Degradation of carbamazepine and sulfamethoxazole in water by dielectric barrier discharge plasma coupled with a far UV-C (222 nm) system†
Received
3rd July 2024
, Accepted 25th September 2024
First published on 25th September 2024
Abstract
Organic micropollutants (OMPs) are now frequently found in wastewater and pose a risk to human and environmental health. This study utilizes an in-house developed plasma source and KrCl* excilamp (far UV-C at 222 nm), a conventional UV (LPUV at 254 nm) lamp and the combinations of plasma with LPUV/far UV-C for the degradation of sulfamethoxazole (SMX) and carbamazepine (CBZ). At first, the concentrations of plasma-produced long-lived (such as H2O2, NO3−, NO2−) and short-lived (such as ·HO) reactive species have been quantified. Accordingly, the role of plasma-produced reactive species in the degradation of CBZ and SMX under UV 222 has been reported. In the case of DIW/plasma + UV 222, the complete degradation of CBZ and SMX only takes 15 and 10 minutes, respectively. Furthermore, the degradation rate considerably accelerated in TW, and CBZ and SMX completely degraded in just 12 and 8 minutes, respectively. Moreover, the degradation rate of CBZ in DIW is found to be 25 times higher when using plasma + UV 222 compared to using plasma + LPUV and 114 times higher compared to LPUV alone. This is due to the abundance of ·OH (46.7 × 10−8 M s−1) generated under UV 222 from plasma-produced reactive species. The required electrical energy per order for the OMP degradation from this hybrid process is relatively low (73.38 kW h m−3), which makes it an energy-efficient approach. This study provides a fresh perspective to broaden the application of plasma coupling with UV 222 for treating wastewater containing numerous OMPs.
Water impact
The combination of plasma and far UV-C radiation has proven to be a highly efficient and eco-friendly method for eliminating organic micropollutants in real water, such as carbamazepine and sulfamethoxazole. We have investigated the impact of plasma-generated reactive species on the degradation of organic micropollutants in water by exposure to far UV-C light, aiming to achieve an additive-free approach.
|
1. Introduction
Organic micropollutants (OMPs) are increasingly being detected in freshwater bodies and are causing concern due to their potentially harmful effects on aquatic life and human health.1,2 Due to the excretion of OMPs through bodily waste and the improper disposal of undesired or expired medications, a significant amount of these pollutants end up in sewage. Consequently, the disposal of untreated or inadequately treated wastewater is recognized as a primary contributor to the presence of these pollutants in environmental systems.3–7 KrCl* excilamp (UV 222 nm) based direct photolysis and advanced oxidation processes (AOPs) are being extensively researched as new methods for effectively removing micropollutants from industrial or municipal wastewater.8–10,11
According to a study by Xu et al.,8 nitrogenous OMPs have high molar absorption coefficients at 222 nm, but sulfonamides have low molar absorption coefficients at 222 nm compared to 254 nm. Hence, nitrogenous OMPs degrade faster under direct photolysis at 222 nm, but sulfonamides degrade faster at 254 nm. However, the time for the direct degradation of the micropollutants is relatively higher than that of KrCl*/AOP. Xin et al.12 examined the effectiveness of 222 nm far UV-C irradiation in the degradation and defluorination of 19 different per- and polyfluoroalkyl substances (PFASs). They found that perfluorocarboxylic acids, fluorotelomer unsaturated carboxylic acids, and GenX showed significantly enhanced photolysis under 222 nm irradiation compared to the conventional 254 nm source at a similar fluence. However, minimal decay was observed for other PFASs. Additionally, Yin et al.13 revealed that using far UV-C at 222 nm in a UV/peroxydisulfate (UV/PDS) AOP increased the fluence-based concentration of hydroxyl radicals (·HO) by 6.40, 2.89, and 6.00 times in deionized, tap, and surface water, respectively. A treatment process that can work on all types of OMPs, effectively, is being researched by integrating different treatment methods. During the UV/H2O2 AOP process, UV exposure to H2O2 with wavelengths below 300 nm results in the generation of ·HO.14 While the UV/H2O2 process is more efficient than UV photolysis, it still suffers an energy cost of 4–5 times greater than the results observed for ozonation15,16 Despite its attractive characteristics, the UV/H2O2 mechanism has major drawbacks. Initially, given the relatively low molar absorption coefficient of hydrogen peroxide (H2O2) at 254 nm, large amounts of H2O2 are necessary to produce ·HO effectively.17 In addition, while operating in real water samples, the generation of ·HO is even more restricted due to the presence of matrix components. Zhao et al.18 showed that the removal of disinfection by-products (DBPs) was more efficient in the UV/H2O2 AOP when using a 222 nm KrCl* excilamp compared to the conventional 254 nm UV/H2O2 AOP. The improved photolysis of 28 halogenated aromatic DBPs at 222 nm was largely attributed to the higher molar absorption coefficients of these compounds at this wavelength compared to 254 nm.
It is generally advised against using high concentrations of H2O2 (over 10 ppm) in most commercial applications because only a small fraction (5–10%) of H2O2 is consumed in the process. Additionally, a non-trivial H2O2 residue remains after treatment, which requires high chlorine demand after the AOP, and its toxicity towards different aquatic organisms is very high. It is, therefore, necessary to eliminate the remaining H2O2 after wastewater treatment. This can be achieved by combining it with biological activated carbon treatment, which further increases the treatment cost.19 To make the degradation process more environmentally friendly and additive free, dielectric barrier discharge (DBD) based non-thermal plasma (NTP) processes are being researched20,21 DBD is a type of NTP that can produce reactive oxygen and nitrogen species (RONS) such as O3, H2O2, ·HO, HO2·, O2·, 1O2, NO2−, and NO3− directly during electrical discharges22–24 However, like other AOPs, DBD shows relatively low mineralization efficiency of OMPs. While it is true that increasing the applied voltage and extending the treatment period will improve the mineralization of pollutants, it is important to note that the energy cost will also inevitably increase.
Many researchers have created a hybrid system that combines plasma with other catalysts, oxidants, and photons in order to get over the drawbacks of NTP, save energy, and improve the breakdown of organic wastes25,26 This has resulted in enhanced degradation of persistent organic compounds and improved efficiency in removing carbonaceous substances27,28 Still, this approach had restrictions regarding commercial applications due to the difficulty in extracting the catalysts or removing residual oxidants after the degradation process. Several research studies have shown the combined effectiveness of UV-C (254 nm) and NTP in treating wastewater29,30 Edri et al.31 demonstrated that exposing nitrates (NO3−) to UV-C light at wavelengths below 240 nm leads to the formation of photo-sensitized oxidants, including ·HO and reactive nitrogen species (RNS). These reactive species were then applied in AOPs for the treatment of wastewater and groundwater.
Little research has been conducted on the utilization of KrCl* excimer lamps (far UV-C at 222 nm) for UV/AOPs32–34 Xu et al.35 demonstrated that far UV-C irradiation at 222 nm, using KrCl* excilamps, can effectively enhance the photolysis and oxidation of micropollutants. However, the strong absorption of NO3− and nitrites (NO2−) at this wavelength may influence the formation of disinfection by-products. The combination of UV 222 and NTP, which is considerably safer for human exposure, has not yet been the subject of any research. Far UV-C can produce an abundance of ·HO when reacting with nitrates (NO3−) and H2O2 due to higher molar absorption coefficients of NO3− and H2O2 at 222 nm.36 Therefore, the combination of plasma and UV 222 has the potential to increase the formation of reactive species, particularly ·HO, improve the degradation process selectivity, and remove the requirement for the addition of oxidants from the outside. To the best of our knowledge, no research has been published or compared on how well plasma coupled with UV 222 degrades micropollutants in contrast to conventional AOPs.
The goal of this study was to provide a method for OMP degradation which is oxidant free, electrically effective and environmentally friendly. In this study, a DBD plasma reactor and a KrCl* excimer lamp have been developed and engineered together for the study of OMP degradation. The experiments were performed for a comparative study of the degradation of CBZ and SMX under DBD plasma, a KrCl* excilamp (far UV-C; 222 nm), low pressure ultraviolet light [LPUV (UV-C; 254 nm)] lamp, and in the different combinations of these methods. The scope includes, quantifying the concentration of plasma-produced reactive species (such as ·HO, H2O2, NO3−, and NO2−) and utilize them under the irradiation of a KrCl* excilamp for degradation of OMPs. The electrical energy per order for each method has been calculated and compared to obtain the most electrically effective method. A potential mechanism underlying the degradation of CBZ and SMX, along with their respective degradation pathways, has been discussed using HR-MS analysis.
