Effects of chloride transport on the bioelectrochemical remediation of nitrate-contaminated groundwater†
Received
23rd April 2024
, Accepted 15th August 2024
First published on 4th September 2024
Abstract
Nitrate is a common groundwater contaminant, primarily caused by the leaching of fertilizers. It poses a risk to human health, prompting the USEPA to set a drinking water limit of 10 mg L−1. Membrane-based bioelectrochemical systems (MBES) are effective treatment mechanisms for remediation of nitrate-rich groundwater. However, there is a knowledge gap surrounding how chloride ions as competing ions impact nitrate removal mechanisms and kinetics. In this study, nitrate-rich groundwater was fed into the cathode side of an MBES equipped with an anion exchange membrane (AEM). Nitrate ions were subsequently transported to the anolyte, where microbe-mediated reduction to N2 was achieved. The system performance was evaluated under varied catholyte nitrate and chloride concentrations as well as with different applied current densities. The MBES consistently achieved nitrate removal efficiencies of at least 85% with catholyte nitrate concentrations ranging from 14 mg L−1 NO3−-N to 56 mg L−1 NO3−-N. Notably, the highest nitrate removal rate of 8.28 ± 0.01 mg NO3−-N L−1 h−1 was achieved when the catholyte influent nitrate concentration was 56 mg L−1 NO3−-N. The nitrate removal behavior in the MBES can be characterized as a pseudo-first-order reaction. The presence of chloride ions, acting as model competing ions to nitrate, was found to decrease the rate of nitrate removal. Additionally, we found that diffusion is the primary driving force for nitrate removal, with electromigration slightly enhancing nitrate transport across the membrane in the MBES. When actual groundwater was used as the catholyte, 90.6 ± 12.1% nitrate was removed and the removal rate reached 5.3 ± 0.4 mg L−1 h−1 NO3−-N, demonstrating the high efficiency of this MBES in treating nitrate-contaminated groundwater.
Water impact
Nitrate (NO3−) contamination is a growing concern for groundwater due to the intensive application of fertilizer for agricultural activities. When treating nitrate-rich groundwater in membrane-based bioelectrochemical systems (MBES), there is a knowledge gap in understanding how competing ions impact the nitrate removal mechanism and kinetics. This article investigated the impact of chloride ions as competing ions on the removal kinetics of NO3− from groundwater. We identified the limiting factors in NO3− removal in the MBES. The system demonstrated a high efficiency in treating nitrate-contaminated groundwater.
|
1. Introduction
Groundwater, accounting for more than 98% of the self-sourced domestic drinking water in the US, is a crucial freshwater resource.1 However, contamination of groundwater resources due to both natural processes and human activities remains a key issue. Leaching from use of fertilizers and domestic sewage contamination frequently threaten groundwater quality.2 Furthermore, overexploitation of available groundwater resources due to increases in population and effects of human-driven climate change endanger the groundwater supply.3 Inorganic contaminants, such as nitrate, arsenic, fluoride, and metals, are the most frequent pollutants found in groundwater.4 Nitrate (NO3−) is the most widespread contaminant in groundwater, largely attributed to the extensive use of nitrate-based fertilizers in agriculture.5 NO3− indirectly jeopardizes human health in its potential reduction to nitrite within the digestive system, and studies have suggested a link between nitrate presence in drinking water and colorectal cancer.6 Moreover, it has also been reported that adverse reproductive outcomes, such as low infant birth weight and body length, are related to nitrate exposure in drinking water during pregnancy.7 When exposed to elevated levels of NO3−, infants are susceptible to developing methemoglobinemia, a dangerous condition where excessive nitrate in the bloodstream impedes the blood's oxygen-carrying capacity.8,9 To mitigate the adverse health impacts of nitrate, the United States Environmental Protection Agency (US EPA) has established an enforceable maximum contaminant level (MCL) of 10 mg L−1 as NO3−-N.10 However, according to the 2020 Wisconsin Groundwater Coordinating Council Report to the Legislature, more than 42
000 private wells in the state exceed the MCL for nitrate.
Conventional technologies for nitrate removal from groundwater include reverse osmosis (RO) and ion exchange processes. Reverse osmosis, a pressure-driven membrane process, is capable of rejecting ionic compounds while allowing the transport of water molecules through the membrane.11,12 Ion exchange processes employ strongly basic anion exchange resins to selectively remove nitrate ions from the influent.13 However, both RO and ion exchange are separation-based technologies which create concentrated waste streams that incur expensive disposal costs. Biological denitrification, which employs anaerobic microorganisms to reduce nitrate into nitrogen gas, is widely used in wastewater treatment as an effective method. However, biological denitrification requires a high concentration of organic molecules in the water matrix, as the microorganisms require these organic, carbon-based molecules as electron donors. Biological denitrification of groundwater is challenging, as this drinking water source typically contains very low concentrations of organics that could serve as these electron donors.
Bioelectrochemical systems (BES), which combine the principles of microbiology and electrochemistry to treat wastes and produce value-added products, have been applied to convert nitrate to nitrogen gas (N2) by heterotrophic or autotrophic denitrification for nitrate-contaminated groundwater.14–18 In such systems, electrons are generated through oxidation of organic matter at the anode and subsequently used by denitrifying bacteria for nitrate reduction in the anode or cathode.19 A potential issue when using BES for nitrate remediation, particularly when treating streams with high organics concentrations like wastewater, is the generation of undesirable compounds during the oxidation of complex organic matter at the anode, such as BES using a biocathode to treat groundwater. Previous studies using BES to treat nitrate in groundwater have used a biocathode configuration with a cation exchange membrane (CEM) separating the electrode chambers.20,21 Although it shows a great removal ability, the existence of microbes in the chamber may add difficulty in post treatment for the separation of microbes and treated water especially when applied in decentralized rural areas.22 To address this concern, an anion exchange membrane (AEM) instead of a cation exchange membrane (CEM) is incorporated into the BES to regulate the ion transport activity within the system.
