Improved microbial water quality and ozone performance following coagulation: implications for carbon based advanced treatment for potable reuse†
Received
31st July 2024
, Accepted 1st October 2024
First published on 1st November 2024
Abstract
To facilitate broader implementation of potable reuse, it is important to fully account for pathogen log10 reduction values (LRVs), including unit processes that are historically uncredited or under-credited. Despite its potential for pathogen removal, coagulation coupled with flocculation (C/F) has historically been omitted or overlooked when pursuing credits for potable reuse. However, with greater implementation of carbon-based advanced treatment (CBAT), which utilizes a combination of ozone, biofiltration, and granular activated carbon treatment as an alternative to membrane treatment (i.e., reverse osmosis), C/F may emerge as a valuable unit process for achieving improvements in water quality, operational performance, and public health protection in potable reuse systems. This study evaluated the ability of C/F with ferric chloride to improve both bulk and microbial water quality of secondary wastewater effluent and improve downstream ozone performance. This study also evaluated potential surrogates for microbial removal during C/F treatment. C/F removed 17–54% of DOC with ferric doses ranging from 10–50 mg Fe per L, with 30 mg Fe per L sufficient for meeting TOC removal requirements from the Stage 1 D/DBPR for all evaluated secondary effluents. Coagulant doses of 30 mg Fe per L obtained LRVs ranging from 2–3 for MS2 and B. subtilis spores. MS2 and B. subtilis spore removal exhibited strong (r ≥ 0.8) and significant (p < 0.05) Pearson's correlation with the removal of intact cell counts and total cell counts via flow cytometry (FCM), DOC, total adenosine triphosphate (ATP), and intracellular ATP. C/F immediately preceding ozone treatment improved inactivation of B. subtilis spores, lowered applied ozone doses, and increased ozone exposure (Ct) for similar specific ozone doses as compared to secondary effluent without C/F pre-treatment. Overall, C/F with ferric chloride was determined to be a valuable treatment step for removal of dissolved organic matter, MS2 bacteriophage, B. subtilis spores, and improvement of downstream ozone treatment. Furthermore, FCM, ATP, and DOC were determined to be strong potential candidates as surrogates for microorganism removal during C/F treatment, although further testing with pathogens is still necessary to justify LRV crediting.
Water impact
This work demonstrated the ability of coagulation to improve overall water quality of secondary effluents, remove spiked viruses and bacterial endospores, achieve correlation of microbial removal with rapidly measured surrogates, and improve the performance of downstream ozone treatment. These data are necessary for greater implementation of coagulation in carbon-based advanced treatment schemes for potable reuse.
|
1. Introduction
In the past decade, interest in advanced wastewater treatment (AWT) and potable reuse has grown significantly in the United States (U.S.) and in Europe, particularly in Switzerland.1 A critical priority for any AWT or potable reuse system is minimizing pathogen exposure through robust multi-barrier treatment that integrates physical removal and disinfection. In the U.S., indirect potable reuse (IPR) and direct potable reuse (DPR) guidelines and regulations include strict criteria for pathogen log10 reduction values (LRVs) that must be carefully demonstrated and monitored.2 For example, California's DPR regulations specify LRVs of 20, 14, and 15 for viruses, Giardia cysts, and Cryptosporidium oocysts, respectively.3 Achieving such LRV targets necessitates implementation of reverse osmosis-based advanced treatment (RBAT) and/or carbon-based advanced treatment (CBAT), both of which have extensive design, capital, and operational costs. CBAT generally includes ozone, biofiltration, and granular activated carbon (GAC) as key unit processes, although other processes can be included to improve overall treatment train performance or achieve specific criteria. To facilitate broader adoption of potable reuse, it is important to fully account for LRVs throughout these AWT trains. This includes unit processes that are historically uncredited or under-credited, such as coagulation/flocculation (C/F) with or without sedimentation and sub-residual ozone applications.