2. Experimental setup
2.1 Selection of micropollutants
Two environmentally significant micropollutants, CBZ and SMX, were selected for this study based on their frequent detection in wastewater treatment plant effluents and possible harmful impacts on the aquatic ecosystem37,38 In addition, CBZ and SMX were selected because of their unique photochemical properties at 222 nm and 254 nm. These pollutants exhibit significant variations in their molar absorption coefficients (ε) at 222 nm and 254 nm, as depicted in Fig. 1. The molar absorption coefficient of CBZ at 222 nm is 22
182 M−1 cm−1, whereas it decreases to 5327 M−1 cm−1 at 254 nm. That means CBZ will degrade four times faster under 222 nm direct photolysis compared to 254 nm. In the case of SMX, the molar absorption coefficient at 222 nm is 8200 M−1 cm−1, and at 254 nm it is found as 14
468 M−1 cm−1. That means SMX will degrade 1.8 times faster at 254 nm compared to 222 nm. So, the differences in absorbance and reactivity towards the KrCl* excilamp (further termed as UV 222 throughout), LPUV lamp (254 nm), ·HO, NO3−, and H2O2 are particularly important for identifying and explaining the potential role in decomposition efficiency among the selected micropollutants. The physical properties, such as chemical structure, chemical formula, molecular mass, and solubility of SMX and CBZ, are presented in Table S1.†
 |
| Fig. 1 Molar absorption spectra of CBZ, SMX, and H2O2 × 10 and UV-vis absorption spectra of plasma treated water (PTW) along with emission spectra of the far UV-C 222 nm excilamp and LPUV source. | |
2.2 Reagents and test waters
Analytical grade SMX and CBZ were purchased from Sigma-Aldrich and used without further purification. All stock solutions for SMX, CBZ, H2O2, and plasma-treated water (PTW) were prepared in lab-grade deionized water (DIW) and tap water (TW). The characteristics of both water matrices are listed in Table S2.† Detailed information about the other chemicals used in this study can be seen in the ESI.†
2.3 Plasma and UV exposure experiments
A double dielectric barrier discharge (DDBD) based plasma device has been developed (further termed as plasma throughout) and used for the degradation of CBZ and SMX in different water matrices. As a source of far UV-C at 222 nm, a DBD-based planar KrCl* excilamp has been developed and used for the degradation process. A 40 W (Philips) conventional LPUV lamp emitting 254 nm has also been used to compare the results. The schematic for the DDBD plasma source and DBD-based KrCl* excilamp is shown in Fig. 2, along with the complete experimental setup used for pollutant degradation. For the development of the DDBD plasma reactor, two cylindrically coaxial quartz tubes with a wall thickness of 1.5 mm are used as the dielectric material. The outer diameter of the smaller quartz tube is 10 mm, and the inner diameter of the larger quartz tube is 13 mm. A gas gap of 1.5 mm is created where plasma formation takes place. The live electrode is made up of an aluminium rod with helical grooves on it, having a diameter of 7 mm. The grounded electrode is made of copper tape with a thickness of 0.04 mm and attached to the outer surface of the larger quartz tube. Pure argon gas (99.999%) for all the experiments was used in this study. The DDBD plasma reactor aligned vertically and the gap between the DDBD plasma reactor and water surface was 1 mm.
 |
| Fig. 2 Experimental setup for the pollutant degradation using plasma and UV 222 equipped with optical and electrical measuring equipment. | |
The planar KrCl* excilamp has dimensions of 50 × 50 × 6 mm3. For the planar DBD geometry, two flat quartz plates with a thickness of 2 mm were pinched together paralleled by keeping a gas gap of 2 mm. The krypton gas with 1% balanced chlorine gas is used as the discharge gas for the 222 nm exciplex formation. More details about the development process of the KrCl* excilamp can be found in our previous paper39,40 and can also be seen from section 1.2 of the ESI.† The experimental setup used for the development of the planar KrCl* excilamp is shown in Fig. S1.† The DDBD plasma source and KrCl* excilamp have been powered with a bipolar pulsed power supply [1–25 kV peak voltage, 5–50 kHz frequency, maximum current up to 1A] having a pulsed width of 2 μs and a typical voltage–current waveform is shown in Fig. S2.† The voltage and current waveforms for the DDBD plasma source have been recorded using a four-channel digital oscilloscope, which was connected to a high voltage probe [Tektronix P6015A, 1000x], and a Pearson current monitor (Model 110, 0.1 V A−1) to measure the corresponding voltage and currents, respectively. The V–I characteristics have been used to calculate the energy consumed and power consumed during plasma discharge using the following equations:41,42
|  | (1) |
| Power Dissipated, P = E·f | (2) |
where
v(
t) is the applied voltage,
i(
t) is the discharge current,
T is the time period, and
f is the frequency (25 kHz for the DDBD plasma reactor and 20 kHz for the KrCl* excilamp). The power dissipated into the electrical discharge is obtained at nearly 15.44 W (at an optimized parameter of 5 kV per 25 kHz) and 17.16 W (at an optimized parameter of 8 kV per 20 kHz) for the DDBD plasma reactor and KrCl* excilamp, respectively.
The optical emission spectrum of the DDBD plasma source and KrCl* excilamp has been recorded using an Andor made spectrometer (SR-500i-B1) integrated with an optical fibre with a core diameter of 1 mm, an opening slit width of 10 μm and 1200 grooves per mm grating. For the absolute irradiation intensity output (μW cm−2) of the excimer source, a Hamamatsu UV power meter (C9556) has been used with a sensor head (H9535), pre-calibrated at 222 nm. The UV power meter shows the real-time data every second, and the data have been recorded for 2 minutes at each voltage value, and the averaged data have been plotted. As shown in Fig. 2, after pre-treatment with the plasma source, the wastewater containing a pool of reactive species was treated with a UV 222 source. The degradation studies were carried out using a single-step process for plasma, LPUV and UV 222 and a two-step process for coupling plasma with LPUV and UV 222.
2.4 Analytical methods
The initial concentration of both micropollutants is 10 mg L−1 and the volume of liquid treated is 100 ml. The UV irradiation tank was a cylindrical type of vessel and has a UV lamp positioned on the upper portion. The radius of the vessel was 5 cm and the height of the vessel was 2 cm. Since the transmission of UV in water is very less, thus the depth of water for the experiments was kept at 1.2 cm with a surface area of 78.5 cm2. Continuous steering of treated wastewater was required for the uniform treatment throughput the experimentation. The plasma vessel has a height of around 10 cm and the diameter was around 5 cm. The DDBD produced reactive oxygen and nitrogen species were exposed to the treated wastewater at a distance of around 1 mm. Again, for uniform treatment, the solution is continuously stirred throughout the experimentation. The used UV fluence of the KrCl* excimer lamp and LPUV lamp is around 1050 μW cm−2, and a detailed explanation is provided in the Results and discussion section. A UV-Vis spectrophotometer (PerkinElmer, USA) was used to measure the absorbance of SMX, CBZ, PTW, and H2O2. The molar absorption coefficients (ε) for SMX, CBZ and H2O2 at 222 nm and 254 nm are determined using the following relationships,43 | 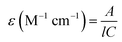 | (3) |
where A is the absorbance of the sample at a particular path length, C is the concentration of the target compound (M), and l is the path length (cm).
Hydroxyl radicals in aqueous suspension were measured using a chemical probe (terephthalic acid: TA) method as reported elsewhere.44 In the same way, 100 mL of distilled water, 2 mM TA, and 5 mM NaOH are mixed and stirred at 200 rpm for 8 hours. The obtained solution was exposed to a plasma source for different time intervals. TA then reacts with ·OH to produce 2-hydroxyterephthalic acid (HTA), and the fluorescence of HTA at 425 nm has been measured using a fluorescence spectrophotometer (LS 55, PerkinElmer, USA) after excitation at 315 nm. NO3− and NO2− were measured using spectrophotometric methods, as discussed elsewhere.45 The pH, conductivity, and TDS of the used water matrices were measured using pre-calibrated probes. High performance liquid chromatography (HPLC) with a UV-Vis detection system (Waters, Model 2489) was used to measure the SMX and CBZ concentration at wavelengths of 260 nm and 286 nm, respectively. The initial and final concentrations over different treatment times are used to calculate the degradation efficiency of the respective pollutant using eqn (4). The SMX and CBZ degradation profiles have been confirmed by HR-MS analysis (Agilent 6500 Q-TOF LC/MS system).