When an AEM is positioned in the BES between the anode and the cathode compartments, anions such as nitrate can be transported from the catholyte to the anolyte. This transport is driven by the flow of electrons from the anode to the cathode and serves to maintain the charge balance within the system.23–26 Donnan dialysis, driven by the concentration gradient of ionic species, can also affect the nitrate movement due to the transport of other anions.25,27 For example, chloride ions, a common unreactive monovalent anion in groundwater, may either favor or impede the transportation of nitrate ions. Elevated chloride concentration in groundwater, which can exceed 700 mg L−1 for domestic wells, is often attributed to road de-icing and seawater intrusion.28,29 Studies found that the typical NO3−-N in ground water is less than 1 mg L−1, while polluted groundwater can reach concentrations as high as 43.6 mg L−1.30,31 Chloride is a relatively conservative (i.e. non-reactive) ion, particularly when compared to other common anions found in groundwater, such as sulfate and bicarbonate, which may undergo biological transformation or volatilization reactions.32 Chloride presence in nitrate-rich groundwater treated with AEM-based BES can present a problem due to the chloride ion's structural similarity to nitrate and affinity for transport across the AEM.
The functional group in typical AEMs is quaternized ammonium, which facilitates anion transport while blocking cations. Nitrate and chloride have similar hydrated radii of 3.40 Å and 3.32 Å, respectively, resulting in a low selectivity between these ions in conventional AEM.33,34 Chloride, as the main monovalent anion in groundwater, plays an important role in affecting nitrate removal and denitrification rate during the MBES process. Other BES, such as microbial desalination cells, were studied to treat nitrate-contaminated groundwater under high salinity.35,36 The system achieved a high nitrate and chloride removal efficiency with the coproduction of chlorine disinfectant.
In this study, we investigated the effect of chloride ion transport on nitrate removal in a membrane-based bioelectrochemical system (MBES). To evaluate the system performance, we assessed key system metrics including nitrate and COD removal rates as well as the pH and conductivity in both chambers under varying concentrations of nitrate in the catholyte. We explored the nitrate removal mechanisms by introducing synthetic groundwater with high nitrate concentrations into the catholyte and anolyte separately. The effect of chloride ions as competing ions was investigated under varying concentrations. To understand the anion transport dynamics in the MBES, the selectivity between nitrate and chloride ions was calculated. In addition, we fed real groundwater into the MBES system to demonstrate its robustness.
2. Experimental
MBES setup
A dual-chamber MBES (Fig. 1 and S1†) was constructed with rectangular polycarbonate plates separated by an anion exchange membrane (AXM-100, Membranes International Inc., NJ, USA). The total volumes of anolyte and catholyte are 500 mL and 600 mL, respectively. The solutions were continuously recirculated at 76 mL min−1. The cathode chamber was aerated with ambient air (Fig. S1†). The preparation procedure and size of the anode and cathode electrode are illustrated elsewhere.37 The anode compartment was inoculated with mesophilic anaerobic digestion sludge from Nine Springs Wastewater Treatment Plant (Madison, WI, USA), as described previously.37
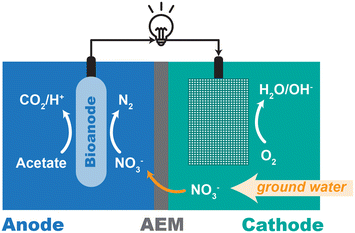 |
| Fig. 1 The schematic of membrane-based bioelectrochemical system (MBES) for nitrate removal from groundwater. | |
Experimental operation
Five different conditions of experiments were conducted using different nitrate (as mg N) and chloride concentrations, different current densities with and without chloride ions, and real groundwater as influent catholyte (Table S1†). Anolyte solutions in all experimental conditions consisted of 1 g L−1 sodium acetate, 10 mL L−1 stock solution, 10 mL L−1 trace solution, and 10 mL L−1 phosphate buffer solution (PBS), as described in Table S1.† The compositions of trace solution, phosphate buffer solution, and stock solution are described in Burns et al.37 To ensure anoxic conditions in the anode chamber, anolyte solutions were purged with N2 gas for 8–10 min immediately before use. Catholyte solutions varied through each experimental condition but always included 0.02 M PBS (Table S1†). The anolyte and catholyte were changed once the potential difference between electrodes dropped to 10 mV. This replacement process was defined as one cycle. Dry chemicals were from Carolina Chemical (Charlotte, NC, USA), Alfa Aesar (Thermo Fisher Scientific, Tewksbury, MA, USA), and Sigma-Aldrich (Merck Kraal, Darmstadt, Germany).
To explore the effect of nitrate concentration in the MBES system, different concentration gradients (14, 28, 42, 56 mg L−1 NO3−-N) were selected. Examining the influence of chloride concentration involved fixing the nitrate concentration at 28 mg L−1 NO3−-N while varying Cl− concentration between 0, 142, and 710 mg L−1. The investigation into the effect of current density, both with and without chloride in the influent, was conducted by adjusting the external resistance from 10 Ω to 470 Ω and infinite resistance conditions were employed with 28 mg L−1 NO3−-N and 0 or 710 mg L−1 of Cl−.
Measurement and analysis
The pH and conductivity of both the influent and effluent were measured via an electrode probe and benchtop meter (Orion Versa Star Pro, Thermo Fisher Scientific, Waltham, MA, USA). Cl− concentration was measured via an Orion Chloride Electrodes conductivity meter (Accumet Research AR50, Fisher Scientific, Waltham, MA, USA) or ion chromatography (Thermo Dionex 2100 Ion Chromatography System, Thermo Fisher Scientific, Waltham, MA, USA). The concentrations of nitrate and nitrite were measured by high-performance liquid chromatography which has a detection limit of 0.4 mg N L−1 (AT VP system, Shimadzu Scientific Instruments, Columbia, MD, USA).
All samples were filtered through a 0.45 μm PVDF membrane (HVLP02500, MilliporeSigma, USA) before testing. Chemical oxygen demand (COD) and ammonia nitrogen (NH3-N) were tested via standard colorimetric methods (COD Digestion Vials High Range, High Range Ammonia Nitrogen AmVer Salicylate Test 'N Tube, Hach, Loveland, CO, USA). Groundwater for the real groundwater experiments was sampled from an edge-of-field monitoring well adjacent to an agricultural field in Portage County, WI, that typically grows a rotation of potato–field corn–peas–sweet corn. The ions in groundwater were tested via inductively coupled plasma mass spectrometer (8900 triple quadrupole ICP-MS, Agilent, Santa Clara, CA, USA) and ion chromatography (Thermo Dionex 1100/2100, Thermo Scientific, USA).
Nitrate and COD removal efficiency (R) is calculated as:
|  | (1) |
where
ci is the initial concentration of COD in the anolyte or nitrate in the catholyte;
cf is the final concentration of COD in the anolyte or total nitrate in the anolyte and catholyte.
