degradation efficiency at 10 °C was the highest among those at three temperature conditions. Especially, there was a considerable difference in the initial degradation rates for 0–15 min, which were 2.410, 0.410, and 0.258 h−1 at 10, 17, and 25 °C, respectively (Table S3†). In contrast to BH4 that was reported to have a lower degradation ability at low temperatures than at room temperature,22 L10.15T exhibited more effective degradation of C8-HSL at 10 °C.
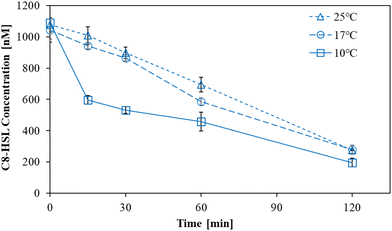 |
| Fig. 3 Degradation of C8-HSLs by L10.15T-beads at 10, 17, and 25 °C (n = 3). | |
Next, the rate constants of AHL degradation by L10.15T at 10 °C for 120 min were compared to those by BH4 for the six AHLs including C8-HSL (Fig. 4). L10.15T exhibited higher level of AHL removal for the four types of AHLs (C6-HSL, C8-HSL, 3-oxo-C6-HSL, and 3-oxo-C8-HSL) than that by BH4 at 10 °C. It is noteworthy that the four types of AHLs were among the five AHLs that showed a positive correlation between the AHL concentration and biofilm formation (Fig. 2). The rate constants for C6-HSL degradation by L10.15T and BH4 were 0.77 and 0.47 h−1, respectively. Those for C8-HSL were 0.65 and 0.53 h−1, respectively; 0.77 and 0.33 h−1 for 3-oxo-C6-HSL, respectively; 0.68 and 0.42 h−1 for 3-oxo-C8-HSL, respectively. Only for the 3-oxo-C12-HSL, the rate constant of degradation by L10.15T was lower than that by BH4. For C12-HSL, the difference in rate constants between L10.15T and BH4 was not significant at the 95% confidence level.
 |
| Fig. 4 Degradation rate constants of six AHLs for 120 min by L10.15T- and BH4-beads at 10 °C (*: p < 0.05). | |
Previous studies reported that Planococcus versutus L10.15T degraded AHLs well because of AidP (autoinducer-degrading enzyme from Planococcus sp.) having high activity at low temperatures.24,34 AidP is an AHL lactonase encoded by the aidP gene contained in Planococcus spp. including L10.15T. The substitution of amino acids in a protein including an enzyme can cause stabilization or destabilization of the protein structure and considerably influence the thermal stability of the protein. Cold-adapted enzymes particularly have high glycine and low arginine composition, which was consistent with the analysis results of AidP. Therefore, the structural characteristics of AidP in L10.15T probably allow AidP to have thermal stability and high activity at low temperatures.
The different patterns of the AHL degradation by L10.15T have been reported previously: L10.15T had the capability of inactivating various AHLs such as C4–C12-HSLs, but the efficiency of the AHL degradation by L10.15T depended on the length of the acyl chain in AHLs.23,24 AHL removal by L10.15T decreased with an increase in the acyl chain length of the AHLs.34 As mentioned above, L10.15T degrades AHLs using AidP, which is known to be a lactonase capable of degrading AHLs, with a decreasing efficiency as the acyl chain length increases.34Fig. 4 exhibits trends similar to those of previous studies: the removal of both unsubstituted and 3-oxo-substituted C6–C12-HSLs by L10.15T gradually decreased with an increase in the acyl chain length. In contrast, AHL removal by BH4 increased with increasing chain length of 3-oxo-substituted AHLs, which is in accordance with a previous result reported by Oh et al.15 Therefore, the removal percentage of 3-oxo-C12-HSL by BH4 was higher than that by L10.15T; however, for most of the other AHLs, L10.15T exhibited better AHL degradation performance than that by BH4. As aforementioned, C6-HSL, C8-HSL, 3-oxo-C6-HSL, and 3-oxo-C8-HSL, which were degraded more efficiently by L10.15T than by BH4 at 10 °C, showed a positive correlation with biofilm formation. This implies that L10.15T would be more effective in biofouling mitigation than BH4 at 10 °C.