When choosing the optimal approach for wastewater treatment, energy cost is often viewed as the most essential parameter. The efficacy of each method was assessed using electrical energy per order (EEO) values using eqn (5), which represent the amount of electric energy required in kilowatt-hours [kW-h] to treat 1 m3 of polluted water.46 The pseudo-first-order kinetic rate constants (k) for SMX and CBZ degradation have been calculated using eqn (6).
|  | (4) |
| 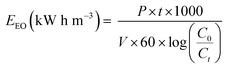 | (5) |
|  | (6) |
where
C0 is the initial concentration of the pollutant (mg L
−1),
Ct is the pollutant concentration after treatment time
t (mg L
−1),
V is the volume of the treated solution (L),
P is the input power (kW),
t is the treatment time (hour), and
k is the first order rate coefficient.
In the next section, the results are categorized into four separate approaches for wastewater treatment.
1. Employing plasma to produce reactive species in DIW and TW along with their characterization and their impact on the degradation of different micropollutants.
2. Employing UV radiation with a wavelength of 222 nm and 254 nm in both DIW and TW and its impact on the degradation of different micropollutants under direct photolysis.
3. Employing plasma pre-treatment followed by LPUV or UV 222 treatment in DIW and TW.
4. Quantification of ·HO generated by coupling both the processes and its impact on the degradation of different micropollutants.
3. Results and discussion
3.1 Optical emission spectroscopy and absolute intensity measurements
3.1.1 Optical emission spectra (OES) of DDBD plasma.
A strong electric field is produced in the space between the dielectric and the electrode due to space charge building over the dielectric surface as the applied voltage increases. N2 emission band peaks dominate the air spectra during DDBD plasma creation because stray electrons accelerate and accumulate enough energy to disintegrate or ionise the molecules or atoms in the air, as illustrated in Fig. 3. To understand the excitation behaviour of different molecules and for the characterization of the plasma, the emission spectra of the DDBD plasma source have been recorded. The air DDBD plasma process will produce excited state atoms, crucial intermediates for producing reactive species in an aqueous solution. Fig. S3† displays the OES of argon plasma in the range of 200–900 nm. In the ultraviolet or visible region, the different excited states of the nitrogen [N2(C–B)] can be visualised from the spectrum. The spectra obtained primarily show significant emission peaks corresponding to the electronic transition of the nitrogen second positive system (C3Πu → B3Πg), shifting from a high-energy vibrational band to a lower-energy one. Additionally, there are weaker emission bands associated with the N2+ first negative system (C3Σ+u → B3Σ+g). The prominent peak at 309 nm illustrates the OH (A–X) generated during plasma formation.47 Different excited argon atomic lines are observable in the visible and infrared regions between 670 and 850 nm. The quantification and effect of different reactive species in the degradation of the SMX and CBZ are studied in further sections.
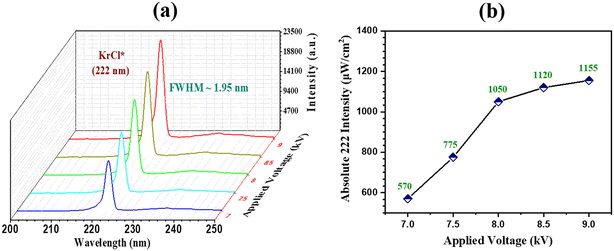 |
| Fig. 3 (a) Emission spectra of the KrCl* 222 nm excilamp at a gas pressure of 150 mbar and different applied voltages; (b) absolute intensity of the KrCl* 222 nm excilamp at different applied parameters. | |
3.1.2 Emission spectra of the KrCl* excilamp and absolute irradiation intensity.
The OES of the developed 222 nm KrCl* excilamp is shown in Fig. 3(a). The figure shows several weak transition bands at 235 nm (B–A transition for KrCl*) and 240 nm (C–A transition for KrCl*), in addition to a strong 222 nm spectral band corresponding to the B–X transition for KrCl*. These weak bands contribute to the breakdown of contaminants by increasing the production of active radicals in addition to the 222 nm spectral band. Because of its narrow and intense band at 222 nm, which is considered highly advantageous, we observed a narrow band with a full-width half maxima (FWHM) of 1.95 nm at reduced gas pressure (150 mbar) with minimal lamp heating. The absolute intensity of the excimer 222 nm has also been measured, and a maximum of 1155 μW cm−2 has been reported. The intensity increases linearly with increasing input voltage up to a certain value (1050 μW cm−2). Still, at higher voltages, it becomes saturated due to the power dissipated in heating rather than in the discharge process, as shown in Fig. 3(b). Therefore, for further experimentation, the KrCl* excilamp has been operated at 8 kV per 20 kHz throughout the experiments, which corresponds to a UV 222 intensity of 1050 μW cm−2.
3.2 Measurement of reactive species in DIW and TW induced by plasma and their roles in the degradation process
3.2.1 Quantification of hydroxyl radicals using a terephthalate probe.
The hydroxyl radical is a strong oxidising reactive species that plays a vital role in the degradation of various micropollutants. According to earlier research, ·HO could also efficiently break down SMX and CBZ in an aqueous solution.48–50 To quantify the concentration of ·HO captured in the different aqueous solutions, fluorescence spectra for various treatment durations have been recorded [see Fig. S4(a) and (b)†] and then compared with the standard fluorescence curve of HTA. As illustrated in Fig. 4(a), the accumulated concentration of ·HO in aqueous solution reaches 20 μM after 3 min of plasma treatment in DIW and TW and increases continuously as the treatment time progresses. In addition to directly producing ·HO by electron dissociation (eqn (7)), water molecules can also be triggered and ionised to produce H2O+, which is then reacted with other molecules to produce H3O+ and ·HO (eqn (8) and (9)).51 | H2O + e− → ·OH + ·H + e− | (7) |
| H2O + H2O+ → H3O+ + ·OH | (9) |
| Ar* + H2O → Ar + H2O+ + e− (12.6 eV) | (11) |
| e− + H2O+ → H + OH (1 eV) | (12) |
The concentration of ·HO increases with increasing plasma exposure time in both types of water matrices, but the rate of production of ·HO is higher in TW (8.24 × 10−8 M s−1) as compared to DIW (7.91 × 10−8 M s−1). This is due to the fact that the presence of dissolved minerals, such as salts and metal ions (like NaCl, MgCl, Na2SO4, etc.), in TW can serve as catalysts for the production of ·HO during plasma treatment.52 The above-mentioned concentration of ·HO was measured in the DIW or TW, while it dropped to almost zero when measured in the presence of SMX or CBZ. This is because SMX and CBZ have a distinct advantage over TA in the competition for ·HO in spiked water. As a result, there is minimal interaction between TA and ·HO, which is why we did not find any HTA when SMX and CBZ were present.
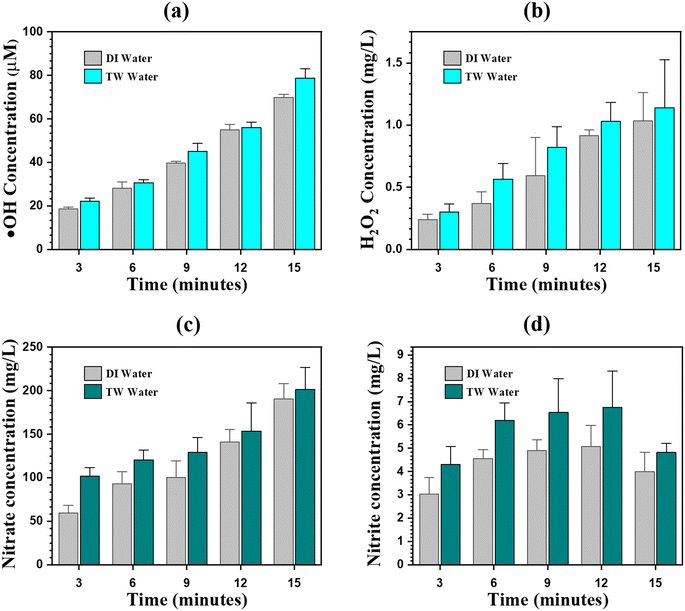 |
| Fig. 4 Reactive species generated by the DDBD plasma reactor. (a) The accumulated amount of ·HO in DIW and TW, (b) concentration of H2O2 in DIW and TW, (c) concentration of NO3− in DIW and TW, and (d) concentration of NO2− in DIW and TW after DDBD plasma treatment over different exposure times. | |