Coulombic efficiency is the ratio of produced charge to the theoretical total available charge from the substrate:
| 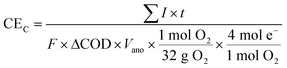 | (2) |
| 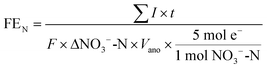 | (3) |
where CE
C is the coulombic efficiency based on acetate as substrate, FE
N is the Faradaic efficiency based on nitrate, ∑
I is the integration of current over the cycle,
t is the duration time of the cycle,
F is Faraday's constant (96
![[thin space (1/6-em)]](https://www.rsc.org/images/entities/char_2009.gif)
485 coulombs per mole of electrons), ΔCOD is the difference between initial and final COD concentrations,
Vano is the total volume of the anode liquid, ΔNO
3−-N (M) is the concentration difference of nitrate in the catholyte.
To characterize the performance of nitrate and chloride competitive behavior in the system, we used the selectivity of NO3− over Cl−,38 which is calculated as
|  | (4) |
where
ρ is the selectivity of NO
3− over Cl
−,
ci,0 is the initial concentration of
i (either NO
3− or Cl
−), Δ
ci is the difference between the calculated concentration and the initial concentration.
3. Results and discussion
3.1. Effect of NO3− concentrations
The MBES performance and nitrate removal in the absence of chloride ions was investigated by varying the nitrate concentrations in the catholyte from 14 to 56 mg L−1 NO3−-N. The maximum current density increased from 0.9 A m−2 to 1.8 A m−2 when the catholyte nitrate concentration increased from 14 to 42 mg L−1 NO3−-N (Fig. 2a). The total coulomb production achieved the peak value of 815 ± 282 C when the catholyte nitrate concentration was 28 mg L−1 NO3−-N. However, the maximum current density and total coulomb production were decreased to 1.0 A m−2 and 230 ± 24 C, respectively, when the catholyte nitrate concentration was 56 mg L−1 NO3−-N. These decreases are attributed to the competition between the current generation and the denitrification pathways for electrons generated at the anode. Higher NO3− concentration in the cathode compartment yields higher NO3− transport across the AEM to the anode chamber. In the anode chamber, NO3− is converted to nitrogen gas via the denitrification process. The denitrification process requires electrons and may compete with the anode electrode for electron uptake, which affects various aspects of MBES performance, such as current production, operating time, COD removal, and columbic efficiency. As the NO3−-N concentration increased from 14 to 42 mg L−1 NO3−-N, the operating time for each cycle decreased from 3 days to 18 hours, indicating an enhanced metabolism interaction between denitrifying bacteria and electricity-generating bacteria, leading to improved bioelectrochemical activity (Fig. 2a).39 This finding is aligned with previous studies using an air cathode treating nitrate-rich wastewater; denitrification improves the COD removal efficiency and as a result decreases the cycle duration time.40 The pH and conductivity values of the effluent from both anode and cathode chambers remained stable regardless of the varied catholyte nitrate concentration (Fig. S2†).
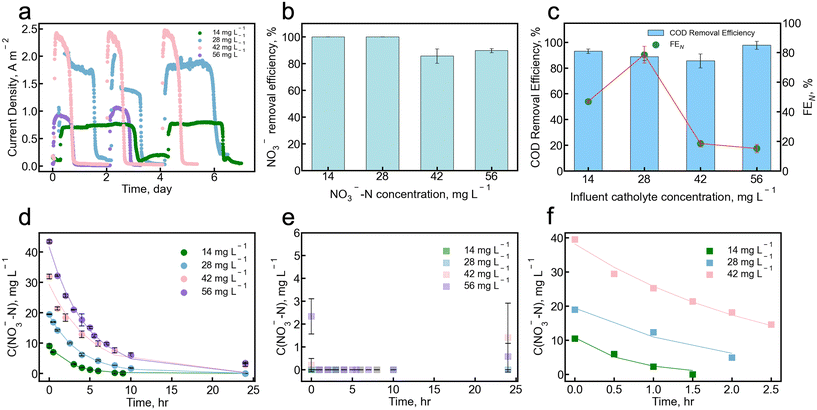 |
| Fig. 2 The system performance of the MBES fed with synthetic nitrate-rich waters in the catholyte. (a) Current density profiles as a function of time. (b) Nitrate removal efficiency. (c) COD removal efficiency and faradaic efficiency. (d) NO3−-N concentration in the catholyte. (e) NO3−-N concentration in the anolyte. (f) NO3−-N concentration in the anolyte when the synthetic nitrate-contaminated waters are fed in the anolyte. For (a), (d), and (e), the nitrate-rich water with NO3−-N concentration of 14 (green dots), 28 (blue dots), 42 (pink dots), and 56 mg L−1 (purple dots) is fed to the cathode compartment. The current density was normalized by the projected area of the cathode electrode. (f) NO3−-N concentration in the anolyte when the nitrate-rich water with NO3−-N concentration of 14 (green dots), 28 (blue dots), and 42 mg L−1 (pink dots) is fed to the anode compartment. For (c) and (d), the lines are simulated NO3− reduction at first-order and zero-order reaction for the whole system separately. | |
A nitrate removal efficiency of 100% from synthetic groundwater in the cathode was achieved when the initial concentration of NO3−-N was 14 and 28 mg L−1 NO3−-N (Fig. 2b). When the catholyte nitrate concentration was further increased to 42 and 56 mg L−1 NO3−-N, the nitrate removal efficiency was slightly decreased to 85.6 ± 5.4% and 89.7 ± 1.5%, respectively, likely due to the high NO3−-N concentration. In this high nitrate concentration scenario, there was not enough COD to provide sufficient electrons for the denitrification process, resulting in a lower removal efficiency. The MBES exhibited high COD removal efficiencies of over 88% in all nitrate concentration experiments (Fig. 2c). The highest faradaic efficiency based on nitrate (FEN) of 78.6 ± 5.7% was achieved when the catholyte nitrate concentration was 28 mg L−1 NO3−-N. As the catholyte nitrate concentration increased to 56 mg L−1 NO3−-N, the FEN decreased to 15.1 ± 2.8%, likely due to the shorter operating time per cycle brought on by the competition of the denitrification process for electron acceptance in the anode chamber.