The cross-sectional CLSM images of the stained L10.15T- and BH4-beads are shown in Fig. 5. The live/dead cell ratios evaluated from the three sets of images for L10.15T and BH4 were 1.39 (±0.12) and 1.52 (±0.19) (p > 0.05), respectively (Table S4†). This indicates that the live/dead ratios of both beads were not significantly different. Despite the similar live/dead ratio values, the anti-biofouling efficiency of the L10.15T-beads in continuous MBRs at 10 °C would be higher than that of BH4 because of the higher AHL degradation efficiency of L10.15T. As mentioned above, the excellent degradation efficiency of L10.15T results from the lactonase AidP with high activity at low temperatures.
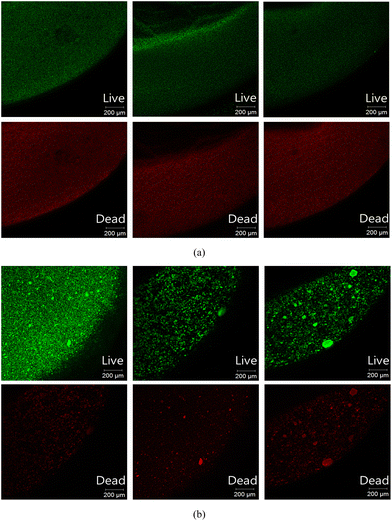 |
| Fig. 5 Live/dead cell analysis of (a) L10.15T- and (b) BH4-beads using CLSM. Green and red fluorescence indicates live and dead cells, respectively. | |
3.3. Application of L10.15T- and BH4-beads to continuous MBRs at 10 °C
L10.15T- or BH4-beads were added to an MBR, which was operated at the same time as another MBR containing NB beads. The resulting TMP profiles are shown in Fig. 6, and the values of TTMP denoting average operation time to reach a TMP of 30 kPa are listed in Table 1. The averaged TTMP values of the L10.15T-MBR and NB-MBR were 4.70 and 2.20 d, respectively, and thus the TTMP ratio of L10.15T-MBR to NB-MBR was 2.14. A TTMP ratio greater than unity indicates that the TMP increase was successfully retarded, compared to that of the NB-MBR. Also, it means that the use of L10.15T results in longer operational time before maintenance or cleaning is required. According to the study by Arif et al., chemical cleaning cost for an MBR with capacity of 680 m3 d−1 was $24
100/y.35 For instance, if L10.15T is applied to the MBR, and thus the TTMP ratio of the L10.15T-MBR and the conventional MBR becomes 2.14, chemical cleaning cost can be theoretically reduced to $11
262/y ($24
100/2.14 = $11
262).
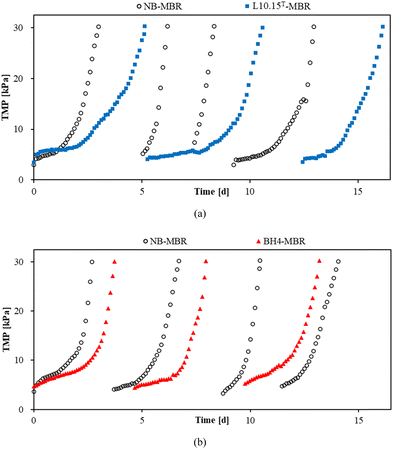 |
| Fig. 6 TMP profiles of (a) L10.15T- and (b) BH4-MBRs (MBRs containing L10.15T- and BH4-beads, respectively) with respect to that of NB-MBR (MBR containing beads with no bacteria) at 10 °C. | |
Table 1 Average operation time to reach a TMP of 30 kPa, TTMP, for the operations of L10.15T- and BH4-MBRs at 10 °C and TTMP ratios
|
T
TMP [d] |
T
TMP ratio |
L10.15T-MBR |
4.70 |
2.14 |
NB-MBR |
2.20 |
BH4-MBR |
3.43 |
1.38 |
NB-MBR |
2.48 |
Meanwhile, the TTMP ratio of L10.15T-MBR (2.14) was 1.55 times greater than that of BH4-MBR (1.38), indicating that the anti-biofouling efficiency of L10.15T was higher than that of BH4 for MBRs at 10 °C. Min et al. reported that when the BH4 cultivated by a modified culture method for the low-temperature adaptation of BH4 was used in MBRs at 10 °C, the TTMP ratio was enhanced by 1.41 times.22 The comparison of TTMP ratio improvement indicates that L10.15T had slightly higher anti-biofouling efficiency than that of the modified BH4 of the previous study.
