Separation behavior of hydrogen isotopes via water pervaporation using proton conductive membranes†
Received
23rd April 2024
, Accepted 24th August 2024
First published on 5th September 2024
Abstract
In this study, we investigated pervaporative hydrogen isotope separation behaviors in proton-conductive membranes. Perfluorosulfonic acid (Nafion) and polybenzimidazole membranes exhibited similar hydrogen isotope separation factors, with varying water permeation fluxes based on membrane type and thickness. Increasing temperature improved water permeation flux, while the H/D separation factor remained unaffected. The highest H/D separation factor (1.086) was achieved with a single layer of Nafion at reduced vacuum, surpassing the 16O/18O separation factor (1.015). The observed H/D separation behavior is attributed to the mobility difference between hydrons (H+ and D+) rather than bulk water diffusion (H3O+ and H2DO+). Experiments with heavy metal-exchanged Nafion membranes suggested a negligible contribution of direct H/D ion exchange of sulfonic acid to the overall H/D separation factor. Additionally, water pervaporation through two membranes increased the H/D separation factor.
Water impact
This paper explores membrane pervaporation for the fractionation and separation of water isotopologues. Understanding their behavior is crucial for environmental water cycle comprehension, as well as for the development of technologies for tritiated wastewater treatment and heavy water production. This study enhances knowledge of isotopic behavior during membrane pervaporation and aids the development of water isotopologue separation technologies.
|
Introduction
The technology for separating hydrogen isotopes is becoming increasingly important not only for the recovery or upgrading of valuable hydrogen isotopes but also for the treatment of radioactive wastewater. The hydrogen isotopes deuterium (D) and tritium (T) are widely used in various fields such as energy, medicine, and semiconductors.1,2 Demand for deuterium is growing worldwide due to its ability to increase the lifespan of semiconductors and OLEDs.3 In addition, deuterium and tritium are essential fuel materials for nuclear fusion, the future of energy generation, making it crucial to secure a stable supply.1 Moreover, the separation of hydrogen isotopes is also important for the purification of water contaminated with the radioactive isotope tritium.4 Because tritium can have serious health and environmental consequences, the efficient and safe removal of this isotope from contaminated water is necessary.5
Nevertheless, the efficient separation of hydrogen isotopes is challenging because they have similar physicochemical properties. In particular, separating water isotopologues, such as H2O/HDO or H2O/HTO, is more difficult than separating gas phase isotopic mixtures such as H2/HD/D2 because of the small differences in molecular weight among H2O, HDO, and HTO.6,7 As a result, there has been active research on converting water into hydrogen gas and then utilizing hydrogen isotope separation technologies rather than the direct separation of water isotopologues. Recent studies have demonstrated that quantum sieving techniques using microporous materials can effectively separate hydrogen isotopes in the gas phase at cryogenic temperatures.8–10 Furthermore, a combined process involving water electrolysis and catalytic exchange has been developed to enrich heavier isotopes in water through the hydrogen isotope exchange between water and hydrogen gas.11–13 However, the aforementioned technologies require energy-intensive electrolysis to hydrogenate the water, which undermines the economic viability of these processes.
Water distillation technology, which relies on differences in vapor pressure among water isotopologues, enables the separation of such isotopologues without the energy-intensive process of water hydrogenation.6,14 For this reason, water distillation technology has been widely adopted for upgrading low-grade heavy water and water detritiation.15 However, due to the low separation factor of this process, it requires a large number of stages as well as significant energy consumption for heating.6 To address these issues, membrane distillation technologies utilizing a separation membrane have recently emerged as a promising and viable alternative.16–18 Unlike conventional water distillation, membrane distillation can operate at relatively low temperatures, offers the ability to increase separation factors by controlling the physicochemical properties of the membrane.16,19
The permeability of heavier water is lower than that of lighter water across a variety of organic and inorganic membranes.20–25 In early studies, pervaporation-based isotopic water separation was performed using organic membranes such as cellulose acetate (CA), polytetrafluoroethylene (PTFE), and polyphosphazene (PPOP) membranes.26–29 Recently, isotopic water separation using inorganic membranes such as zeolite and graphene oxide has been attempted. Using silico alumino phosphate (SAPO-34) membranes substituted with cations such as K, Li, Co, and Ba, a H/D separation factor of ∼1.023 was observed.21 Graphene oxide (GO) membranes have long diffusion pathways and a variety of oxygen-containing functional groups such as –OH, which have different binding energies for hydrogen isotopes.22,23 Therefore, by utilizing the difference in diffusion and adsorption properties among water isotopologues in the microchannels and maximizing the separation performance through a long diffusion path, an improved separation factor of H/D = 1.22 was achieved.22 Furthermore, addition of 2D materials such as boron nitride to GO membranes was found to enhance the separation performance of isotopic water.25 However, the flux (∼0.03 kg m−2 h−1) of this separation membrane based on vapor permeation was significantly lower than that of organic membranes based on pervaporation (3–6 kg m−2 h−1) such as CA and PTFE membranes.25 More recently, various attempts have been made to increase hydrogen isotope separation performance by introducing hydrophobic compounds and nanoparticles into GO membranes.24,25 Nevertheless, the mechanism underlying the membrane separation of water isotopologues remains unclear, despite various proposed isotope effects related to proton transport, water diffusion, surface sorption, and D/H bond stability within the membrane. For the technical improvement of water isotopologue separation using membrane technologies, understanding the isotope effect on water transport in various types of membranes is an important subject to investigate. Although various membranes have been used to investigate the separation of water isotopes, the study of isotopic effects on pervaporative water transport in hydrogen-conducting polymeric membranes has received little attention.