Nitrate removal rate was found to increase as catholyte nitrate concentration increased, despite the decreased removal efficiencies achieved at these higher catholyte nitrate concentrations. As shown in Fig. 2d, unlike the biological denitrification process that is typically simplified into a zero-order kinetic model in simple batch reactors, the kinetics of nitrate removal in our MBES cathode follow a pseudo-first-order reaction model.41 The kinetics is modeled using the following first-order reaction equation:
| 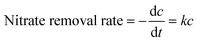 | (5) |
|  | (6) |
where
ci is the initial concentration of the nitrate in the catholyte,
ct is the concentration of the nitrate in the whole system at time
t, and
k is the reaction rate constant.
The nitrate removal rates were 2.96 ± 0.24, 4.77 ± 0.14, 5.40 ± 0.33, and 8.28 ± 0.01 mg L−1 NO3−-N h−1 when the initial catholyte nitrate concentrations were 14, 28, 42, and 56 mg L−1, respectively (Table S2†). To further confirm that the diffusion impacts the nitrate removal kinetics in the MBES, nitrate was introduced into the anolyte. The nitrate removal rate was as high as 7.78 ± 1.10 mg L−1 NO3−-N h−1 when the nitrate ions were introduced into the anolyte side with varied concentrations from 14 to 42 mg L−1 (Fig. 2f). At anolyte nitrate concentrations of 14, 28, and 42 mg L−1 NO3−-N, the nitrate removal rate is higher than that in the MBES with similar nitrate concentrations in the catholyte only. However, at a high anolyte nitrate concentration of 56 mg L−1 NO3−-N, the nitrate removal rate is lower than that achieved in the catholyte-only nitrate condition. This observation indicates that the limiting factor in overall nitrate removal may vary depending on the nitrate concentration in the influent catholyte. Nitrate removal in the MBES can be described in two steps: (1) transport across the AEM from catholyte to anolyte by diffusion and migration and (2) denitrification in the anode. When the nitrate concentration in the catholyte is relatively low, the transport of nitrate ions across the membrane may become the limiting step. This is evident as the overall nitrate removal rate was lower compared to the rate of simple denitrification in the anolyte. When the MBES has a higher catholyte nitrate concentration (over 56 mg L−1 NO3−-N), membrane transport is enhanced and the rate of denitrification at the anode becomes the limiting factor.
Another potential factor influencing the nitrate removal rates could be the COD/NO3−-N ratio under different nitrate concentrations. The COD/NO3−-N ratio is defined as the concentration ratio of COD in the anolyte influent and the NO3−-N concentration in the catholyte influent. Here, the overall COD/NO3−-N ratios were 42.8, 21.4, 14.3, and 10.7 when the catholyte NO3−-N concentration was 14, 28, 42 and 56 mg L−1 NO3−-N, respectively. Vijay et al. revealed that denitrification is most efficient when the COD/NO3−-N ratio is between 7 and 12.42 A lower COD/NO3−-N ratio in an anoxic environment enhances denitrification activity.43,44 As the COD in the anolyte is fixed in our study, a higher nitrate concentration results in a lower COD/NO3−-N ratio. A higher nitrate concentration in the catholyte increases the nitrate concentration in the anolyte side according to Fick's second law of diffusion, thus favoring the denitrification process.
Throughout our experiments, the anolyte nitrite concentration was consistently below 0.1 mg L−1 NO2−-N where the detection limit of the method is 0.4 mg L−1, indicating that all the nitrate removed was converted into nitrogen gas. The presence of nitrite and ammonium was not observed in the catholyte, indicating that electrochemical nitrate reduction at the cathode did not occur in our system. This absence can be attributed to both the properties of the Pt/C cathode electrode and the lower reduction potential of NO3−/NO2− compared with the oxygen reduction reaction (ORR) in this material. The current density in this MBES is not high enough to facilitate the completion of nitrate reduction on the Pt/C within 48 hours.45–47 As a result, the nitrate reduction in the cathode chamber is negligible.48
3.2. Effect of competing ions in synthetic groundwater
We also investigated the impact of chloride ions in the catholyte on nitrate removal by varying the chloride concentrations between 0 mg L−1, 142 mg L−1, and 710 mg L−1 (Fig. 3). These experiments explore the effects of varying chloride levels in groundwater on nitrate removal, as extensive use of road salts containing chloride during winter is common in many areas serviced by groundwater. For all experiments, the chloride concentration in the anolyte influent is fixed at around 500 mg L−1. Varying chloride concentration in the catholyte affected the current generation in the MBES; the maximum current density increased from around 1.8 A m−2 to 2.0 A m−2 when the catholyte chloride concentration increased from 142 to 710 mg L−1, respectively (Fig. S3†). The observed increase can be attributed to the higher conductivity in the catholyte, which reduces the internal resistance of the reactor and thus leads to a higher maximum current density (Fig. S4†). However, the total produced charges during each cycle were reduced by 24.2% when the catholyte chloride concentration increased from 142 to 710 mg L−1. CEc, the ratio of current production to charges calculated based on COD removal (eqn (2)), was observed to be the lowest with a high catholyte chloride concentration of 710 mg L−1. The COD removal efficiency remained consistent under different chloride influent concentrations (approximately 90%, Table S3†).49
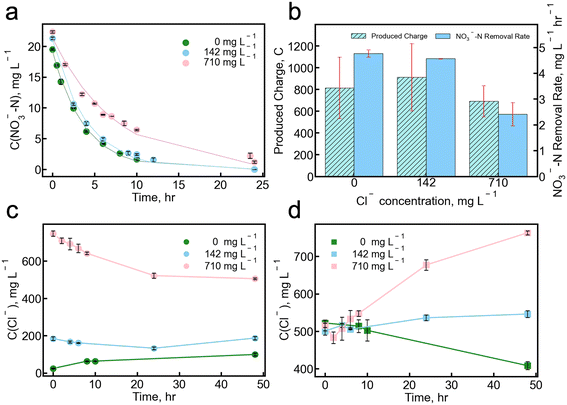 |
| Fig. 3 The system performance of the MBES fed with a chloride concentration of 0 (green dots), 142 (blue dots), and 710 mg L−1 (purple dots) in nitrate-rich waters as the catholyte. (a) Nitrate concentration in the catholyte as a function of time. Measured data are indicated as discrete points, and the solid line simulates the pseudo-first-order reaction for the whole system. (b) Produced charges and NO3− removal rate. (c) Chloride concentration profile in the catholyte as a function of time. (d) Chloride concentration profile in the anolyte. | |
The MBES achieved 100% nitrate removal efficiency at catholyte chloride concentrations of 0 and 142 mg L−1 (Fig. 3a). When the catholyte chloride concentration was increased to 710 mg L−1, the removal efficiency dropped slightly to 97.8 ± 2.2%. Even with chloride as a competing ion in the catholyte, the nitrate removal still follows a pseudo-first-order reaction model. The nitrate removal rate exhibited a minor decrease from 4.77 ± 0.14 mg L−1 NO3−-N h−1 to 4.57 ± 0.01 mg L−1 NO3−-N h−1 when the chloride concentration was increased from 0 to 142 mg L−1 in the catholyte influent. When the chloride concentration was 710 mg L−1, the nitrate removal rate was 2.41 ± 0.45 mg L−1 NO3−-N h−1, twice as low as that at the lower chloride concentrations. This suggests that, at high chloride concentrations, the transport of chloride ions competes with the transport of nitrate ions, leading to a decreased rate in nitrate removal (Fig. 3b).