Bound EPS extracted from the biocake formed on the membrane surface, which is closely related to biofouling via QS, was examined in terms of polysaccharide and protein concentrations when MBR operation was stopped at 30 kPa. As shown in Table 2, the polysaccharide and protein concentrations of L10.15T- and BH4-MBR were lower than those of NB-MBR. However, L10.15T exhibited a greater reduction in the level of polysaccharides than BH4 did. Specifically, the polysaccharide concentration in the biocake was 24.5 (±1.67) mg g−1 for the L10.15T-MBR, corresponding to 42.6% reduction in comparison to 42.7 (±5.68) mg g−1 of the NB-MBR (p < 0.05). For the BH4-MBR, the reduction was 31.4% based on 31.3 (±6.34) and 45.6 (±7.66) mg g−1 of BH4- and NB-MBRs, respectively (p < 0.05). Meanwhile, protein contents in the biocake of L10.15T- and BH4-MBRs were 8.34 (±1.27) and 6.33 (±2.60) mg g−1, respectively, which are not significantly different from each other (p > 0.05). In some previous studies, the concentration of polysaccharides decreased more greatly than that of proteins, which is similar to the results of this study.20,22 However, the degree of reduction in polysaccharide and protein concentrations varied. In our experiences, polysaccharides decreased significantly when the concentration of polysaccharides was much higher than that of proteins. Therefore, it is presumed that when microorganisms secreting polysaccharides more than proteins are dominant, the polysaccharide concentration is influenced significantly by QQ.
Table 2 Bound EPSs extracted from the biocake sampled in L10.15T- and BH4-MBRs (n = 3)
|
Polysaccharide [mg g−1 biocake] |
Protein [mg g−1 biocake] |
L10.15T-MBR |
24.5 (±1.67) |
8.34 (±1.27) |
NB-MBR |
42.7 (±5.68) |
12.7 (±3.26) |
BH4-MBR |
31.3 (±6.34) |
6.33 (±2.60) |
NB-MBR |
45.6 (±7.66) |
9.65 (±3.22) |
There was no significant difference in chemical oxygen demand (COD) and mixed liquor suspended solids during the MBR operation (Table S5†). These results are consistent with those of several previous studies.22,36,37 Therefore, the greater anti-biofouling efficiency of L10.15T-beads is attributed mainly to the more effective reduction in the polysaccharide content resulting from its higher QQ activity than that of BH4 at 10 °C.
4. Conclusion
This study demonstrated that L10.15T was effective in controlling biofouling in MBRs operated at low temperatures. Our data indicate that this was due to the higher degradation rates of AHLs (C6-HSL, C8-HSL, 3-oxo-C6-HSL, and 3-oxo-C8-HSL) by L10.15T than by BH4 at 10 °C. The application of the L10.15T-beads to a continuous MBR confirmed that the anti-biofouling efficiency of L10.15T at 10 °C was greater than that of BH4. This is attributed to the more effective reduction in polysaccharide content in the biocake resulting from the higher QQ activity of L10.15T compared to that of BH4 at 10 °C. It is worth noting that L10.15T was effective in mitigating biofouling in MBRs for wastewater treatment although it was isolated from a soil sample in Antarctica, irrespective of wastewater treatment by MBRs. This implies that QQ microorganisms isolated not only from MBRs but also from other sources at various temperatures would have feasibility for biofouling control in MBRs at conditions similar to their sources. However, as the first application of psychrotolerant QQ bacteria from sources other than MBRs to MBRs, there are some limitations of this study: using a specific bacterium, testing at fixed temperature (10 °C), and conducting experiments only in a laboratory scale using synthetic wastewater having composition similar to domestic wastewater. Future studies will be needed to overcome the limitations and to further develop the findings of this study as follows: i) tests of diverse psychrotolerant QQ bacteria at various temperatures, ii) expansion of research scope to applying various QQ bacteria having some activities under severe conditions such as low/high pH, high salinity, and toxic components in feed solutions, iii) optimization of procedures and conditions for QQ bacteria culture and polymeric media fabrication to enhance QQ performance, iv) assessments of psychrotolerant and other QQ bacteria in pilot- and full-scale MBR plants with a variety of wastewater, and v) evaluation of psychrotolerant QQ bacteria to controlling biofilm formation in cold climates in fields other than MBRs. These will certainly contribute to expanding practical applicability as well as theoretical knowledge of QQ technology for biofouling control.