In the present study, we investigated the pervaporative separation behavior of water isotopologues through proton-conductive membranes under various conditions and explored the underlying separation mechanism. Although proton conductive membranes, such as perfluorosulfonic acid (Nafion) membranes, have been successfully applied to the pervaporative separation of various mixtures (e.g., alcohol/water and acid/water),30–32 to our knowledge no studies have examined their application to isotopic water separation. Moreover, proton conductive membranes allow us to explore various factors that contribute to pervaporative H/D separation, including the transport of hydrons via the Grotthuss mechanism, bulk diffusion of water molecules in the form of hydronium, and ion exchange at functional groups (such as sulfonic acid).33 In addition to the widely used Nafion membrane, the proton conductive polybenzimidazole (PBI) membrane was investigated. Since it is known that proton-exchangeable functional groups of Nafion and PBI, such as sulfonate and benzimidzole groups, can induce significant zero-point energy differences between hydrogen isotope,34,35 water pervaporation through these two types of proton conductive membranes was investigated.
Results and discussion
Comparison of membrane for H/D separation
The pervaporation experimental apparatus (Fig. 1) described in the Experimental section was used to evaluate the hydrogen and oxygen isotope separation factor of Nafion and PBI membranes from a deionized water feed at 35 °C. The initial deionized water had D/H and 18O/16O ratios of 147.57 ± 0.10 and 1988.61 ± 0.11 μmol mol−1, respectively. The permeation of water through the membrane in the pervaporation cell resulted in a decrease in the concentration of deuterium in the permeate, leading to a decrease in the D/H ratio to 143.5–144.5 μmol mol−1. The average H/D separation factors for the tested membranes are listed in Table 1.
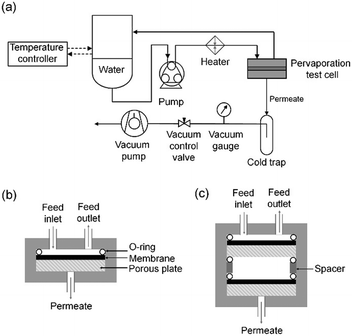 |
| Fig. 1 (a) Schematic of the membrane pervaporation apparatus and the structure of the assembled pervaporation test cell with (b) a single layer of membrane and (c) a double layer of membrane for demonstration of isotopic water separation. | |
Table 1 H/D and 16O/18O separation factors and water permeation fluxes (J) of different membranes as determined by pervaporation of deionized water at 35 °C
Membrane |
Thickness/dry (μm) |
Thickness/wet (μm) |
β (H/D) |
β (16O/18O) |
J (kg m−2 h−1) |
PBI |
39.9 ± 0.9 |
40.4 ± 0.7 |
1.026 ± 0.002 |
1.000 ± 0.000 |
0.10 ± 0.05 |
N212 |
50.1 ± 0.7 |
52.8 ± 1.0 |
1.020 ± 0.004 |
1.000 ± 0.000 |
15.64 ± 3.64 |
N117 |
179.1 ± 3.8 |
193.4 ± 3.4 |
1.026 ± 0.002 |
1.001 ± 0.000 |
5.04 ± 1.71 |
Despite N212 and PBI membranes having comparable thicknesses, the H/D separation factor of the PBI membrane was slightly higher than that of the N212 membrane. Previous reports indicate that the difference in zero point energy between H and T bound PBI (3–3.8 kcal mol−1)34 is higher than that of Nafion (∼2 kcal mol−1),35 which is predicted to be the source of the difference in the separation factor. The thicker N117 membrane exhibited a similar H/D separation factor to the PBI membrane. This H/D separation factor was slightly higher than that of the thinner N212 membrane. Specifically, the H/D separation factor increased from 1.020 ± 0.004 to 1.026 ± 0.002 when the thickness of the dried Nafion was increased from 50.1 ± 0.7 μm (N212) to 179.1 ± 3.8 μm (N117). This difference can be attributed to the short diffusion path of water molecules and hydrons in the N212 membrane, which limited the separation of H and D. Our findings are in line with previous results showing a non-linear increase in the separation factor of pervaporation membranes with increasing membrane thickness.36
The separation of 16O/18O during the pervaporation process was also analyzed. The 18O/16O ratio in deionized water (1988.61 ± 0.11 μmol mol−1) was higher than the D/H ratio in the same deionized water (147.57 ± 0.10 μmol mol−1). These oxygen isotopes are contained in chemical species such as H2O, H3O+, and OH− in the water phase. The change in the 18O/16O ratio during pervaporation using the PBI, N212, or N117 membrane was negligible, and the 16O/18O separation factor was found to be in the range of 0.9997–1.0008 (Table 1). These results indicate that, whereas these membranes could separate hydrogen isotopes at meaningful levels, the separation of oxygen isotopes was negligible.
Although the Nafion and PBI membranes exhibited similar H/D separation factors, the water permeation flux varied considerably depending on the thickness and type of membrane. The water permeation flux was calculated by measuring the amount of water collected in the cold trap during the pervaporation test (Fig. 1a), as detailed in the experimental section. PBI exhibited a very low flux, while N117 and N212 showed high water permeation fluxes (Table 1). This behavior can be attributed to the very low swelling of non-acid-doped PBI membranes exposed to water.37 The water permeation flux of N212 (15.6 ± 3.6 kg m−2 h−1) was 3.1 times higher than that of N117 (5.0 ± 1.7 kg m−2 h−1). This indicates that the water permeation flux is proportional to the thickness of the Nafion membrane, demonstrating that the permeation flux is highly dependent on the membrane thickness.38 Due to the low water permeation flux of PBI, subsequent experiments were conducted only on the Nafion membranes.