3.2.2 Hydrogen peroxide.
Fig. 4(b) shows that the concentration of H2O2 in the DIW and TW is gradually increased with increasing plasma exposure time and reaches 1.2 mg L−1 after 15 minutes of plasma exposure. Aqueous H2O2 is generated by the combination reaction of the aqueous phase ·HO (eqn (13)),51 as well as the penetration of gaseous H2O2 has the potential to be the source of aqueous H2O2 (eqn (16)). However, no ·HO was detected in the treated solution in the presence of SMX and CBZ. At that point, no H2O2 should be correspondingly present in the aqueous solution, according to eqn (13). The concentration of H2O2 in the SMX and CBZ solution gradually increased with the discharge time and reached ∼1 mg L−1 after 15 minutes of plasma exposure. Therefore, aqueous ·HO is not only a major source of aqueous H2O2; rather, the dissolution of gaseous H2O2 (eqn (16)) may play a regnant role in the production of aqueous H2O2, as supported by Chen et al.53 The main reactions involved in the generation of H2O2 in the gas and aqueous phase are as follows:51,54 | 2 ·OH (aq.) → H2O2 (aq.) | (13) |
| 3H2O + O3 → 3H2O2 (g) | (14) |
| H2O2 (g) → H2O2 (aq.) | (16) |
| H2O2 (aq.) + hv → 2 ·OH | (17) |
In contrast to ·HO, the concentration of H2O2 reduces relatively little (see Table S3†) when SMX and CBZ are present in the aqueous solution, suggesting that the aqueous H2O2 has no discernible impact on the degradation of SMX and CBZ. But the above results demonstrate the crucial role of ·HO in the degradation of SMX and CBZ. The role of H2O2 in aqueous solution generated by the plasma is not significant. However, the photolysis of aqueous H2O2 (via UV 222) can generate ·HO in the aqueous solution. Hu et al.55 performed the degradation of SMX using oxygen plasma and reported that the aqueous phase ·HO is the main cause of the degradation. However, H2O2 in the aqueous phase plays a negligible role in the degradation of SMX. To sum up, the leftover concentration of NO3− and H2O2 can be used for the faster degradation of SMX and CBZ via photolysis of NO3− and H2O2 using 222 nm UV-C light to generate ·HO.
3.2.3 Nitrites and nitrates.
Along with reactive oxygen species (ROS), the DDBD plasma also generates reactive nitrogen species (RNS), especially NO2− and NO3−, in the aqueous medium. The concentration of NO2− and NO3− has been detected using spectrophotometry, and their concentration increased with respect to plasma exposure time. Eqn (18) states that NO is created when N2 in the gaseous medium reacts with primary oxygen species. The electron produced by the plasma process has the ability to dissociate N2 to form atomic nitrogen in addition to atomic oxygen. The detailed reaction mechanism involved in the formation of NOx is also explained by Rayaroth et al.56 The formation of RNS during the plasma process is evidenced by NO2− and NO3− generation and shown in Fig. 4(c) and (d). The concentration of NO2− and NO3− increased with time in both types of water matrices, and SMX and CBZ spiked solutions (see Table S3†). The maximum concentration of NO3− (201 mg L−1) has been recorded after 15 minutes of treatment, whereas the NO2− (5.9 mg L−1) concentration reached a maximum after 9 minutes and started decreasing after further treatment. This is because, after a certain time interval, NO2− starts converting into NO3− after reacting with H2O2 and O3 (eqn (21) and (22)). | N (g) + xO (g) → NOx (g) | (18) |
| 2NO2 (aq.) + H2O (aq.) → NO2− (aq.) + NO3− (aq.) + 2H+ (aq.) | (19) |
| NO (aq.) + NO2 (aq.) + H2O (aq.) → 2NO2− (aq.) + 2H+ ( aq.) | (20) |
| NO2− (aq.) + H2O2 (aq.) → NO3− (aq.) + H2O (aq.) | (21) |
| NO2− (aq.) + O3 ( aq.) → NO3− (aq.) + O2 (aq.) | (22) |
Rayaroth et al.56 performed the degradation of CBZ in ultrapure water. They reported that the concentration of NO2− decreased when the solution was spiked with CBZ and treated with the plasma, whereas the concentration of NO3− increased in the presence of CBZ. A similar pattern is observed in this study, and we found that the concentration of NO2− is almost identical when SMX and CBZ are spiked in DIW and TW. However, the concentration of NO3− increases up to twofold, indicating that the nitrogen in SMX/CBZ and their intermediate products are oxidized to release NO2 ions. On the other hand, NO3− is not involved in the product formation or in the degradation process. Therefore, this residual concentration of NO3− can be utilized to produce ·HO under photolysis (λ < 240 nm) viaeqn (23) and (24), which can improve the overall efficiency of the process. | NO3− + hv (222 nm) → ·NO2 + ·O− | (23) |
| ·O− + H2O → ·OH + OH− | (24) |
It is to be emphasized that the photolysis of H2O2 and NO3− takes place in the presence of UV 222 radiation, which is why the wavelength 222 nm was chosen. Due to the prolonged degradation time of CBZ and SMX when exposed to plasma, we have employed DBD plasma to generate a pool of reactive species, specifically H2O2 and NO3−. These reactive species are then used as a radical promoter under UV 222 radiation.
During plasma exposure, reactive ions such as H+, NO2−, and NO3− are primarily produced. As the plasma treatment duration increases, the concentration of these active ions in the water rises, leading to a continuous drop in the pH of the treated water. In our measurements, we observed a notable reduction in pH, decreasing from 6.91 to 2.74 for DIW and from 5.63 to 2.27 for TW with extended plasma exposure.
3.3 Hydroxyl radicals from PTW and UV 222 radiation
A previously described planar 222 nm KrCl* excilamp is utilized for the photolysis of H2O2 and NO3− in an aqueous solution. Because of higher molar absorption coefficients of H2O2 at 222 nm (98.7 M−1 cm−1) compared to 254 nm (18.8 M−1 cm−1), the rate of production of ·HO is found to be higher in the case of UV 222 radiation [see Fig. 1]. Enhanced generation of ·HO from the plasma produced H2O2 (∼1–1.5 mg L−1) is observed in UV 222 compared to LPUV in both water matrices, i.e., DIW and TW. This is one of the benefits of employing a DDBD plasma reactor in conjunction with UV 222 radiation. On the other hand, the presence of NO3− in the PTW provides an additional advantage because in a recent study by Wu et al.,57 it was reported that NO3− can be used as a source of ·HO and RNS in photochemical AOPs, adding to the radicals generated by the UV-AOPs for OMP oxidation. When a similar concentration of H2O2 and NO3− was added to the DIW and TW separately, it was found that the rate of production of ·HO in the presence of H2O2 and NO3− (plasma produced) was significantly higher. As can be seen from Fig. 5, the concentration and rate of production of ·HO measured in DIW for plasma + UV 222 (46.7 × 10−8 M s−1) is higher as compared to plasma + LPUV (32.2 × 10−8 M s−1) and plasma alone (8.24 × 10−8 M s−1). The main reason for the lower production of ·HO in the case of LPUV is that NO3− is not absorbed appreciably at 254 nm. However, NO2− and NO3− are absorbed very strongly at shorter wavelengths, i.e., below 240 nm.32,58
 |
| Fig. 5 ·HO concentration and their rate of production under plasma, plasma + LPUV, and plasma + UV 222. | |
3.4 Role of RONS on the degradation of CBZ and SMX
It can be visualized from Fig. 1 that the water treated using plasma absorbs more photons of 222 nm light than 254 nm light. Payne et al.32 conducted a comparative analysis of ·HO generation in various AOPs such as 222 nm/NO3−, 222 nm/H2O2 and 254 nm/H2O2. They observed that the 222 nm/NO3− system generated the highest amount of ·HO in the water matrix, surpassing the 222 nm/H2O2 and 254 nm/H2O2 systems. The present study investigates the degradation of SMX and CBZ using five distinct treatment methods i.e., plasma, LPUV, UV 222, plasma + LPUV, and plasma + UV 222. To gain insight into the practicability, the degradation process is conducted by utilizing two different water matrices, namely DIW and TW. Firstly, the aqueous solution of SMX and CBZ in DIW is treated using plasma, and only ∼11.2% degradation of CBZ takes place after 15 minutes of plasma treatment. In contrast, the degradation of CBZ in TW (real water matrix) decreases further to ∼6.5% due to the presence of different ions in the TW and their competition with the plasma-generated reactive species. But in the case of SMX, 15 minutes of plasma treatment shows ∼30% degradation in DIW, which decreases to ∼23% when the treatment is performed in TW, as shown in Fig. 6. Rayaroth et al.56 also reported comparable findings, as they observed a degradation rate of more than 90% for CBZ in pure water, whereas the degradation rate decreased to 83% when subjected to TW during a treatment time of 40 minutes.