Author contributions
We all contributed to preparing the manuscript as the following contributor roles taxonomy: data curation, investigation, and writing (original draft), Sojin Min; formal analysis and writing (original draft), Hosung Lee; data curation, Joowan Lim; methodology, Sang Hyun Lee; methodology and writing (review and editing), Seungjin Lee; resources and validation, Kwang-Ho Choo; conceptualization and writing (review and editing), Chung-Hak Lee; conceptualization, supervision and writing (review and editing), Pyung-Kyu Park.
Conflicts of interest
There are no conflicts of interest to declare.
Acknowledgements
This work was supported by Korea Environment Industry & Technology Institute (KEITI) through a project for developing innovative drinking water and wastewater technologies funded by Korea Ministry of Environment (MOE) [No. 2021002690006]; “Regional Innovation Strategy (RIS) project” through the National Research Foundation of Korea (NRF) funded by the Ministry of Education (MOE) [2022RIS-005].
References
- Z. U. Islam, M. Ayub, S. Chung and H. Oh, Effect of Aeration Intensity on Performance of Lab-Scale Quorum-Quenching Membrane Bioreactor, Membranes, 2022, 12, 289 CrossRef CAS PubMed.
- K.-M. Yeon, W.-S. Cheong, H.-S. Oh, W.-N. Lee, B.-K. Hwang, C.-H. Lee, H. Beyenal and Z. Lewandowski, Quorum Sensing: A New Biofouling Control Paradigm in a Membrane Bioreactor for Advanced Wastewater Treatment, Environ. Sci. Technol., 2009, 43, 380–385 CrossRef CAS PubMed.
- N. R. Maddela, A. S. Abiodun, S. Zhang and R. Prasad, Biofouling in Membrane Bioreactors—Mitigation and Current Status: a Review, Appl. Biochem. Biotechnol., 2023, 195, 5643–5668 CrossRef CAS PubMed.
- C. Fuqua, M. R. Parsek and E. P. Greenberg, Regulation of gene expression by cell-to-cell communication: acyl-homoserine lactone quorum sensing, Annu. Rev. Genet., 2001, 35, 439–468 CrossRef CAS PubMed.
- N. A. Whitehead, A. M. Barnard, H. Slater, N. J. Simpson and G. P. Salmond, Quorum-sensing in Gram-negative bacteria, FEMS Microbiol. Rev., 2001, 25, 365–404 CrossRef CAS PubMed.
-
M. E. A. Churchill, H. M. Sibhatu and C. L. Uhlson, in Quorum Sensing: Methods and Protocols, ed. K. P. Rumbaugh, Humana Press, Totowa, NJ, 2011, pp. 159–171, DOI:10.1007/978-1-60761-971-0_12.
- S. Sahreen, H. Mukhtar, K. Imre, A. Morar, V. Herman and S. Sharif, Exploring the Function of Quorum Sensing Regulated Biofilms in Biological Wastewater Treatment: A Review, Int. J. Mol. Sci., 2022, 23, 9751 CrossRef CAS PubMed.
- S. Mukherjee and B. L. Bassler, Bacterial quorum sensing in complex and dynamically changing environments, Nat. Rev. Microbiol., 2019, 17, 371–382 CrossRef CAS PubMed.
- M. Medina-Martinez, M. Uyttendaele, V. Demolder and J. Debevere, Effect of temperature and glucose concentration on the N-butanoyl-L-homoserine lactone production by Aeromonas hydrophila, Food Microbiol., 2006, 23, 534–540 CrossRef CAS PubMed.
- K. Lee, J.-S. Park, T. Iqbal, C. H. Nahm, P.-K. Park and K.-H. Choo, Membrane biofouling behaviors at cold temperatures in pilot-scale hollow fiber membrane bioreactors with quorum quenching, Biofouling, 2018, 34, 912–924 CrossRef CAS PubMed.
- C. H. Nahm, K. Kim, S. Min, H. Lee, D. Chae, K. Lee, K.-H. Choo, C.-H. Lee, I. Koyuncu and P.-K. Park, Quorum sensing: an emerging link between temperature and membrane biofouling in membrane bioreactors, Biofouling, 2019, 35, 443–453 CrossRef CAS PubMed.