In the U.S., pathogen LRV targets and credits are well established for drinking water treatment systems through various guidance manuals and regulations.4,5 The C/F process has been demonstrated to remove viruses and other pathogens from source waters in addition to its more common treatment targets such as turbidity and dissolved organic matter (DOM).6–12 Because of this, U.S. drinking water systems are credited with LRVs of 2.5 to 3.0 for Cryptosporidium, 2.0 to 2.5 for Giardia, and 1.0 to 2.0 for viruses with direct filtration (i.e., coagulation, flocculation, filtration) and conventional filtration (i.e., coagulation, flocculation, sedimentation, and filtration), respectively.4,5
Despite its potential for pathogen removal, C/F has historically been omitted or overlooked when pursuing LRV credits for potable reuse. However, with greater implementation of CBAT, C/F may emerge as a valuable unit process for achieving improvements in water quality, operational performance, and public health protection in potable reuse systems. For example, removal of bulk organic matter can be a challenge in IPR/DPR systems seeking to avoid RBAT, which highlights an opportunity for an ‘enhanced’ C/F process. As defined by the Stage 1 Disinfectants and Disinfection Byproducts Rule (D/DBPR) in the U.S., enhanced coagulation refers to C/F processes targeting removal of dissolved organic matter (DOM), often by decreasing pH and/or applying a coagulant dose beyond what is optimal for particle removal.13 This approach has been demonstrated to yield improvements in downstream ozone performance in wastewater applications.14 C/F removes ozone-reactive DOM, which decreases ozone demand and decay, leads to greater dissolved ozone exposure (i.e., Ct), and justifies a higher pathogen LRV credit for a given applied ozone dose.15 More generally, lower levels of DOM may also reduce disinfection byproduct (DBP) formation during downstream disinfection processes.16
Treatment processes that are awarded pathogen LRV credits in potable reuse treatment trains are often described as critical control points (CCPs). Removal or inactivation at a CCP must initially be demonstrated for the pathogen target itself, but it is also necessary to frequently and rapidly monitor performance during long-term operation. The most accurate approach would be to directly analyze the fate of the pathogen(s) of interest. However, current methodologies for directly monitoring pathogen concentrations require timelines ranging from hours (PCR-based techniques) to weeks (cultivation), and these methods require skilled technicians, potentially large sample volumes, and a range of labor-intensive sample processing steps. Therefore, LRV crediting often involves identification of one or more surrogate water quality parameters that correlates to pathogen attenuation and can be rapidly analyzed to demonstrate treatment process performance.
Flow cytometry (FCM) allows for rapid (<30 min) detection of bacterial cells, and some studies also highlight its potential to rapidly detect virus-like particles.17,18 It can be implemented on-line, allowing for near-real-time assessment of microbial water quality.19–21 An additional microbial target amenable to rapid measurement is adenosine triphosphate (ATP). ATP is a molecule that provides energy to cells during cellular metabolism, the measurement of which can provide an estimate of cellular activity in an environmental sample. There are several commercialized methods for rapid estimation of ATP concentrations on surfaces and in aqueous samples, ranging from grab sample tests (Hygiena, LuminUltra) to on-line measurements (Hach). ATP measurements take anywhere from 15 seconds to 15 minutes, depending on the method used. Previous studies have demonstrated the ability of FCM cell counts and ATP to monitor the disinfection of autochthonous bacteria during ozone treatment of drinking water22 and wastewater effluents.23 However, the applicability of these methods to C/F has yet to be demonstrated.
The objectives of this study were as follows: (i) evaluate the performance of C/F with ferric chloride for treatment of secondary wastewater effluent based on common water quality parameters (e.g., DOM and turbidity) and removal of spiked MS2 bacteriophage and Bacillus subtilis spores; (ii) evaluate FCM, ATP, DOC, and UVA254 as surrogate measures of MS2 bacteriophage and B. subtilis spore removal; and (iii) evaluate the benefits of C/F on downstream ozone process performance. This study provides valuable information for utilities, regulators, and other stakeholders considering treatment options for potable reuse, particularly for inland communities where CBAT may be preferred over RBAT. The microbial removal data may help justify extension of drinking water-focused regulations/benchmarks for C/F to potable reuse applications, and the surrogate measurements may offer a means of CCP verification and future LRV crediting.
2. Experimental
2.1 Wastewater sample collection and storage
Testing was conducted in two phases (Fig. 1). For test one, secondary wastewater effluent was collected from full-scale water reclamation facilities located in Nevada (WWNV), Virginia (WWVA), and California (WWCA). Test 2 was solely conducted on secondary effluent from Virginia collected on a different date (WWVA-2). All three treatment plants receive wastewater primarily from municipal sources, with limited industrial inputs. All three plants are nitrified and either fully or partially denitrified via activated sludge processes. For the current study's sampling events, the three plants varied in their usage of coagulant at full-scale: WWCA added no coagulant, WWNV added ferric chloride at the headworks at 6 mg Fe per L, and WWVA added ferric chloride to the mixed liquor entering the secondary clarifiers at concentrations ranging from 5–20 mg Fe per L (depending on plant flow). Secondary effluent was shipped overnight to the laboratory at Southern Nevada Water Authority and stored for less than one week at 4 °C until experimentation. Testing with each individual water was conducted within the same day after equilibrating to room temperature. Initial characterizations of wastewater samples are provided in Table 1.