During the pervaporation process, a pressure gradient of water is created between the feed side and the permeate side of the membrane by the continuous removal of permeate by a vacuum pump (Fig. 2). This gradient acts as a driving force for water transport through the membrane.39 In addition to this pressure-driven water transport, protons can migrate in proton conductive membranes by i) hopping between water network and ionic clusters (i.e. sulfonic acid, imidazole) based on the Grotthuss mechanism and ii) bulk diffusion of hydrated hydronium clusters (H3O+(H2O)n) based on the vehicle mechanism.33
 |
| Fig. 2 Mechanisms of hydrogen and oxygen isotope transport and separation in proton conductive membranes. | |
The kinetic isotope effect, which is influenced by the difference in mass between isotopologues, is known to be directly proportional to the square root of the mass ratio between substances,40,41i.e.,
where
D represents the diffusivity and
m represents the mass of a substance (1 or 2). Consequently, the isotope effect between transport of H
+ and D
+ through the Grotthuss mechanism is approximately 1.4 (the square root of 2/1), while that between the transport of H
3O
+ and H
2DO
+ through vehicular bulk diffusion is approximately 1.026 (the square root of 20/19).
41,42
Although oxygen atoms bound to water molecules can only be transported across membranes through the vehicle mechanism, the separation factor for oxygen isotopes for all three membranes tested in the present work was close to 1, which was significantly smaller than the expected isotope effect (D(H3O+)/D(H2DO+) = 1.026). This finding implies that the isotope effect resulting from bulk diffusion based on the vehicle mechanism was not observed under the experimental conditions (∼1.3 × 102 m−1 s−2), indicating that the contribution of bulk diffusion to H/D separation was negligible. The H/D separation observed in the membranes is therefore believed to be primarily due to differences in mobility among hydrons.
Effect of vacuum level on H/D separation
To investigate the separation behavior in relation to the level of vacuum, the H/D and 16O/18O separation factors were measured for the N117 membrane while adjusting the vacuum level on the vacuum side at 25 °C (Table 2). The results revealed that the H/D and 16O/18O separation factors increased as the vacuum level changed from ∼1.3 × 102 to 8.0 × 104 kg m−1 s−2. Although the 16O/18O separation factor was 1.001 ± 0.000 (negligible uncertainty) at 1.3 × 102 kg m−1 s−2, it reached 1.015 ± 0.005 at 8.0 × 104 kg m−1 s−2. This finding indicates that the isotope effect resulting from bulk diffusion in the water channel of the membrane does not contribute significantly to the separation at very large pressure gradient (vacuum level = 1.3 × 102 kg m−1 s−2), likely due to the rapid suction of water molecules towards the vacuum side by pressure-driven water transport. However, at small pressure gradient (vacuum level = 8.0 × 104 kg m−1 s−2), bulk diffusion contributes to the isotope effect and separation of oxygen isotopes.
Table 2 H/D and 16O/18O separation factors and water permeation fluxes (J) of N117 at various vacuum levels, as determined by pervaporation of deionized water at 25 °C
Vacuum level (kg m−1 s−2) |
β (H/D) |
β (16O/18O) |
J (kg m−2 h−1) |
1.3 × 102 |
1.026 ± 0.001 |
1.001 ± 0.000 |
3.92 ± 1.47 |
4.0 × 104 |
1.053 ± 0.008 |
1.011 ± 0.001 |
0.58 ± 0.21 |
8.0 × 104 |
1.086 ± 0.013 |
1.015 ± 0.005 |
0.22 ± 0.12 |
Meanwhile, the H/D separation factor increased from 1.026 ± 0.001 to 1.086 ± 0.013 as the vacuum level changed from 1.3 × 102 to 8.0 × 104 kg m−1 s−2. This improvement is not only attributed to the contribution of the isotope effect (approximately 1.015) resulting from the bulk diffusion of hydronium but is also predicted to be due to the facilitated transport of protons and deuterons as the water content and water connectivity in the Nafion increases.43 In particular, the H/D separation factor exceeds the theoretically achievable value for vehicular bulk diffusion of hydronium (=1.026), indicating that the isotope effect of hydron transport via the Grotthuss mechanism contributed significantly to H/D separation by N117. This result aligns with the previous finding that the Grotthus mechanism dominates the isotope effect in proton/deuteron transfer in Nafion, as determined by nuclear magnetic resonance diffusometry.41
As expected, the water permeation flux through the N117 membrane decreased with decreasing vacuum, with a very low permeation flux of 0.22 ± 0.12 kg m−2 h−1 at 8.0 × 104. Due to the low water permeation flux at low vacuum, subsequent experiments were conducted at 1.3 × 102 kg m−1 s−2.
Effect of H/D exchange of sulfonic acid on separation
To investigate the contribution of proton exchange on sulfonic acid to the H/D separation factor of Nafion-based water pervaporation, the H/D separation performances of metal-exchanged N117 membranes were determined after 2 hours of water pervaporation at 35 °C (Fig. 3). Because heavy metals such as Fe, Co, and Ni selectively bind to sulfonic acids in Nafion over hydrogen, it was assumed that they would interfere with the protonation of sulfonic acid.44,45 Hydrated metal ions in Nafion can facilitate proton transport through the Grotthuss mechanism by providing water connectivity in certain cases, although the bulk transport of water molecules can be restricted by the limited mobility of hydrated metal ions and strong binding of water molecules to metal ions.46–48
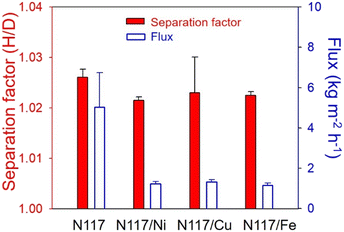 |
| Fig. 3 H/D separation factor and water permeation flux of cation-exchanged N117 membranes obtained by pervaporation of deionized water for 2 hours at 35 °C. | |
Immersion of the N117 membrane in heavy metal solution caused the water permeation flux of the membrane to decrease to only 74% to 77% of that of the pristine N117. By contrast, the metal-exchanged N117 membranes exhibited separation factors that were only 0.3–0.5% lower than that of the pristine N117; specifically, the separation factors of N117/Ni, N117/Cu, and N117/Fe were 1.0215 ± 0.001, 1.0230 ± 0.007, and 1.0225 ± 0.001, respectively. Assuming that this decrease is due to differences in the binding stability of H or D on the sulfonic acid groups of Nafion resulting from ion exchange, the effect of ion exchange on H/D separation is not significant.