- S. Tabraiz, E. Petropoulos, B. Shamurad, M. Quintela-Baluja, S. Mohapatra, K. Acharya, A. Charlton, R. J. Davenport, J. Dolfing and P. J. Sallis, Temperature and immigration effects on quorum sensing in the biofilms of anaerobic membrane bioreactors, J. Environ. Manage., 2021, 293, 112947 CrossRef CAS PubMed.
- J. Huang, Y. Shi, G. Zeng, Y. Gu, G. Chen, L. Shi, Y. Hu, B. Tang and J. Zhou, Acyl-homoserine lactone-based quorum sensing and quorum quenching hold promise to determine the performance of biological wastewater treatments: an overview, Chemosphere, 2016, 157, 137–151 CrossRef CAS PubMed.
- K.-M. Yeon, C.-H. Lee and J. Kim, Magnetic Enzyme Carrier for Effective Biofouling Control in the Membrane Bioreactor Based on Enzymatic Quorum Quenching, Environ. Sci. Technol., 2009, 43, 7403–7409 CrossRef CAS PubMed.
- H. S. Oh, S. R. Kim, W. S. Cheong, C. H. Lee and J. K. Lee, Biofouling inhibition in MBR by Rhodococcus sp. BH4 isolated from real MBR plant, Appl. Microbiol. Biotechnol., 2013, 97, 10223–10231 CrossRef CAS PubMed.
- S. Huang, B. Xu, T. C. A. Ng, M. He, X. Shi and H. Y. Ng, Feasibility of implementing quorum quenching technology to mitigate membrane fouling in MBRs treating phenol-rich pharmaceutical wastewater: Application of Rhodococcus sp. BH4 and quorum quenching consortium, Bioresour. Technol., 2022, 358, 127389 CrossRef CAS PubMed.
- S. S. A. Shah, L. De Simone, G. Bruno, H. Park, K. Lee, M. Fabbricino, I. Angelidaki and K.-H. Choo, Quorum quenching, biological characteristics, and microbial community dynamics as key factors for combating fouling of membrane bioreactors, npj Clean Water, 2021, 4, 19 CrossRef.
- C. H. Nahm, D.-C. Choi, H. Kwon, S. Lee, S. H. Lee, K. Lee, K.-H. Choo, J.-K. Lee, C.-H. Lee and P.-K. Park, Application of quorum quenching bacteria entrapping sheets to enhance biofouling control in a membrane bioreactor with a hollow fiber module, J. Membr. Sci., 2017, 526, 264–271 CrossRef CAS.
- S. H. Lee, S. Lee, K. Lee, C. H. Nahm, H. Kwon, H. S. Oh, Y. J. Won, K. H. Choo, C. H. Lee and P. K. Park, More Efficient Media Design for Enhanced Biofouling Control in a Membrane Bioreactor: Quorum Quenching Bacteria Entrapping Hollow Cylinder, Environ. Sci. Technol., 2016, 50, 8596–8604 CrossRef CAS PubMed.
- S. Lee, S. K. Park, H. Kwon, S. H. Lee, K. Lee, C. H. Nahm, S. J. Jo, H. S. Oh, P. K. Park, K. H. Choo, C. H. Lee and T. Yi, Crossing the Border between Laboratory and Field: Bacterial Quorum Quenching for Anti-Biofouling Strategy in an MBR, Environ. Sci. Technol., 2016, 50, 1788–1795 CrossRef CAS PubMed.
- L. Seonki, L. Sang Hyun, L. Kibaek, K. Hyeokpil, N. Chang Hyun, L. Chung-Hak, P. Pyung-Kyu, C. Kwang-Ho and L. Jung-Kee, Effect of the Shape and Size of Quorum-Quenching Media on Biofouling Control in Membrane Bioreactors for Wastewater Treatment, J. Microbiol. Biotechnol., 2016, 26, 1746–1754 CrossRef PubMed.
- S. Min, H. Lee, D. Chae, J. Park, S. H. Lee, H.-S. Oh, K. Lee, C.-H. Lee, S. Chae and P.-K. Park, Innovative Biofouling Control for Membrane Bioreactors in Cold Regions by Inducing Environmental Adaptation in Quorum-Quenching Bacteria, Environ. Sci. Technol., 2022, 56, 4396–4403 CrossRef PubMed.