 |
| Fig. 6 Degradation profiles of CBZ in (a) DIW; (b) TW and degradation profiles of SMX in (c) DIW; (d) TW in the case of plasma, LPUV, UV 222, plasma + LPUV, and plasma + UV 222 (C0 = 10 mg L−1, UV intensity = 1050 μW cm−2 and pH 6–7). | |
In the case of only LPUV treatment for 15 minutes, the degradation performance for CBZ was much poorer and only 4.75% degraded in TW, whereas in DIW, it decreased further to ∼3.4%. The main reason behind this degradation behaviour would be the lowest molar absorption coefficient of CBZ at 254 nm (see Fig. 1). In contrast, due to the higher molar absorption coefficients of SMX at 254 nm, LPUV shows exceptional performance in the degradation of SMX, and ∼57% of SMX degradation takes place in the case of DIW in just 15 minutes of treatment (see Fig. 6). Further, the degradation of SMX accelerates in the TW under LPUV due to the presence of NO3− and other reactive species and reaches up to ∼63.7%. In contrast, CBZ shows a higher molar absorption coefficient at 222 nm; therefore; it degrades faster under direct photolysis of UV 222 (∼34.7%) in DIW and also due to higher absorption of NO3− at 222 nm, the degradation of CBZ becomes higher (∼42.7%) in the case of the TW matrix. Liu et al.43 also found that CBZ degraded faster in the secondary effluent than in ultrapure water. In the case of SMX, the 222 nm light is less effective as compared to LPUV and degraded only ∼25% pollutant in 15 minutes of the treatment, i.e., the degradation is approximately two-fold slower in DIW under 222 nm as compared to LPUV. However, due to the tendency of 222 nm to form more ·HO in the presence of NO3−, the degradation in TW is higher and reaches ∼60%, approximately equal to the LPUV treatment in TW. The LPUV or KrCl* excilamp (222 nm) has been integrated with the plasma to improve the degradation efficiency and shorten the treatment time. The degradation of CBZ and SMX by the combination of plasma and UV 222 is remarkable; in the case of DIW, the full degradation of CBZ and SMX required just 15 minutes and 10 minutes, respectively. Additionally, the rate of degradation accelerated in the case of TW, and it only took 12 minutes and 8 minutes for CBZ and SMX to completely degrade, which is perhaps due to the presence of more NO3− and reactive species (see Fig. 6).
UV/H2O2 based AOPs are currently being used for the treatment of wastewater. Still, the problem lies in adding H2O2 externally and then the extraction/removal of residual H2O2 after wastewater treatment, which is the biggest problem59,60 This study addressed the first problem by integrating the plasma with UV 222. The H2O2 produced by the plasma in this hybrid system is within the bounds established by global regulatory bodies.61 Thus, the external addition of H2O2 to the reactor is eliminated by this method. Furthermore, because H2O2 has a low molar absorption coefficient at 254 nm, LPUV can only photolyze a small fraction of H2O2 into ·HO. We have utilized a KrCl* excilamp (UV 222 nm) to target this issue because H2O2 has a high molar absorption coefficient at 222 nm, which has allowed to convert H2O2 into ·HO. Thus, the use of a 222 nm source also solves the second issue of residual H2O2. This combination also offers an added benefit, as long-lived plasma species like NO3− have a greater ability to absorb 222 nm photons compared to 254 nm photons. This enhances the feasibility and additive-free nature of the aforementioned process, which can also be referred to as a green AOP. Fig. S5† displays the decomposition rate at which CBZ and SMX degrade when subjected to various treatment processes for a duration of 15 minutes.
Fig. 7(a) shows that the k values of SMX and CBZ have supported their respective degradation trends. All treatment methods follow a pseudo-first-order kinetics model, with the plasma + UV 222 method exhibiting the greatest k value (0.341). When utilizing plasma + UV 222 in DIW, the rate of CBZ degradation is 30 times faster than using plasma alone. Moreover, it is remarkably 114 times faster than using LPUV alone and 10 times faster when utilizing UV 222 alone. In the case of SMX in DIW, the degradation rate is 15 times faster under plasma + UV 222 than using only plasma. Comparing the degradation to LPUV, however, it is just 6 times quicker. It is clear that the rate of SMX degradation is 1.3 times higher than the rate of CBZ degradation in the DIW by comparing the k values of the pollutants in the plasma + UV 222. On the other hand, both micropollutants exhibit the same degrading behaviours in the TW.
 |
| Fig. 7 (a) Respective k values for the different methods in the DIW and TW for the degradation of SMX and CBZ (C0 = 10 mg L−1, UV intensity = 1050 μW cm−2 and pH 6–7); (b) electrical energy per order (EEO) required in the process of degrading SMX and CBZ by various treatment processes. | |
The degradation process is significantly aided by the use of plasma with UV 222 radiation. This is primarily because nearly all the plasma-produced species have a higher absorption of 222 nm light, as illustrated in Fig. 1. The generation of NO3− and H2O2 by plasma is of significant importance in the breakdown process of both micropollutants. Table S4† demonstrates that the complete degradation time varies significantly across different methods, owing to the distinct properties of the micropollutants. But the complete degradation time for the plasma + UV 222 exhibits a substantial disparity compared to other treatment methods for both pollutants. The approach employed in this study has the advantage of keeping the plasma/far UV-C devices out of contact with the wastewater, which prevent it from soiling unwanted microorganisms and prolongs their lifespan. This procedure is also environmentally friendly because no additional external chemicals are being used as a catalyst or radical promoter. Further research is required to test the system's effectiveness with other pollutants as well as on the mixture of the contaminants. Additionally, in order to determine whether the suggested technology can be applied in industrial wastewater treatment plants, testing on actual industrial wastewater is required.
3.5 Electrical energy per order (EEO) comparison and degradation mechanism
Energy cost is often viewed as the most important parameter, along with degradation rate, when choosing the most efficient wastewater treatment method. The EEO concept has been applied to assess the energy needs for eliminating each OMP through different procedures. The initial concentration of pollutants and the power consumed in each treatment method have been used to compute the EEO values (eqn (5)) for SMX and CBZ degradation. Fig. 7(b) shows the electrical energy required in the process of degrading SMX and CBZ by various treatment processes. The most electrically efficient approach for degrading SMX in DIW is LPUV, but in TW, it is UV 222 due to the increased production of ·HO in the system. Plasma + UV 222 is the most electrically efficient approach for degrading CBZ in DIW, while UV 222 is the most energy-efficient approach in TW. Since the plasma process requires more power than some other processes, the EEO of the plasma + UV 222 process is slightly higher than that of only UV 222. But, when considering the degradation of various micropollutants, the plasma + UV 222 approach is the most stable and suitable due to its distinctive ability to produce higher quantities of ·HO and comparable treatment time for distinct OMPs.
In general, plasma systems consume a significant amount of power. However, if it is possible to generate a large quantity of RONS in the treated water using plasma, even at a low power threshold, then the system could become more energy efficient. Therefore, more investigation is needed to create an NTP source that is more energy-efficient. This research underscores the potential for making plasma systems more energy-efficient, offering hope for sustainable water treatment solutions.