Effect of pervaporation temperature and acidic pretreatment of Nafion
Variations of the H/D separation factor and water permeation flux as a function of temperature were analyzed in the range from 25 to 45 °C using the N212 and N117 membranes (Fig. 4). To investigate the effect of Nafion pretreatment, water pervaporation experiments were also performed using a N117 membrane that had been sequentially pretreated with hydrogen peroxide and sulfuric acid (designated H-N117). All of the tested membranes exhibited a slight decrease in the H/D separation factor within the marginal errors when the temperature was increased from 25 to 45 °C. The N117 pretreated with acid (H-N117) showed a H/D separation factor comparable to that of the pristine N117 in the experimental temperature range (Fig. 4a).
 |
| Fig. 4 (a) The H/D separation factor and (b) the water permeation flux of N212, N117, and acid-pretreated N117 (H-N117) determined by pervaporation of deionized water for 2 hours at temperatures ranging from 25 to 45 °C. | |
In contrast to the H/D separation factor, the water permeation flux was found to increase substantially with temperature. N117 and H-N117 showed similar variations in water permeation flux at each temperature, with the water permeation fluxes of both of these membranes increasing from approximately 4 to 7 kg m−2 h−1 as the temperature was raised from 25 to 45 °C (Fig. 4b). The results indicate that the acid pretreatment of Nafion had no discernible effect on the H/D separation factor or water permeation flux. Similar to N117, the water permeation flux of N212 increased substantially, from 11.7 ± 1.2 to 21.7 ± 5.1 kg m−2 h−1, as the temperature was raised from 25 to 45 °C. These findings are in line with previous results showing a positive correlation between temperature and water permeation through Nafion membranes.31,38 Although water permeation increased with increasing temperature, the change in the H/D separation factor was negligible.
Time-dependent pervaporative H/D separation in Nafion
To evaluate the water pervaporation behavior of N212 and N117 over time at 35 °C, pervaporation tests were paused every 2 hours, and the collected permeates were recovered for analysis of the H/D separation factor and water permeation flux. To prevent changes in the D/H ratio on the feed side, a sufficient amount of fresh deionized water was continuously supplied to the water reservoir. As shown in Fig. 5, N212 and N117 maintained comparable H/D separation factors over 6 hours, indicating no change in the separation factor under conditions of constant D/H ratio in the feed. On the other hand, a slight decline in the water permeation flux was observed following each 2 hour interval of water pervaporation. For example, the water permeation flux of N212 decreased from 15.6 (0–2 hours) to 13.5 kg m−2 h−1 (4–6 hours). This decline may be due to the evaporation of water from the initially hydrated membrane influencing the permeation flux during the initial stages of water permeation.
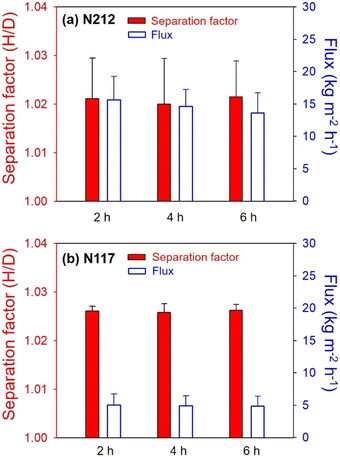 |
| Fig. 5 H/D separation factor and water permeation flux of (a) N212 and (b) N117 determined by pervaporation of deionized water at 35 °C at 2 hour intervals. | |
To examine the changes in membrane properties after the pervaporation test, the through-plane proton conductivity and tensile strength of the membranes were analyzed following a 6 hour water pervaporation test at 35 °C. Before the test, the fully hydrated N212 and N117 membranes exhibited proton conductivities of 32.2 ± 4.1 and 32.6 ± 5.1 mS cm−1, respectively, at 25 °C (Fig. 6a). After the pervaporation test, these values were measured to be 33.2 ± 4.6 and 31.9 ± 3.8 mS cm−1 for N212 and N117, respectively. Proton conductive membranes typically show changes in proton conductivity when contamination occurs.44,45 The negligible changes in membrane proton conductivity imply that fouling of the membranes during the pervaporation test was insignificant.
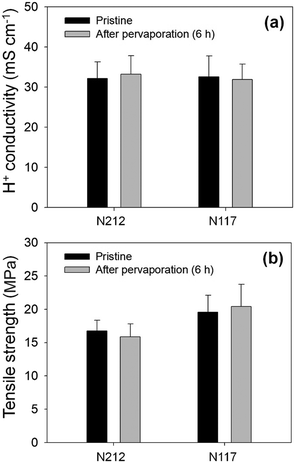 |
| Fig. 6 (a) Through-plane proton conductivities of the fully hydrated membranes at 25 °C and (b) tensile strength of membranes measured at ambient conditions before and after a water pervaporation test for 6 hours at 35 °C. | |
The tensile strength of dried N212 and N117 membranes before and after the pervaporation test was analyzed, and it was found that each membrane type maintained similar levels within the margin of error (Fig. 6b and S1†). Therefore, it can be concluded that the pressure gradient applied to the membranes during the pervaporation process under these experimental conditions does not significantly affect their mechanical properties.