The selectivity of nitrate to chloride in the catholyte was calculated to be −3.57 ± 0.02 and 3.42 ± 0.30 when the chloride concentration was 142 and 710 mg L−1, respectively. The negative selectivity value at 142 mg L−1 was due to the opposite transport direction (i.e., from anolyte to catholyte) of nitrate and chloride ions. As shown in Fig. 3c and d, when the initial chloride concentration was 0 mg L−1 in the catholyte influent, the chloride concentration in the anolyte decreased as a function of time, while it kept increasing in the catholyte. When the catholyte influent chloride concentration increased to 142 mg L−1, both anolyte and catholyte chloride concentrations slightly fluctuated. With a higher catholyte chloride concentration of 710 mg L−1, the chloride ions transported from the cathode compartment to the anode compartment, resulting in a concentration decrease in the catholyte and an increase in the anolyte. The initial influent chloride concentration in the anolyte was around 500 mg L−1. In contrast, the final anolyte chloride concentrations were 408.5 ± 10.5, 546 ± 29.0, and 762.9 ± 19.3 mg L−1 when the influent chloride concentrations were 0, 142, and 710 mg L−1, respectively.
The opposite transport of chloride ions at varying concentrations primarily resulted from Donnan dialysis, which is a process in which ions of the same charge move in opposite directions through an ionomer membrane driven by a concentration gradient.50,51 This synergistic effect leads to an enhanced nitrate removal rate, particularly in situations with lower chloride concentrations in the catholyte influent. We note that diffusion plays a more important role than migration in nitrate removal since migration becomes negligible when the current density decreases to zero, which will be further discussed in section 3.3.
3.3. Effect of current generation
The effect of current generation on the transport of nitrate ions and chloride ions was investigated by varying the external resistance of 10 Ω, 470 Ω, and infinite resistance both with and without the presence of chloride ions in the catholyte. Catholyte chloride concentrations of 0, 142, 710 mg L−1 were investigated, while the concentration of nitrate ions in the catholyte was set at 28 mg L−1 NO3−-N throughout the study.
In the absence of chloride in the influent catholyte, the maximum current density decreased from 2.0 to 0.24 A m−2 when the external resistance increased from 10 Ω to 470 Ω (Fig. S5†). The nitrate removal efficiency slightly decreased as the current density decreased, with 100% nitrate removal efficiency observed with 10 Ω external resistance and 95.4 ± 0.1% nitrate removal efficiency observed under open circuit (i.e. infinite resistance) conditions. As shown in Fig. 4a, the kinetics of nitrate removal still followed a pseudo-first-order reaction model. Similar to nitrate removal efficiency, the nitrate removal rate also decreased with decreases in current density. The lowest nitrate removal rate of 3.31 ± 0.34 mg L−1 NO3−-N h−1 was observed in the open circuit condition, which was 30.6% lower than that observed with 10 Ω external resistance, 4.77 ± 0.14 mg L−1 NO3−-N h−1. This indicates that while ion migration contributes to nitrate removal, its impact is relatively minor compared to the diffusion driven by the concentration gradient. This observation aligns well with previous BES studies showing that diffusion contributes more to ion transport than migration.52 The movement of nitrate ions from the catholyte to the anolyte under open circuit conditions indicates that the concentration gradient difference contributes to the removal process.12,13 Similar findings were observed in previous studies on air cathode MFCs, where denitrification efficiency was not influenced by external resistance.14 Consequently, the limiting factor of denitrification in this MBES configuration is the diffusion of nitrate ion. Nevertheless, ion migration can still enhance the overall nitrate removal process in our system.
 |
| Fig. 4 The system performance of the MBES fed with synthetic nitrate-rich waters under different external resistances and chloride concentrations, which includes high chloride concentration in the influent catholyte (10 Ω (dark blue dots), 470 Ω (dark green dots), infinite (pink dots)) and no chloride ions in the influent catholyte (10 Ω (light blue dots), 470 Ω (light green dots), infinite (rose pink dots)). (a) The nitrate removal rate of different external resistances with and without chloride in the catholyte. (b) The nitrate concentration profile of different external resistances with and without chloride in the catholyte. The lines simulate the nitrate removal as a first-order reaction in the whole system. | |
When chloride ions were present in the catholyte as competing ions, the maximum current density was observed when the external resistance increased (Fig. S6†). The highest nitrate removal efficiency and removal rate (97.8 ± 2.2% and 2.96 ± 0.53 mg L−1 NO3−-N h−1, respectively) were observed when the external resistance was 470 Ω. Interestingly, the transport of chloride ions was less with 470 Ω external resistance than that with 10 Ω external resistance (Fig. S7†). This decreased transport leads to a higher selectivity under 470 Ω external resistance, of 3.61 ± 0.24 compared to 3.42 ± 0.30 with 10 Ω external resistance. Under open circuit conditions (i.e. with no current generation), both the nitrate removal efficiency and the removal rate were observed to be the lowest (92.4 ± 1.1% and 2.18 ± 0.39 mg L−1 NO3−-N h−1, respectively), indicating that current generation enhances the nitrate removal rate, which was consistent with non-chloride conditions. Consequently, the selectivity under open circuit conditions was substantially higher than in closed circuit conditions, reaching as high as 6.44 ± 0.77. This increased selectivity can be attributed to the limited transport of chloride ions across the membrane in the open-circuit condition. There was less chloride ion transport, leading to a smaller difference between the initial and the final chloride concentrations, which ultimately contribute to an enhanced calculated selectivity. In regular abiotic membrane processes, the selectivity of chloride to nitrate in commercial AEM is usually around 1.34,53 Here, we conclude that removing the nitrate ions on the anode side enhanced the selectivity of nitrate to chloride.