3.6 Degradation pathway of SMX and CBZ
The untreated and treated SMX and CBZ solutions have been investigated using HRMS analysis to determine the intermediate products and degradation pathway. Initially, the SMX molecules in the aqueous solution were directly attached by ·HO so that the C–H–C bond was split and then C3H2 was separated from the SMX molecule. After the destruction of the C–H–C bond, the SMX molecules were converted into 4-amino-N-carbamolybenzene-sulfonamide (m/z = 233). The degradation of this intermediate by ·HO resulted in the generation of sulphanilamide (m/z = 97). The intermediate groups are consequently attacked by ·HO, being converted to 2,4-dinitrobenzene-sulfinic acid (m/z = 217), then phenylsulfinic acid (m/z = 165), and finally into malic acid (m/z = 81) and oxalic acid (m/z = 39). Therefore, oxalic acid has high potential to become CO2 and H2O, and it can easily be converted into these compounds as an end-product. It should be noted that only malic acid was identified in the analysis of HRMS. Fig. 8(a) shows a probable degradation pathway for SMX in the plasma/UV 222 process based on the previous studies and molecular fragments identified in the HRMS analysis.62,63
 |
| Fig. 8 Proposed degradation pathway of (a) SMX and (b) CBZ in the plasma/UV 222 process. | |
In the case of CBZ, the initial steps of degradation involve the attack of ·HO on the CBZ molecule, leading to the formation of hydrolyzed CBZ (m/z = 253). Then, this intermediate was converted into further intermediates as 2,3-benzoquinoline acrydine (m/z = 180), 2-aminoanthraquinone (m/z = 224), and 9,10-dihydroacridine (m/z = 196). Finally, all mentioned intermediates will be attacked by ·HO and then mineralized into CO2 and H2O molecules. Fig. 8(b) shows a probable degradation pathway for CBZ in the plasma/UV 222 process based on the previous studies and molecular fragments identified in the HRMS analysis.56,64
The plasma + UV 222 approach-based degradation studies of SMX and CBZ in DIW and TW are also confirmed by scavenging studies, as seen in Fig. 9(a). These studies examine the role of the reactive species, especially ·OH, in the plasma + UV 222-based degradation mechanism. This study uses isopropanol (IPA) as a scavenger for ·OH. The experiments are carried out using the plasma + UV 222 approach with and without a scavenger. It is found that adding IPA considerably reduces the rate of SMX and CBZ degradation. Without adding any scavengers, the degradation rate of SMX for a treatment time of 9 minutes is found to be 98.58% and 99.99% in DIW and TW, respectively. However, with IPA as a scavenger, SMX exhibits only 11.51% and 29.51% degradation in DIW and TW, respectively. A similar trend has been obtained in the case of CBZ, which degrades 69.52% and 84.52% in DIW and TW, respectively, without a scavenger. In contrast, it degrades only 17.19% and 22.29% in DIW and TW, respectively, when a scavenger is introduced. Therefore, this experiment shows that ·OH is highly involved in the degradation mechanism of SMX and CBZ. Fig. 9(b) depicts the suggested reaction process for OMP degradation under the plasma + UV 222. We have investigated several ways to generate ·HO, such as the breakdown of plasma-produced NO3− and H2O2 when exposed to excimer 222 and the interaction of ozone and/or KrCl* excilamp 222 nm light with the water molecules. This research underscores the need for further investigation into the potential of plasma coupling with UV 222 nm to develop a more efficient wastewater treatment process. We can aid in the development of more effective and long-lasting water treatment technologies by investigating and improving these approaches further.
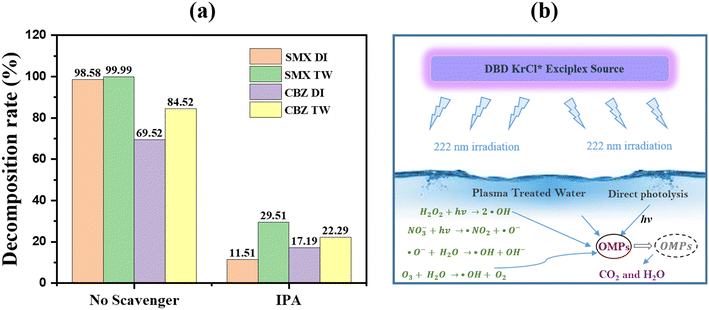 |
| Fig. 9 (a) Quenching studies of hydroxyl radicals during plasma + UV 222 approach based degradation of CBZ and SMX in DIW and TW, and (b) proposed plasma–UV222 mediated degradation mechanism of OMPs. | |
Conclusion
This work shows the development and use of a DBD-based KrCl* excilamp and a DDBD plasma reactor to degrade SMX and CBZ in an aqueous solution. The UV/H2O2 based AOPs are generally recommended for OMP degradation; however, these processes necessitate the addition of H2O2 to the water matrix. In this study, the plasma-produced long-lived species (NO3− and H2O2) are utilized under the 222 nm KrCl* excilamp for the enhanced generation of ·HO, and consequently, faster degradation of the OMPs. This hybrid technique eliminates the burden of removal of residual H2O2 and hence can be termed as a greener technique. The degradation of CBZ under DDBD plasma alone requires 210 minutes for 99.9% degradation, whereas UV 222 alone requires 40 minutes of treatment for the same level of degradation. Interestingly by coupling plasma with UV 222, for the 99.9% degradation of CBZ requires only 12 minutes of treatment.
In comparison to previous methods, the required EEO for the OMP deterioration from this hybrid strategy is quite low (73.38 kW h m−3). When comparing the CBZ and SMX deterioration over time using this hybrid technique, we find that SMX requires 8 minutes of treatment to reach full degradation while CBZ requires 12 minutes. It's interesting to see that all other approaches take significantly longer to degrade two selected OMPs with differing physical properties. But this hybrid strategy is quite successful because it takes almost the same amount of time for both OMPs to degrade. Using HRMS, the intermediate and by-products are also identified, and a degradation pathway has been suggested. More investigations are needed before it can be used to treat actual wastewater that contains a combination of OMPs.
Data availability
Data will be made available on request.
Conflicts of interest
The authors declare that they have no known competing financial interests or personal relationships that could have appeared to influence the work reported in this paper.
Acknowledgements
KA and RJ would like to acknowledge the University Grants Commission (UGC) for providing senior research fellowship during the PhD program. The authors acknowledge the Core Research Grant (CRG), Science and Engineering Research Board (SERB), Department of Science and Technology (DST), Government of India, through project # CRG/2020/005369, for financial assistance with this work.
References
- S. R. Hughes, P. Kay and L. E. Brown, Global synthesis and critical evaluation of pharmaceutical data sets collected from river systems, Environ. Sci. Technol., 2013, 47, 661–677 CrossRef CAS PubMed.
- M. Gavrilescu, K. Demnerová, J. Aamand, S. Agathos and F. Fava, Emerging pollutants in the environment: Present and future challenges in biomonitoring, ecological risks and bioremediation, New Biotechnol., 2015, 32, 147–156 CrossRef CAS PubMed.
- Y. Luo, W. Guo, H. H. Ngo, L. D. Nghiem, F. I. Hai, J. Zhang, S. Liang and X. C. Wang, A review on the occurrence of micropollutants in the aquatic environment and their fate and removal during wastewater treatment, Sci. Total Environ., 2014, 473–474, 619–641 CrossRef CAS PubMed.
- C. Burzio, J. Ekholm, O. Modin, P. Falås, O. Svahn, F. Persson, T. van Erp, D. J. I. Gustavsson and B. M. Wilén, Removal of organic micropollutants from municipal wastewater by aerobic granular sludge and conventional activated sludge, J. Hazard. Mater., 2022, 438, 129528 CrossRef CAS PubMed.
- A. Kumar, N. Škoro, W. Gernjak and N. Puač, Cold atmospheric plasma technology for removal of organic micropollutants from wastewater—a review, Eur. Phys. J. D, 2021, 75, 283 CrossRef CAS.
- Z. A. Yacouba, J. Mendret, G. Lesage, F. Zaviska and S. Brosillon, Removal of organic micropollutants from domestic wastewater: The effect of ozone-based advanced oxidation process on nanofiltration, J. Water Process Eng., 2021, 39, 101869 CrossRef.
- M. Ahmed, M. O. Mavukkandy, A. Giwa, M. Elektorowicz, E. Katsou, O. Khelifi, V. Naddeo and S. W. Hasan, Recent developments in hazardous pollutants removal from wastewater and water reuse within a circular economy, npj Clean Water, 2022, 5, 1–25 CrossRef.
- J. Xu and C. H. Huang, Enhanced Direct Photolysis of Organic Micropollutants by Far-UVC Light at 222 nm from KrCl* Excilamps, Environ. Sci. Technol. Lett., 2023, 10, 543–548 CrossRef CAS PubMed.
- T. Li, Y. Zhang, J. Gan, X. Yu and L. Wang, Superiority of UV222 radiation by in situ aquatic electrode KrCl excimer in disinfecting waterborne pathogens: Mechanism and efficacy, J. Hazard. Mater., 2023, 452, 131292 CrossRef CAS PubMed.