Water pervaporation test with double layer membranes
Finally, the feasibility of sequential hydrogen isotope separation was explored by placing two layers of N117 membrane, spaced ∼2 cm apart, inside the pervaporation test cell (Fig. 1c). The feed side of the first membrane was in contact with excess water at 25 °C, whereas that of the second membrane was in contact with trace amounts of permeate that had already passed through the first membrane. The D/H ratio in the permeate obtained from the two layers of N117 was lower than the ratio in the permeate obtained from a single N117 membrane (Fig. 7). After the water underwent sequential permeation through two layers of N117, the D/H ratio decreased significantly from 147.57 ± 0.10 to 139.70 ± 1.22 μmol mol−1. These results correspond to a total separation factor of 1.056, indicating enhanced separation of hydrogen isotopes compared to a single layer of N117. The total separation factor was close to the square of the value of the H/D separation factor achieved through a single layer of N117 at 25 °C (1.026 ± 0.001).
 |
| Fig. 7 H/D separation factor and permeation flux obtained from water pervaporation through one or two layers of N117 at 25 °C for 2 hours. | |
In contrast to the increase in separation factor, the water permeation flux through two layers of N117 (0.10 ± 0.01 kg m−2 h−1) was considerably lower than that through a single layer of N117 (3.92 ± 1.47 kg m−2 h−1), as shown in Fig. 7. As the double-membrane pervaporation test was conducted by applying a vacuum to the permeate side of the second membrane, the reduced flux through the double-layered membrane may be due to an insufficient vacuum being applied to the first membrane, thereby limiting the overall performance. Therefore, a better approach may be to use a separation process that allows a vacuum to be applied across each membrane in a multimembrane system to increase the flux. The conceptualization and implementation of such a process warrants critical attention.
Experimental
Membrane materials and characterization
Nafion 117 (N117) and Nafion NR212 (N212) were purchased from DuPont. PBI membrane (Fumapem AM-40) was purchased from Fumatech. Acid-treated N117 (H-N117) was prepared by sequential treatment of N117 with aqueous solutions of H2O2 (3 wt%) and H2SO4 (1 M) at 65 °C, followed by rinsing with deionized water at the same temperature. The thickness of each membrane in a dried or fully hydrated state was measured using a thickness gauge (Mitutoyo) at 25 °C.
To obtain metal-exchanged N117, the membrane was immersed in a 1 M aqueous solution of NiCl2, CuCl2, or FeCl2 for 24 hours at room temperature. The resulting metal-exchanged membranes were rinsed by immersion in excess deionized water, with the water replaced 4 times per hour. The individual metal-exchanged membranes were labeled as N117/Ni, N117/Cu, and N117/Fe.
The proton conductivity of the fully hydrated membrane was evaluated using a ZIVE SP2 instrument (WonA Tech, South Korea) through electrochemical impedance analysis. This analysis was conducted at 25 °C using a custom-made through-plane conductivity cell equipped with platinum electrodes (active area: 1 × 1 cm2), following the procedure outlined in a previous study49 (refer to the ESI†).
The tensile test of the dried membrane (40 × 6 mm2) was conducted using a universal testing machine (Qmesys, South Korea) at ambient conditions. The displacement was adjusted at a rate of 10 mm min−1 to obtain the stress–strain curves.
Pervaporation experiment
Before conducting the pervaporation measurements, the membranes were hydrated by soaking overnight in deionized water and then dried using Kimtech wipers. Circular sheets of membrane of diameter 47 mm were prepared and mounted onto a porous steel support in a pervaporation test cell (PHILOS, South Korea). The effective area of the membrane, exclusive of the O-ring coverage, was determined to be 12.57 cm2. Deionized water (250 mL) was introduced into a temperature-controlled water bath and circulated in the pervaporation cell at a rate of 300 mL min−1 using a peristaltic pump (Fig. 1). The water flowed across one side of the membrane, while the other side was subjected to depressurization using a vacuum pump. The water that permeated the membrane was collected using an electrically controlled cold trap (−75 °C). The degree of vacuum in the downstream was controlled using a needle valve located between the cold trap and the vacuum pump, and the vacuum level was measured using a convection vacuum gauge (Super Bee, Instru Tech). The vacuum level was maintained at approximately 133 kg m−1 s−2 unless otherwise stated. The collected water in the cold trap was weighed after a specified time interval, and the permeation flux (J, kg m−2 h−1) was calculated using the following equation:
where m, A, and Δt represent the weight of the collected water in the cold trap, the effective area of the membrane, and the operation time, respectively.25,50 The operation time was typically set to 2 hours unless otherwise stated.
The D/H or 18O/16O ratio in both the feed and permeated water was analyzed using cavity ring-down spectroscopy (CRDS, L2140-i, Picarro). The manufacturer-guaranteed precision (1σ) levels of the instrument for D/H and 18O/16O in water are 0.015 and 0.049 μmol mol−1, respectively. Stable isotope ratio reference materials (SLAP2, VSMOW2, GRESP, and IAEA 604) were procured from the IAEA and utilized to calibrate the analytical values.51 To minimize the memory effect of previous measurements, each sample was measured 8 times, and the average of the last 3 measurements was used. The 18O/16O and D/H ratios in deionized water were measured to be 1988.61 ± 0.11 and 147.57 ± 0.10 μmol mol−1, respectively.
The H/D separation factor, β(H/D), was determined using the following equation:
where (D/H)
f and (D/H)
p are the molar ratios of deuterium and hydrogen in the feed and permeated water, respectively.