When comparing the nitrate removal with and without chloride ions as competing ions, it is evident that the presence of chloride lowered the nitrate removal rate (Fig. 4b). Specifically, when chloride ions were present in the catholyte, the highest nitrate removal rate was 2.96 ± 0.53 mg L−1 NO3−-N h−1, which was achieved at the 470 Ω condition. In contrast, the lowest nitrate removal rate in the absence of chloride ions was 3.31 ± 0.34 mg L−1 NO3−-N h−1, achieved under open circuit conditions and higher than all conditions with chloride ions present in the solution. The concentration gradients of both nitrate and chloride ions across the membrane play a significant role in the system performance, as discussed in section 3.2. Denitrification acts as a crucial method of nitrate removal in the anolyte, maintaining a consistent nitrate concentration gradient within the system. When chloride ions are absent in the cathode compartment, the nitrate transport is notably enhanced by the opposite transport of chloride ions from anolyte to catholyte, underscoring the importance of Donnan dialysis for nitrate transport.54 However, when chloride ions are present with a higher concentration in the cathode compartment, they compete with nitrate ions for the transport across the membrane. We note that the effect of high current generation on the transport of chloride ions is more significant than for nitrate ions. Therefore, we observed a higher selectivity of nitrate to chloride with a lower current generation.
3.4. Nitrate removal from real groundwater
We also fed real groundwater in the cathode compartment of the MBES to test the system under real environmental conditions. The groundwater was collected from an edge-of-field monitoring well adjacent to an agricultural field in Portage County, WI, that typically grows a rotation of potato–field corn–peas–sweet corn. The pH and conductivity of the groundwater sample were 8.116 and 0.775 mS cm−1, respectively. The detailed composition of the groundwater, including concentrations of major ions, is listed in Table S5.† As shown in the table, Ca2+ and Mg2+ are the major cations (Table S5†). As for the anions, the concentrations of nitrate and chloride ions in the groundwater were 44.5 mg L−1 NO3−-N and 44.2 mg L−1, respectively. During the 3-cycle experiment, the MBES demonstrates consistent and stable performance (Fig. S8†). The COD removal efficiency achieved was 98.2 ± 3.1%, while the coulombic efficiency reached 6.2 ± 0.5%. The COD removal efficiency achieved with real groundwater is comparable to that achieved in the synthetic experiments when the nitrate concentration was 56 mg L−1. The low coulombic efficiency might be due to low conductivity in groundwater (Fig. 5a). Additionally, the low coulombic efficiency could also be due to the relatively high nitrate concentration discussed in section 3.1. The nitrate removal efficiency was 90.6 ± 12.1% and the nitrate removal rate was 5.3 ± 0.4 mg L−1 NO3−-N h−1 (Fig. 5b). The nitrate removal rate was slightly higher than that achieved with synthetic groundwater, which may be a result of the relatively low concentration of anions in groundwater compared with the anolyte solution, creating a large concentration gradient across the membrane.
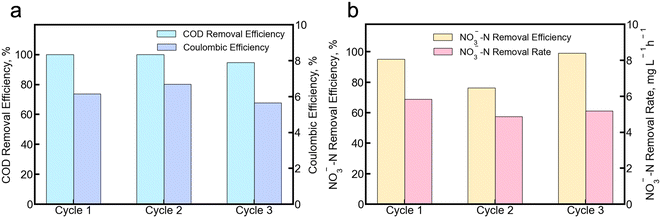 |
| Fig. 5 The system performance of MBES fed with real groundwater. a) COD removal efficiency and coulombic efficiency b) NO3−-N removal efficiency and removal rate. | |
4. Conclusions
In this work, we investigated the impact of ion competition on nitrate removal from contaminated groundwater using MBES. Chloride ions were used as the model competing ions in the system. The MBES achieved a nitrate removal efficiency of over 85% throughout the study. Results indicate that the nitrate removal in the MBES is a pseudo-first-order reaction, supporting the conclusion that ion transport across the AEM is the limiting step for nitrate remediation in the MBES. A high concentration of chloride in the catholyte (710 mg L−1) hindered the nitrate transport, resulting in a slower nitrate removal rate. In the absence of chloride ions, the nitrate removal rate decreased from 4.77 ± 0.14 mg L−1 NO3−-N h−1 to 3.31 ± 0.34 mg L−1 NO3−-N h−1 as the decreasing current generation went from 2.0 A m−2 to 0. When the concentrations of chloride in the anolyte and catholyte are similar, the transport of chloride between chambers is influenced by current density, thereby slightly affecting nitrate transport. The MBES consistently demonstrated stable performance, even when fed with real nitrate-rich groundwater, exhibiting 90.6 ± 12.1% nitrate removal efficiency and a nitrate removal rate of 5.3 ± 0.4 mg L−1 NO3−-N h−1, highlighting the efficacy of the system.
Data availability
The data supporting this article have been included as part of the ESI.†
Author contributions
Hanyu Tang: conceptualization, methodology, data curation, writing – original draft, visualization, investigation. McKenzie Burns: investigation and writing. Mohan Qin: conceptualization, methodology, resources, supervision, writing – review & editing, funding acquisition.
Conflicts of interest
The authors declare that they have no known competing financial interests or personal relationships that could have appeared to influence the work reported in this paper.
Acknowledgements
The authors would like to acknowledge the startup fund from the Department of Civil and Environmental Engineering, College of Engineering, the Office of the Vice-Chancellor for Research and Graduate Education (OVCRGE) at the University of Wisconsin-Madison, and the Wisconsin Alumni Research Foundation (WARF) for the support of this study. The authors gratefully acknowledge the support from Jackie Cooper of the Environmental Engineering Core Facility at the University of Wisconsin-Madison for the use of facilities and equipment. We also thank Dr. Kevin Masarik for providing the nitrate-contaminated groundwater samples to us.
References
-
C. A. Dieter, M. A. Maupin, R. R. Caldwell, M. A. Harris, T. I. Ivahnenko, J. K. Lovelace, N. L. Barber and K. S. Linsey, Estimated use of water in the United States in 2015: U.S. Geological Survey Circular 1441, 2017, DOI:10.3133/cir1441.
- N. M. Burri, R. Weatherl, C. Moeck and M. Schirmer, A review of threats to groundwater quality in the anthropocene, Sci. Total Environ., 2019, 684, 136–154 CrossRef CAS PubMed.