- I. Tsenter, N. Garkusheva, G. Matafonova and V. Batoev, A novel water disinfection method based on dual-wavelength UV radiation of KrCl (222 nm) and XeBr (282 nm) excilamps, J. Environ. Chem. Eng., 2022, 10, 107537 CrossRef CAS.
- R. Yin, X. Ruan, J. Peng, J. Zhao, Y. Zhang, A. Heuzard and C. Shang, Control of Micropollutants in Water by Far-UVC Photolysis of Peracetic Acid, Environ. Sci. Technol. Lett., 2024, 11, 759–763 CrossRef CAS.
- X. Xin, J. Kim, D. C. Ashley and C. H. Huang, Degradation and Defluorination of Per- and Polyfluoroalkyl Substances by Direct Photolysis at 222 nm, ACS ES&T Water, 2023, 3, 2776–2785 Search PubMed.
- R. Yin, Y. Zhang, Y. Wang, J. Zhao and C. Shang, Far-UVC Photolysis of Peroxydisulfate for Micropollutant Degradation in Water, Environ. Sci. Technol., 2024, 58, 6030–6038 CrossRef CAS PubMed.
- K. Ahlawat, R. Jangra, V. Gadodia and R. Prakash, Efficacy of 222 nm radiation on degradation of organic micropollutants in water, Radiat. Eff. Defects Solids, 2024, 179, 1032–1039 CrossRef CAS.
- N. Wardenier, Z. Liu, A. Nikiforov, S. W. H. Van Hulle and C. Leys, Micropollutant elimination by O3, UV and plasma-based AOPs: An evaluation of treatment and energy costs, Chemosphere, 2019, 234, 715–724 CrossRef CAS PubMed.
- K. Ahlawat, R. Jangra and R. Prakash, Environmentally Friendly UV-C Excimer Light Source with Advanced Oxidation Process for Rapid Mineralization of Azo Dye in Wastewater, ACS Omega, 2024, 9, 15615–15632 CrossRef CAS PubMed.
- M. Zhao, T. Li, Y. Zhang, J. Gan, Y. Zhao, X. Yu and L. Wang, Kinetic modeling and mechanisms of parahalogenated phenols degradation by UV222/H2O2, J. Cleaner Prod., 2024, 434, 140255 CrossRef CAS.
- J. Zhao, W. Gong, R. Yin, C. Shang and W. A. Mitch, Control of Aromatic Disinfection Byproducts in Potable Reuse Water by the UV222/H2O2 vs UV254/H2O2 Advanced Oxidation Processes, Environ. Sci. Technol., 2024, 58, 15846–15854 CrossRef CAS PubMed.
- D. B. Miklos, C. Remy, M. Jekel, K. G. Linden, J. E. Drewes and U. Hübner, Evaluation of advanced oxidation processes for water and wastewater treatment – A critical review, Water Res., 2018, 139, 118–131 CrossRef CAS PubMed.
- S. A. A. Balkhi, S. M. Allabakshi, P. S. N. S. R. Srikar, S. Gomosta, R. K. Gangwar and S. M. Maliyekkal, Unwinding the correlation between atmospheric pressure plasma jet operating parameters and variation in antibiotic wastewater characteristics, J. Water Process Eng., 2024, 60, 105186 CrossRef.
- M. Ansari, G. Moussavi, M. H. Ehrampoosh and S. Giannakis, A systematic review of non-thermal plasma (NTP) technologies for synthetic organic pollutants (SOPs) removal from water: Recent advances in energy yield aspects as their key limiting factor, J. Water Process Eng., 2023, 51, 103371 CrossRef.
- R. Jangra, K. Ahlawat and R. Prakash, An SDBD plasma-based source for efficient degradation of VOCs in an enclosed environment, Phys. Lett. A, 2023, 490, 129184 CrossRef CAS.
- V. Rathore, A. Pandey, S. Patel, H. Dave and S. K. Nema, Degradation of dyes using reactive species of atmospheric pressure dielectric barrier discharge formed by a pencil plasma jet, Phys. Scr., 2024, 99, 035602 CrossRef.
- S. Jangra, A. Mishra, R. Mishra, S. Pandey and R. Prakash, Transformative impact of atmospheric cold plasma on mung bean seeds: Unveiling surface characteristics, physicochemical alterations, and enhanced germination potential, AIP Adv., 2024, 14, 075215 CrossRef CAS.
- H. Guo, Y. Su, X. Yang, Y. Wang, Z. Li, Y. Wu and J. Ren, Dielectric Barrier Discharge Plasma Coupled with Catalysis for Organic Wastewater Treatment: A Review, Catalysts, 2023, 13, 10 CrossRef CAS.
- P. Attri, K. Koga, T. Okumura, F. L. Chawarambwa, T. E. Putri, Y. Tsukada, K. Kamataki, N. Itagaki and M. Shiratani, Treatment of organic wastewater by a combination of non-thermal plasma and catalyst: a review, Rev. Mod. Plasma Phys., 2022, 6, 1–27 CrossRef.
- J. Ren, H. Song, H. Guo, Z. Yao, Q. Wei, K. Jiao, Z. Li, C. Zhong, J. Wang and Y. Zhen, Removal of chloramphenicol in water by an improved water falling film dielectric barrier discharge reactor: Performance, mechanism, degradation pathway and toxicity evaluation, J. Cleaner Prod., 2021, 325, 129332 CrossRef CAS.
- Z. Cao, L. Zhao, Y. Sun and J. Feng, Simultaneous removal of enrofloxacin and Cr(VI) using uniaxial wet wall dielectric barrier discharge plasma, J. Cleaner Prod., 2022, 379, 134800 CrossRef CAS.
- S. M. Allabakshi, P. S. N. S. R. Srikar, S. Gomosta, R. K. Gangwar and S. M. Maliyekkal, UV-C photon integrated surface dielectric barrier discharge hybrid reactor: A novel and energy-efficient route for rapid mineralisation of aqueous azo dyes, J. Hazard. Mater., 2023, 446, 130639 CrossRef CAS PubMed.
- J. Lou, J. An, X. Wang, X. Yang, G. Lu, L. Wang and Z. Zhao, Enhanced degradation of oxytetracycline in aqueous solution by DBD plasma-coupled vacuum ultraviolet/ultraviolet (VUV/UVC) system, Chemosphere, 2023, 335, 139021 CrossRef CAS PubMed.
- L. Edri, K. G. Linden, N. Ibrahim, D. Avisar, A. Kaplan, S. Hayoune and Y. Lester, Prediction of organic groundwater contaminant degradation during medium pressure UV/NO3− treatment, Environ. Sci.: Water Res. Technol., 2023, 9, 2275–2282 RSC.
- E. M. Payne, B. Liu, L. Mullen and K. G. Linden, UV 222 nm Emission from KrCl* Excimer Lamps Greatly Improves Advanced Oxidation Performance in Water Treatment, Environ. Sci. Technol. Lett., 2022, 9, 779–785 CrossRef CAS.
- R. Yin, C. E. Anderson, J. Zhao, A. B. Boehm and W. A. Mitch, Controlling contaminants using a far-UVC-based advanced oxidation process for potable reuse, Nat. Water, 2023, 1, 555–562 CrossRef.
- K. Ahlawat, R. Jangra and R. Prakash, Accelerated mineralization of textile wastewater under 222 nm irradiation from Kr/Cl2 excilamp : an environmentally friendly and energy efficient approach, Sci. Rep., 2024, 14, 1–18 CrossRef PubMed.
- J. Xu, R. J. Kann, D. Mohammed and C. H. Huang, Far-UVC 222 nm Treatment: Effects of Nitrate/Nitrite on Disinfection Byproduct Formation Potential, Environ. Sci. Technol., 2024, 58, 15311–15320 CAS.
- X. Ao, X. Zhang, W. Sun, K. G. Linden, E. M. Payne, T. Mao and Z. Li, What is the role of nitrate/nitrite in trace organic contaminants degradation and transformation during UV-based advanced oxidation processes?, Water Res., 2024, 253, 121259 CrossRef CAS PubMed.
- S. Feijoo, M. Kamali and R. Dewil, A review of wastewater treatment technologies for the degradation of pharmaceutically active compounds: Carbamazepine as a case study, Chem. Eng. J., 2023, 455, 140589 CrossRef CAS.