25
To determine the 16O/18O separation factor for mechanism study, β(16O/18O), the following equation was used:
where (
18O/
16O)
f and (
18O/
16O)
p represent the molar ratios of oxygen isotopes in the feed and permeated water, respectively.
All experiments were performed at least three times under the same conditions, and the experimental values are expressed as mean ± standard deviation. The error bars in the graphs represent the standard deviation.
Conclusions
In conclusion, this study analyzed the pervaporative separation behaviors of hydrogen isotopes in proton conductive membranes (Nafion and PBI) and investigated the underlying separation mechanism. The H/D separation factor achieved by single-layer Nafion and PBI membranes via water pervaporation ranged from 1.020 to 1.026 at 1.3 × 102 kg m−1 s−2. The Nafion membranes demonstrated higher water permeation fluxes than did previously reported membranes, such as graphene oxide and PTFE membranes;25,27 however, the PBI membrane exhibited a low flux due to its limited swelling by water without acid doping. Furthermore, the study showed that pervaporation had a negligible effect on the oxygen isotope ratio, indicating the bulk diffusion of oxygen-containing molecules (i.e., hydronium) had little effect on H/D separation. The H/D separation factor increased to 1.086 when the vacuum was reduced to minimize pressure-driven transport of water, surpassing the predicted isotope effect arising from the vehicular transport of hydronium (=1.026). The significant H/D separation observed using the Nafion membranes was attributed primarily to the difference in mobility between protons and deuterons resulting from water-mediated hydron hopping based on the Grotthuss mechanism. Based on analyses of heavy metal-exchanged Nafion membranes, the contribution of direct H/D ion exchange of sulfonic acid to the overall H/D separation factor was estimated to be less than 0.5%. The H/D separation by Nafion could be increased by increasing the thickness of the membrane, and the water permeation flux could be enhanced by increasing the operating temperature. The present results provide valuable insights into the factors that affect the separation performance of pervaporation membranes. These insights can be used to inform future efforts to design and optimize such membranes for various applications including deuterium or tritium separation.
Data availability
All data is available in the main manuscript and ESI.†
Author contributions
Chan Woo Park: writing – original draft, funding acquisition, conceptualization Sung-Wook Kim: conceptualization, validation Hyung-Ju Kim: methodology, writing – review & editing Euna Jeong: validation, investigation In-Ho Yoon: data curation.
Conflicts of interest
There are no conflicts to declare.
Acknowledgements
This work was supported by grants from the National Research Foundation of Korea (NRF), funded by the Korean government, Ministry of Science and ICT (RS-2022-00155422), and from the Korea Evaluation Institute of Industrial Technology, funded by Korean government, Ministry of Trade, Industry and Energy (Grant No. 20022479).
Notes and references
- M. Glugla, R. Lässer, L. Dörr, D. K. Murdoch, R. Haange and H. Yoshida, The inner deuterium/tritium fuel cycle of ITER, Fusion Eng. Des., 2003, 69, 39–43 CrossRef CAS
.
- J. Atzrodt, V. Derdau, W. J. Kerr and M. Reid, Deuterium- and Tritium-Labelled Compounds: Applications in the Life Sciences, Angew. Chem., Int. Ed., 2018, 57, 1758–1784 CrossRef CAS PubMed
.
- K. Cheng, J. Lee and J. W. Lyding, Approach to enhance deuterium incorporation for improved hot carrier reliability in metal-oxide-semiconductor devices, Appl. Phys. Lett., 2000, 77, 2358 CrossRef CAS
.
- A. N. Bukin, S. A. Marunich, Y. S. Pak, I. L. Rastunova, M. B. Rozenkevich and A. Y. Chebotov, Specific features and current status of processes for tritium removal from water: a critical review, Fusion Sci. Technol., 2022, 78, 595–606 CrossRef
.
- M. F. Ferreira, A. Turner, E. L. Vernon, C. Grisolia, L. Lebaron-Jacobs, V. Malard and A. N. Jha, Tritium: Its relevance, sources and impacts on non-human biota, Sci. Total Environ., 2023, 162816 CrossRef CAS PubMed
.
-
G. Vasaru, Tritium Isotope Separation, CRC Press, 1st edn, 1993 Search PubMed
.
- J. Noh, A. M. Fulgueras, L. J. Sebastian, H. G. Lee, D. S. Kim and J. Cho, Estimation of thermodynamic properties of hydrogen isotopes and modeling of hydrogen isotope systems using Aspen Plus simulator, J. Ind. Eng. Chem., 2017, 46, 1–8 CrossRef CAS
.
- J. Y. Kim, H. Oh, H. R. Moon, J. Y. Kim, H. R. Moon and H. Oh, Hydrogen isotope separation in confined nanospaces: carbons, zeolites, metal–organic frameworks, and covalent organic frameworks, Adv. Mater., 2019, 31, 1805293 CrossRef PubMed
.
- J. Ren, W. Zeng, Y. Chen, X. Fu and Q. Yang, In silico screening and experimental study of anion-pillared metal-organic frameworks for hydrogen isotope separation, Sep. Purif. Technol., 2022, 295, 121286 CrossRef CAS
.
- X. Z. Chu, Z. P. Cheng, Y. J. Zhao, J. M. Xu, M. S. Li, L. Zhou and C. H. Lee, Adsorption dynamics of hydrogen and deuterium in a carbon molecular sieve bed at 77 K, Sep. Purif. Technol., 2015, 146, 168–175 CrossRef CAS
.
- K. A. Mistry, N. S. Shenoy, K. Bhanja, D. K. Kohli and K. T. Shenoy, Modeling and experimental investigation for development of Combined Electrolysis and Catalytic Exchange process for hydrogen isotope separation, Chem. Eng. Res. Des., 2023, 192, 487–499 CrossRef CAS
.