- C. Ren and Q. Zhang, Groundwater Chemical Characteristics and Controlling Factors in a Region of Northern China with Intensive Human Activity, Int. J. Environ. Res. Public Health, 2020, 17, 9126 CrossRef CAS PubMed.
- P. Li, D. Karunanidhi, T. Subramani and K. Srinivasamoorthy, Sources and Consequences of Groundwater Contamination, Arch. Environ. Contam. Toxicol., 2021, 80, 1–10 CrossRef CAS PubMed.
- K. Wick, C. Heumesser and E. Schmid, Groundwater nitrate contamination: Factors and indicators, J. Environ. Manage., 2012, 111, 178–186 CrossRef CAS PubMed.
- A. Temkin, S. Evans, T. Manidis, C. Campbell and O. V. Naidenko, Exposure-based assessment and economic valuation of adverse birth outcomes and cancer risk due to nitrate in United States drinking water, Environ. Res., 2019, 176, 108442 CrossRef CAS PubMed.
- V. R. Coffman, A. S. Jensen, B. B. Trabjerg, C. B. Pedersen, B. Hansen, T. Sigsgaard, J. Olsen, I. Schaumburg, J. Schullehner, M. Pedersen and L. T. Stayner, Prenatal Exposure to Nitrate from Drinking Water and Markers of Fetal Growth Restriction: A Population-Based Study of Nearly One Million Danish-Born Children, Environ. Health Perspect., 2021, 129, 027002 CrossRef CAS PubMed.
- L. Fewtrell, Drinking-Water Nitrate, Methemoglobinemia, and Global Burden of Disease: A Discussion, Environ. Health Perspect., 2004, 112, 1371–1374 CrossRef PubMed.
- A. Kapoor and T. Viraraghavan, Nitrate Removal From Drinking Water—Review, J. Environ. Eng., 1997, 123, 371–380 CrossRef CAS.
- U.S. Environmental Protection Agency, Drinking Water Regulations and Health Advisories, Office of Water, Washington, DC, 1995 Search PubMed.
- G. Kang and Y. Cao, Development of antifouling reverse osmosis membranes for water treatment: A review, Water Res., 2012, 46, 584–600 CrossRef CAS PubMed.
- L. Malaeb and G. M. Ayoub, Reverse osmosis technology for water treatment: State of the art review, Desalination, 2011, 267, 1–8 CrossRef CAS.
- H. He, Y. Huang, M. Yan, Y. Xie and Y. Li, Synergistic effect of electrostatic adsorption and ion exchange for efficient removal of nitrate, Colloids Surf., A, 2020, 584, 123973 CrossRef CAS.
- B. E. Logan and K. Rabaey, Conversion of Wastes into Bioelectricity and Chemicals by Using Microbial Electrochemical Technologies, Science, 2012, 337, 686–690 CrossRef CAS PubMed.
- W.-W. Li, H.-Q. Yu and Z. He, Towards sustainable wastewater treatment by using microbial fuel cells-centered technologies, Energy Environ. Sci., 2013, 7, 911–924 RSC.
- P. T. Kelly and Z. He, Nutrients removal and recovery in bioelectrochemical systems: A review, Bioresour. Technol., 2014, 153, 351–360 CrossRef CAS PubMed.
- D. Molognoni, M. Devecseri, D. Cecconet and A. G. Capodaglio, Cathodic groundwater denitrification with a bioelectrochemical system, J. Water Process Eng., 2017, 19, 67–73 CrossRef.
- S. Zou, L. Guan, D. P. Taylor, D. Kuhn and Z. He, Nitrogen removal from water of recirculating aquaculture system by a microbial fuel cell, Aquaculture, 2018, 497, 74–81 CrossRef CAS.
- P. Clauwaert, K. Rabaey, P. Aelterman, L. De Schamphelaire, T. H. Pham, P. Boeckx, N. Boon and W. Verstraete, Biological Denitrification in Microbial Fuel Cells, Environ. Sci. Technol., 2007, 41, 3354–3360 CrossRef CAS PubMed.
- D. Cecconet, M. Devecseri, A. Callegari and A. G. Capodaglio, Effects of process operating conditions on the autotrophic denitrification of nitrate-contaminated groundwater using bioelectrochemical systems, Sci. Total Environ., 2018, 613–614, 663–671 CrossRef CAS PubMed.
- N. Pous, S. Puig, M. Dolors Balaguer and J. Colprim, Cathode potential and anode electron donor evaluation for a suitable treatment of nitrate-contaminated groundwater in bioelectrochemical systems, Chem. Eng. J., 2015, 263, 151–159 CrossRef CAS.
- O. Modin and F. Aulenta, Three promising applications of microbial electrochemistry for the water sector, Environ. Sci.: Water Res. Technol., 2017, 3, 391–402 RSC.
- W. T. Mook, M. H. Chakrabarti, M. K. Aroua, G. M. A. Khan, B. S. Ali, M. S. Islam and M. A. Abu Hassan, Removal of total ammonia nitrogen (TAN), nitrate and total organic carbon (TOC) from aquaculture wastewater using electrochemical technology: A review, Desalination, 2012, 285, 1–13 CrossRef CAS.
- K. Lee, I. Choi and K. Lim, Nitrogen removal and electrochemical characteristics depending on separators of two-chamber microbial fuel cells, Environ. Eng. Res., 2018, 24, 443–448 CrossRef.
- A. Breytus, D. Hasson, R. Semiat and H. Shemer, Removal of nitrate from groundwater by Donnan dialysis, J. Water Process Eng., 2020, 34, 101157 CrossRef.
- H. Shemer, Y. Huang, D. Hasson and R. Semiat, Coupling donann dialysis and electro-reduction process for nitrate removal from simulated groundwater, Sep. Purif. Technol., 2022, 299, 121718 CrossRef CAS.
- M. Hichour, F. Persin, J. Molénat, J. Sandeaux and C. Gavach, Fluoride removal from diluted solutions by Donnan dialysis with anion-exchange membranes, Desalination, 1999, 122, 53–62 CrossRef CAS.
- K. W. F. Howard and P. J. Beck, Hydrogeochemical implications of groundwater contamination by road de-icing chemicals, J. Contam. Hydrol., 1993, 12, 245–268 CrossRef CAS.
-
G. E. Granato, L. A. DeSimone, J. R. Barbaro and L. C. Jeznach, Methods for evaluating potential sources of chloride in surface waters and groundwaters of the conterminous United States, Reston, VA, 2015, DOI:10.3133/ofr20151080.