- P. S. Peixoto, P. H. Carvalho, A. Machado, L. Barreiros, A. A. Bordalo, H. P. Oliveira and M. A. Segundo, Development of a Screening Method for Sulfamethoxazole in Environmental Water by Digital Colorimetry Using a Mobile Device, Chemosensors, 2022, 10, 25 CrossRef CAS.
- K. Ahlawat, R. Jangra, A. Ish, N. Jain and R. Prakash, A dielectric barrier discharge based low pressure narrow band far UV-C 222 nm excimer lamp and its efficiency analysis, Phys. Scr., 2024, 99, 025018 CrossRef.
- K. Ahlawat, V. Gadodia, P. Yadav, R. Jangra and R. Prakash, Surface modification of fibres using bipolar pulsed driven DBD plasma based KrCl* and XeI* exciplex sources, Fundamental Plasma Physics, 2024, 12, 100068 CrossRef.
- R. Jangra, K. Ahlawat, A. Dixit and R. Prakash, Efficient deactivation of aerosolized pathogens using a dielectric barrier discharge based cold - plasma detergent in environment device for good indoor air quality, Sci. Rep., 2023, 13, 1–14 CrossRef PubMed.
- R. Jangra, K. Ahlawat, M. K. Mohan and R. Prakash, Indoor air disinfection by non thermal atmospheric pressure plasma: a comparative study of performance in low and high humidity environments, Phys. Scr., 2024, 99, 085612 CrossRef.
- B. Liu, L. Mullen, E. M. Payne and K. G. Linden, Accelerated Ultraviolet Treatment of Carbamazepine and NDMA in Water under 222 nm Irradiation, Environ. Sci. Technol., 2023, 57, 18909–18917 CrossRef CAS PubMed.
- K. Ahlawat, R. Jangra, A. Ish, A. Dixit, D. Fulwani, N. Jain and R. Prakash, Analysis of a UV photocatalytic oxidation-based disinfection system for hydroxyl radicals, negative air ions generation and their impact on inactivation of pathogenic micro-organisms, Rev. Sci. Instrum., 2023, 94, 104103 CrossRef CAS PubMed.
- V. Rathore and S. K. Nema, Optimization of process parameters to generate plasma activated water and study of physicochemical properties of plasma activated solutions at optimum condition, J. Appl. Phys., 2021, 129, 084901 CrossRef CAS.
- J. R. Bolton, K. G. Bircher, W. Tumas and C. A. Tolman, Figures-of-merit for the technical development and application of advanced oxidation technologies for both electric- and solar-driven systems, Pure Appl. Chem., 2001, 73, 627–637 CrossRef CAS.
- C. O. Laux, T. G. Spence, C. H. Kruger and R. N. Zare, Optical diagnostics of atmospheric pressure air plasmas, Plasma Sources Sci. Technol., 2003, 12, 125–138 CrossRef CAS.
- Amina, X. Si, K. Wu, Y. Si and B. Yousaf, Synergistic effects and mechanisms of hydroxyl radical-mediated oxidative degradation of sulfamethoxazole by Fe(II)-EDTA catalyzed calcium peroxide: Implications for remediation of antibiotic-contaminated water, Chem. Eng. J., 2018, 353, 80–91 CrossRef CAS.
- R. Xiao, J. Ma, Z. Luo, W. Zeng, Z. Wei, R. Spinney, W. P. Hu and D. D. Dionysiou, Experimental and theoretical insight into hydroxyl and sulfate radicals-mediated degradation of carbamazepine, Environ. Pollut., 2020, 257, 113498 CrossRef CAS PubMed.
- X. Zhang, M. Kamali, X. Yu, M. E. V. Costa, L. Appels, D. Cabooter and R. Dewil, Kinetics and mechanisms of the carbamazepine degradation in aqueous media using novel iodate-assisted photochemical and photocatalytic systems, Sci. Total Environ., 2022, 825, 153871 CrossRef CAS PubMed.
- Z. Zhang, Z. Xu, C. Cheng, J. Wei, Y. Lan, G. Ni, Q. Sun, S. Qian, H. Zhang, W. Xia, J. Shen, Y. Meng and P. K. Chu, Bactericidal Effects of Plasma Induced Reactive Species in Dielectric Barrier Gas–Liquid Discharge, Plasma Chem. Plasma Process., 2017, 37, 415–431 CrossRef CAS.
- C. Canal, F. Tampieri and M. P. Ginebra, Quantification of plasma-produced hydroxyl radicals in solution and their dependence on the pH, Anal. Chem., 2021, 93, 3666–3670 CrossRef PubMed.
- Z. Chen, D. Liu, H. Xu, W. Xia, Z. Liu, D. Xu, M. Rong and M. G. Kong, Decoupling analysis of the production mechanism of aqueous reactive species induced by a helium plasma jet, Plasma Sources Sci. Technol., 2019, 28, 025001 CrossRef CAS.
- C. A. J. Van Gils, S. Hofmann, B. K. H. L. Boekema, R. Brandenburg and P. J. Bruggeman, Mechanisms of bacterial inactivation in the liquid phase induced by a remote RF cold atmospheric pressure plasma jet, J. Phys. D: Appl. Phys., 2013, 46, 175203 CrossRef.
- S. Hu, W. Yan, J. Yu, B. Zhu, Y. Lan, W. Xi, Z. Xu, W. Han and C. Cheng, Degradation of sulfamethoxazole in water by dielectric barrier discharge plasma jet: influencing parameters, degradation pathway, toxicity evaluation, Plasma Sci. Technol., 2023, 25, 035510 CrossRef.
- M. P. Rayaroth, O. Aubry, H. Rabat, E. Marilleau, Y. Gru, D. Hong and P. Brault, Degradation and transformation of carbamazepine in aqueous medium under non-thermal plasma oxidation process, Chemosphere, 2024, 352, 141449 CrossRef PubMed.
- Y. Wu, L. Bu, X. Duan, S. Zhu, M. Kong, N. Zhu and S. Zhou, Mini review on the roles of nitrate/nitrite in advanced oxidation processes: Radicals transformation and products formation, J. Cleaner Prod., 2020, 273, 123065 CrossRef CAS.
- S. L. Vinge, S. W. Shaheen, C. M. Sharpless and K. G. Linden, Nitrate with benefits: Optimizing radical production during UV water treatment, Environ. Sci.: Water Res. Technol., 2020, 6, 1163–1175 RSC.
- Y. Deng and R. Zhao, Advanced Oxidation Processes (AOPs) in Wastewater Treatment, Curr. Pollut. Rep., 2015, 1, 167–176 CrossRef CAS.
- T. H. Bokhari, N. Ahmad, M. I. Jilani and M. Saeed, UV/H2O2, UV/H2O2/SnO2 and Fe/H2O2 based advanced oxidation processes for the degradation of disperse violet 63 in aqueous medium, Mater. Res. Express, 2020, 7, 015531 CrossRef CAS.
- D. Li, B. Stanford, E. Dickenson, W. O. Khunjar, C. L. Homme, E. J. Rosenfeldt and J. O. Sharp, Effect of advanced oxidation on N-nitrosodimethylamine (NDMA) formation and microbial ecology during pilot-scale biological activated carbon filtration, Water Res., 2017, 113, 160–170 CrossRef CAS PubMed.
- A. R. Yazdanbakhsh, A. Eslami, M. Massoudinejad and M. Avazpour, Enhanced degradation of sulfamethoxazole antibiotic from aqueous solution using Mn-WO3/LED photocatalytic process: Kinetic, mechanism, degradation pathway and toxicity reduction, Chem. Eng. J., 2020, 380, 122497 CrossRef CAS.
- K. Zheng, Y. Sun, S. Gong, G. Jiang, X. Zheng and Z. Yu, Degradation of sulfamethoxazole in aqueous solution by dielectric barrier discharge plasma combined with Bi2WO6 -rMoS2 nanocomposite: Mechanism and degradation pathway, Chemosphere, 2019, 222, 872–883 CrossRef CAS.
- E. M. El Mouchtari, L. Bahsis, L. El Mersly, H. Anane, S. Lebarillier, A. Piram, S. Briche, P. Wong-Wah-Chung and S. Rafqah, Insights in the Aqueous and Adsorbed Photocatalytic Degradation of Carbamazepine by a Biosourced Composite: Kinetics, Mechanisms and DFT Calculations, Int. J. Environ. Res., 2021, 15, 135–147 CrossRef CAS.
|
This journal is © The Royal Society of Chemistry 2024 |
Click here to see how this site uses Cookies. View our privacy policy here.