- N. Zeng, C. Hu, C. Lv, A. Liu, L. Hu, Y. An, P. Li, M. Chen, X. Zhang, M. Wen, K. Chen, Y. Yao, J. Cai and T. Tang, Large-current density and high-durability proton exchange membrane water electrolysis for practical hydrogen isotope separation, Sep. Purif. Technol., 2023, 310, 123148 CrossRef CAS
.
- R. Wang, F. Xin, X. Chen and X. Xia, Simulation of the catalyzed isotopic exchange between hydrogen and water in a trickle bed reactor, Chem. Eng. Sci., 2017, 171, 179–188 CrossRef CAS
.
- Z. Zhang, Z. Zhang, X. Zhang, Z. Wang, F. Wang, G. Li, X. Zhang, X. Wang and Q. Liu, Effect of hydrophilic properties of packings on mass transfer performance of water distillation, Chem. Eng. Sci., 2023, 269, 118486 CrossRef CAS
.
- V. D. Trenin, I. A. Alekseev, I. A. Baranov, S. D. Bondarenko, S. N. Chernoby, G. A. Sukhorukova, O. A. Fedorchenko and V. V. Uborski, Full-Scale Experimental Studies of the Various Type Mass Exchange Packings by Water Distillation, Fusion Technol., 1995, 28, 1579–1584 CrossRef CAS
.
- N. Thomas, M. O. Mavukkandy, S. Loutatidou and H. A. Arafat, Membrane distillation research & implementation: Lessons from the past five decades, Sep. Purif. Technol., 2017, 189, 108–127 CrossRef CAS
.
- M. K. Alsebaeai and A. L. Ahmad, Membrane distillation: Progress in the improvement of dedicated membranes for enhanced hydrophobicity and desalination performance, J. Ind. Eng. Chem., 2020, 86, 13–34 CrossRef CAS
.
- H. Fareed, K. Jang, W. Lee, I. S. Kim and S. Han, Dehydroxylation-assisted self-crosslinking of MXene-based pervaporation membranes for treating high-salinity water, J. Ind. Eng. Chem., 2023, 119, 506–515 CrossRef CAS
.
- H. J. Kim, S. J. Kim, K. Lee and R. I. Foster, A short review on hydrophobic pervaporative inorganic membranes for ethanol/water separation applications, Korean J. Chem. Eng., 2022, 39, 2263–2274 CrossRef CAS
.
-
D. A. Nelson, J. B. Duncan, G. A. Jensen and S. D. Burton, Separation of HTO from water using membrane technology, Proceedings of the Canadian Nuclear Society. 15 Annual Conference, Montreal, PQ, Jun 5–8, 1994 Search PubMed.
-
R. Bhave, R. T. Jubin and B. Spencer, Investigation of sorption and diffusion mechanisms, and preliminary dconomic analysis,Oak Ridge National Lab. (ORNL), Oak Ridge, TN (United States), Report number: ORNL/TM-2017/59 Search PubMed.
-
G. J. Sevigny, R. K. Motkuri, D. W. Gotthold, L. S. Fifield, A. P. Frost and W. Bratton, Separation of Tritiated Water Using Graphene Oxide Membrane, Richland, WA (United States), 2015 Search PubMed
.
- A. Mohammadi, M. R. Daymond and A. Docoslis, New insights into the structure and chemical reduction of graphene oxide membranes for use in isotopic water separations, J. Membr. Sci., 2022, 659, 120785 CrossRef CAS
.
- M. Wen, M. Chen, K. Chen, P. L. Li, C. Lv, X. Zhang, Y. Yao, W. Yang, G. Huang, G. K. Ren, S. J. Deng, Y. K. Liu, Z. Zheng, C. G. Xu and D. L. Luo, Superhydrophobic composite graphene oxide membrane coated with fluorinated silica nanoparticles for hydrogen isotopic water separation in membrane distillation, J. Membr. Sci., 2021, 626, 119136 CrossRef CAS
.
- M. Wen, M. Chen, G. K. Ren, P. L. Li, C. Lv, Y. Yao, Y. K. Liu, S. J. Deng, Z. Zheng, C. G. Xu and D. L. Luo, Enhancing the selectivity of hydrogen isotopic water in membrane distillation by using graphene oxide, J. Membr. Sci., 2020, 610, 118237 CrossRef CAS
.
- A. G. Chmielewski, G. Zakrzewska-Trznadel, N. R. Miljevic and W. A. van Hook, Investigation of the separation factor between light and heavy water in the liquid/vapor membrane permeation process, J. Membr. Sci., 1991, 55, 257–262 CrossRef CAS
.
- A. G. Chmielewski, G. Zakrzewska-Trznadel, N. R. Miljevic and W. A. Van Hook, 16O/18O and H/D separation factors for liquid/vapor permeation of water through an hydrophobic membrane, J. Membr. Sci., 1991, 60, 319–329 CrossRef CAS
.
- J. B. Duncan and D. A. Nelson, The separation of tritiated water using supported polyphosphazene membranes, J. Membr. Sci., 1999, 157, 211–217 CrossRef CAS
.
- D. Sun, R. Li, M. Wen, X. Zhang, M. Chen, H. Yang, D. Guan, C. Xu and G. Zhang, Hydrogen isotopic water separation in membrane distillation through BN, MoS2 and their heterostructure membranes, Sep. Purif. Technol., 2023, 314, 123634 CrossRef CAS
.
- S. Kato, K. Nagahama and H. Asai, Permeation rates of aqueous alcohol solutions in pervaporation through Nafion membranes, J. Membr. Sci., 1992, 72, 31–41 CrossRef CAS
.