- J. Luczaj and K. Masarik, Groundwater Quantity and Quality Issues in a Water-Rich Region: Examples from Wisconsin, USA, Resources, 2015, 4, 323–357 CrossRef.
- B. T. Nolan, C. T. Green, P. F. Juckem, L. Liao and J. E. Reddy, Metamodeling and mapping of nitrate flux in the unsaturated zone and groundwater, Wisconsin, USA, J. Hydrol., 2018, 559, 428–441 CrossRef CAS.
-
B. Wang, P. Strelakos and B. Jokela, Nitrate source indicators in ground water of the Scimitar Subdivision, Peters Creek area, Anchorage, Alaska, US Department of the Interior, US Geological Survey, 2000 Search PubMed.
- A. G. Volkov, S. Paula and D. W. Deamer, Two mechanisms of permeation of small neutral molecules and hydrated ions across phospholipid bilayers, Bioelectrochem. Bioenerg., 1997, 42, 153–160 CrossRef CAS.
- R. Epsztein, E. Shaulsky, M. Qin and M. Elimelech, Activation behavior for ion permeation in ion-exchange membranes: Role of ion dehydration in selective transport, J. Membr. Sci., 2019, 580, 316–326 CrossRef CAS.
- G. Puggioni, S. Milia, E. Dessì, V. Unali, N. Pous, M. D. Balaguer, S. Puig and A. Carucci, Combining electro-bioremediation of nitrate in saline groundwater with concomitant chlorine production, Water Res., 2021, 206, 117736 CrossRef CAS PubMed.
- X. Chen, P. Liang, X. Zhang and X. Huang, Bioelectrochemical systems-driven directional ion transport enables low-energy water desalination, pollutant removal, and resource recovery, Bioresour. Technol., 2016, 215, 274–284 CrossRef CAS PubMed.
- M. Burns and M. Qin, Ammonia recovery from organic nitrogen in synthetic dairy manure with a microbial fuel cell, Chemosphere, 2023, 325, 138388 CrossRef CAS PubMed.
- C. He, J. Ma, C. Zhang, J. Song and T. D. Waite, Short-Circuited Closed-Cycle Operation of Flow-Electrode CDI for Brackish Water Softening, Environ. Sci. Technol., 2018, 52, 9350–9360 CrossRef CAS PubMed.
- X. Jin, F. Guo, W. Ma, Y. Liu and H. Liu, Heterotrophic anodic denitrification improves carbon removal and electricity recovery efficiency in microbial fuel cells, Chem. Eng. J., 2019, 370, 527–535 CrossRef CAS.
- H. Huang, S. Cheng, J. Yang, C. Li, Y. Sun and K. Cen, Effect of nitrate on electricity generation in single-chamber air cathode microbial fuel cells, Chem. Eng. J., 2018, 337, 661–670 CrossRef CAS.
- C. Glass and J. Silverstein, Denitrification kinetics of high nitrate concentration water: pH effect on inhibition and nitrite accumulation, Water Res., 1998, 32, 831–839 CrossRef CAS.
- A. Vijay, M. Vaishnava and M. Chhabra, Microbial fuel cell assisted nitrate nitrogen removal using cow manure and soil, Environ. Sci. Pollut. Res., 2016, 23, 7744–7756 CrossRef CAS PubMed.
- J. C. Akunna, C. Bizeau and R. Moletta, Denitrification in anaerobic digesters: Possibilities and influence of wastewater COD/N-NOX ratio, Environ. Technol., 1992, 13, 825–836 CrossRef CAS.
- G. Ruiz, D. Jeison and R. Chamy, Development of denitrifying and methanogenic activities in USB reactors for the treatment of wastewater: Effect of COD/N ratio, Process Biochem., 2006, 41, 1338–1342 CrossRef CAS.
- C. Wang, J. Dong, W. Hu and Y. Li, Enhanced simultaneous removal of nitrate and perchlorate from groundwater by bioelectrochemical systems (BESs) with cathodic potential regulation, Biochem. Eng. J., 2021, 173, 108068 CrossRef CAS.
- C. Fang, B. Min and I. Angelidaki, Nitrate as an Oxidant in the Cathode Chamber of a Microbial Fuel Cell for Both Power Generation and Nutrient Removal Purposes, Appl. Biochem. Biotechnol., 2011, 164, 464–474 CrossRef CAS PubMed.
- M. Li, C. Feng, Z. Zhang, Z. Shen and N. Sugiura, Electrochemical reduction of nitrate using various anodes and a Cu/Zn cathode, Electrochem. Commun., 2009, 11, 1853–1856 CrossRef CAS.
- F. Harnisch and U. Schröder, From MFC to MXC: chemical and biological cathodes and their potential for microbial bioelectrochemical systems, Chem. Soc. Rev., 2010, 39, 4433 RSC.
- J.-Y. Nam, H.-W. Kim, K.-H. Lim, H.-S. Shin and B. E. Logan, Variation of power generation at different buffer types and conductivities in single chamber microbial fuel cells, Biosens. Bioelectron., 2010, 25, 1155–1159 CrossRef CAS PubMed.
-
W. Grot, Fluorinated Ionomers, William Andrew, 2011, pp. 81–156 Search PubMed.
-
B. Van Der Bruggen, in Fundamental Modelling of Membrane Systems, Elsevier, 2018, pp. 251–300 Search PubMed.
- P. Kuntke, M. Geleji, H. Bruning, G. Zeeman, H. V. M. Hamelers and C. J. N. Buisman, Effects of ammonium concentration and charge exchange on ammonium recovery from high strength wastewater using a microbial fuel cell, Bioresour. Technol., 2011, 102, 4376–4382 CrossRef CAS PubMed.
- L. Kong, E. Palacios, X. Guan, M. Shen and X. Liu, Mechanisms for enhanced transport selectivity of like-charged ions in hydrophobic-polymer-modified ion-exchange membranes, J. Membr. Sci., 2022, 658, 120645 CrossRef CAS.
- M. Rodrigues, T. Sleutels, P. Kuntke, D. Hoekstra, A. ter Heijne, C. J. N. Buisman and H. V. M. Hamelers, Exploiting Donnan Dialysis to enhance ammonia recovery in an electrochemical system, Chem. Eng. J., 2020, 395, 125143 CrossRef CAS.
|
This journal is © The Royal Society of Chemistry 2024 |
Click here to see how this site uses Cookies. View our privacy policy here.