- W.-S. See-Too, R. Ee, M. Madhaiyan, S.-W. Kwon, J. Y. Tan, Y. L. Lim, P. Convey, D. Pearce, W. F. Yin and K.-G. Chan, Planococcus versutus sp. nov., isolated from soil, Int. J. Syst. Evol. Microbiol., 2017, 67, 944–950 CrossRef CAS PubMed.
- W. S. See-Too, R. Ee, Y.-L. Lim, P. Convey, D. A. Pearce, W.-F. Yin and K.-G. Chan, AidP, a novel N-Acyl homoserine lactonase gene from Antarctic Planococcus sp, Sci. Rep., 2017, 7, 1–11 CrossRef PubMed.
- G. A. O'Toole, Microtiter dish biofilm formation assay, J. Visualized Exp., 2011, 2437 Search PubMed.
- K. Lee, S. Lee, S. H. Lee, S.-R. Kim, H.-S. Oh, P.-K. Park, K.-H. Choo, Y.-W. Kim, J.-K. Lee and C.-H. Lee, Fungal Quorum Quenching: A Paradigm Shift for Energy Savings in Membrane Bioreactor (MBR) for Wastewater Treatment, Environ. Sci. Technol., 2016, 50, 10914–10922 CrossRef CAS PubMed.
-
K. S. Sudhir and T. S. Rao, An Improved Crystal Violet Assay for Biofilm Quantification in 96-Well Microtitre Plate, bioRxiv, 2017, preprint, p. 100214, DOI:10.1101/100214.
- K. Lee, S. S. Shah, H. Park, C.-H. Lee and K.-H. Choo, Layered Antibiofouling Composite Membrane for Quenching Bacterial Signaling, Membranes, 2022, 12, 296 CrossRef CAS PubMed.
- T. Kawaguchi, Y. P. Chen, R. S. Norman and A. W. Decho, Rapid screening of quorum-sensing signal N-acyl homoserine lactones by an in vitro cell-free assay, Appl. Environ. Microbiol., 2008, 74, 3667–3671 CrossRef CAS PubMed.
- H. Zhang, X. Jie, Y. Yang, W. Zixing and Y. Fenglin, Mechanism of calcium mitigating membrane fouling in submerged membrane bioreactors, J. Environ. Sci., 2009, 21, 1066–1073 CrossRef CAS PubMed.
- T. Masuko, A. Minami, N. Iwasaki, T. Majima, S.-I. Nishimura and Y. C. Lee, Carbohydrate analysis by a phenol–sulfuric acid method in microplate format, Anal. Biochem., 2005, 339, 69–72 CrossRef CAS PubMed.
- M. M. Bradford, A rapid and sensitive method for the quantitation of microgram quantities of protein utilizing the principle of protein-dye binding, Anal. Biochem., 1976, 72, 248–254 CrossRef CAS PubMed.
- Z. Zhang, R. Cao, L. Jin, W. Zhu, Y. Ji, X. Xu and L. Zhu, The regulation of N-acyl-homoserine lactones (AHLs)-based quorum sensing on EPS secretion via ATP synthetic for the stability of aerobic granular sludge, Sci. Total Environ., 2019, 673, 83–91 CrossRef CAS PubMed.
- W. S. See-Too, P. Convey, D. A. Pearce and K.-G. Chan, Characterization of a novel N-acylhomoserine lactonase, AidP, from Antarctic Planococcus sp, Microb. Cell Fact., 2018, 17, 1–14 CrossRef PubMed.
- A. U. A. Arif, M. T. Sorour and S. A. Aly, Cost analysis of activated sludge and membrane bioreactor WWTPs using CapdetWorks simulation program: Case study of Tikrit WWTP (middle Iraq), Alexandria Eng. J., 2020, 59, 4659–4667 CrossRef.
- N. A. Weerasekara, K.-H. Choo and C.-H. Lee, Hybridization of physical cleaning and quorum quenching to minimize membrane biofouling and energy consumption in a membrane bioreactor, Water Res., 2014, 67, 1–10 CrossRef CAS PubMed.
- T. Iqbal, S. S. A. Shah, K. Lee and K.-H. Choo, Porous shell quorum quenching balls for enhanced anti-biofouling efficacy and media durability in membrane bioreactors, Chem. Eng. J., 2021, 406, 126869 CrossRef CAS.
|
This journal is © The Royal Society of Chemistry 2024 |
Click here to see how this site uses Cookies. View our privacy policy here.