- C. J. Orme and F. F. Stewart, Pervaporation of water from aqueous sulfuric acid at elevated temperatures using Nafion® membranes, J. Membr. Sci., 2009, 326, 507–513 CrossRef CAS
.
- R. H. Elder, G. H. Priestman and R. W. K. Allen, Dewatering of HIx solutions by pervaporation through Nafion® membranes, Int. J. Hydrogen Energy, 2009, 34, 6129–6136 CrossRef CAS
.
- M. Phonyiem, S. Chaiwongwattana, C. Lao-Ngam and K. Sagarik, Proton transfer reactions and dynamics of sulfonic acid group in Nafion®, Phys. Chem. Chem. Phys., 2011, 13, 10923–10939 RSC
.
- B. S. Jorgensen, R. C. Dye, L. R. Pratt, M. A. Gomez and J. E. Meadows, Concentrating low-level tritiated water through isotope exchange, Fusion Technol., 2017, 37, 124–130 CrossRef
.
- M. Lozada-Hidalgo, S. Hu, O. Marshall, A. Mishchenko, A. N. Grigorenko, R. A. W. Dryfe, B. Radha, I. V. Grigorieva and A. K. Geim, Sieving hydrogen isotopes through two-dimensional crystals, Science, 2016, 351, 68–70 CrossRef CAS PubMed
.
- S. Claes, P. Vandezande, S. Mullens, K. De Sitter, R. Peeters and M. K. Van Bael, Preparation and benchmarking of thin film supported PTMSP-silica pervaporation membranes, J. Membr. Sci., 2012, 389, 265–271 CrossRef CAS
.
- J. Escorihuela, A. García-Bernabé and V. Compañ, A deep insight into different acidic additives as doping agents for enhancing proton conductivity on polybenzimidazole membranes, Polymers, 2020, 12, 1374 CrossRef CAS PubMed
.
- Q. Duan, H. Wang and J. Benziger, Transport of liquid water through Nafion membranes, J. Membr. Sci., 2012, 392–393, 88–94 CrossRef CAS
.
-
J. G. Crespo and C. Brazinha, Fundamentals of pervaporation, Pervaporation, in Vap. Permeat. Membr. Distill. Princ. Appl., 2015, pp. 3–17 Search PubMed
.
-
H. L. Friedman, Isotope Effects Upon Motions in Liquids in the Classical Limit, in Molecular Motions in Liquids, ed. J. Lascombe, Springer, Dordrecht, 1974, pp. 87–96 Search PubMed
.
- A. F. Privalov, E. Galitskaya, V. Sinitsyn and M. Vogel, Isotope Effect on Diffusion in Nafion Studied by NMR Diffusometry, Appl. Magn. Reson., 2020, 51, 145–153 CrossRef CAS
.
- N. Agmon, The Grotthuss mechanism, Chem. Phys. Lett., 1995, 244, 456–462 CrossRef CAS
.
- T. A. Zawodzinski, C. Derouin, S. Radzinski, R. J. Sherman, V. T. Smith, T. E. Springer and S. Gottesfeld, Water Uptake by and Transport Through Nafion® 117 Membranes, J. Electrochem. Soc., 1993, 140, 1041–1047 CrossRef CAS
.
- M. J. Kelly, G. Fafilek, J. O. Besenhard, H. Kronberger and G. E. Nauer, Contaminant absorption and conductivity in polymer electrolyte membranes, J. Power Sources, 2005, 145, 249–252 CrossRef CAS
.
- T. Okada, Y. Ayato, M. Yuasa and I. Sekine, The Effect of Impurity Cations on the Transport Characteristics of Perfluorosulfonated Ionomer Membranes, J. Phys. Chem. B, 1999, 103, 3315–3322 CrossRef CAS
.
- K. Kawai, T. Mabuchi and T. Tokumasu, Molecular simulation of proton conductivity in nafion membrane contaminated with ferrous ion, ECS Trans., 2015, 69, 579–586 CrossRef CAS
.
- G. Zhang, G. Yang, Q. Shen, S. Li, Z. Li, J. Liao, Z. Jiang, H. Wang, H. Zhang and W. Ye, Study on the transport performance degradation of Nafion membrane due to the presence of Na+ and Ca2+ using molecular dynamics simulations, J. Power Sources, 2022, 542, 231740 CrossRef CAS
.
- K. Kawai, T. Mabuchi and T. Tokumasu, Numerical analysis of proton conductivity in hydrated Nafion membrane contaminated with iron ion, ECS Trans., 2014, 64, 789–792 CrossRef CAS
.
- T. Kim, Y. Sihn, I. H. Yoon, S. J. Yoon, K. Lee, J. H. Yang, S. So and C. W. Park, Monolayer Hexagonal Boron Nitride Nanosheets as Proton-Conductive Gas Barriers for Polymer Electrolyte Membrane Water Electrolysis, ACS Appl. Nano Mater., 2021, 4, 9104–9112 CrossRef CAS
.
- H. J. Kim, S. J. Kim, S. Hyeon, H. H. Kang and K. Y. Lee, Application of desalination membranes to nuclide (Cs, Sr, and Co) separation, ACS Omega, 2020, 5, 20261–20269 CrossRef CAS PubMed
.
- S. de Graaf, H. B. Vonhof, E. J. Levy, M. Markowska and G. H. Haug, Isotope ratio infrared spectroscopy analysis of water samples without memory effects, Rapid Commun. Mass Spectrom., 2021, 35, e9055 CrossRef CAS PubMed
.
|
This journal is © The Royal Society of Chemistry 2024 |
Click here to see how this site uses Cookies. View our privacy policy here.