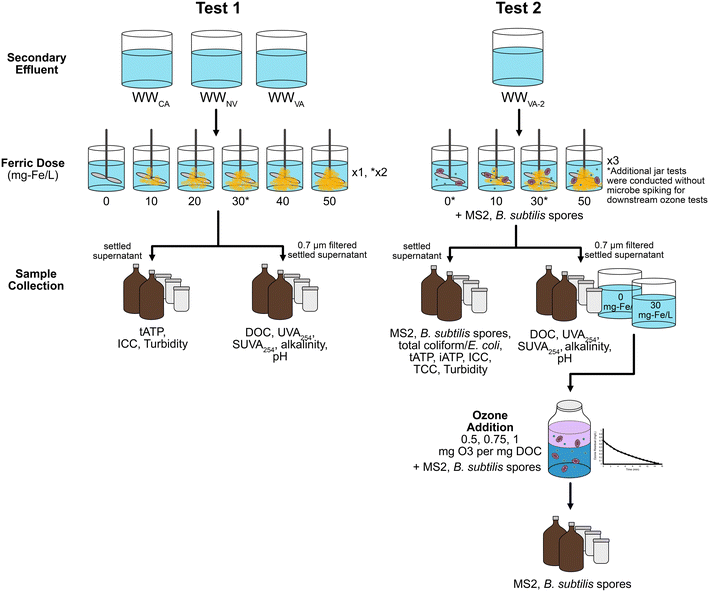 |
| Fig. 1 Overview of the experimental set up. Test 1 focused on bulk water quality changes due to coagulation/flocculation with ferric chloride across three secondary effluents from different locations in the United States (Nevada, Virginia, and California). Test 2 evaluated the removal of spiked microorganisms by coagulation/flocculation with ferric chloride on a single secondary effluent from Virginia. Additionally, the secondary effluent from Virginia was treated with either 0 or 30 mg Fe per L and subsequently filtered, spiked with microorganisms, and ozonated to evaluate the impact of upstream coagulation/flocculation on ozone treatment. | |
Table 1 Secondary wastewater effluent characterization
Wastewater |
pH |
DOC (mg C per L) |
UVA254 (cm−1) |
Alkalinity (mg L−1 as CaCO3) |
Bromide (μg L−1) |
Ammonia (mg N L−1) |
Nitrate (mg N L−1) |
Nitrite (mg N L−1) |
Total phosphate (mg P L−1) |
ND = not determined. |
Nevada (WWNV) |
7.5 |
6.3 |
0.116 |
123 |
189 |
0.71 |
11.9 |
0.084 |
0.120 |
Virginia-1 (WWVA) |
6.9 |
6.7 |
0.148 |
127 |
241 |
0.06 |
0.8 |
<0.020 |
0.046 |
California (WWCA) |
7.7 |
9.1 |
0.173 |
126 |
214 |
1.00 |
3.1 |
0.518 |
0.056 |
Virginia-2 (WWVA-2) |
7.0 |
7.0 |
0.144 |
107 |
ND |
0.11 |
0.8 |
<0.020 |
ND |
2.2 Target microorganisms and surrogate parameters
MS2 bacteriophage and Bacillus subtilis spores were used in spiking experiments as surrogates for enteric viruses and encysted protozoan parasites, respectively. MS2 is a single-stranded RNA, Escherichia coli-infecting bacteriophage. It has an icosahedral capsid that is roughly 20–30 nm in diameter, has an isoelectric point of 3.1–3.9,24 and is hydrophobic in nature.25 It is commonly used as a surrogate for human enteric viruses due to its ease of use and non-pathogenicity. B. subtilis spores are bacterial endospores that have been proposed as surrogates for protozoan pathogens, particularly Cryptosporidium oocysts. The spores are oval shaped, generally 1 μm × 2 μm in size, have an isoelectric point of <3.0,26 and are also hydrophobic.27 Additionally, removal of indigenous total coliforms and E. coli was evaluated. Total and intracellular ATP were measured to rapidly estimate changes in microbial activity. Total cell counts (TCC) and intact cell counts (ICC) (i.e., limited or no cellular membrane damage) were analyzed by FCM as potential surrogates for C/F process efficacy.
2.3 Cultivation and enumeration of MS2 bacteriophage and B. subtilis spores
MS2 stock solutions were propagated by growing ∼104 plaque forming units (PFU) on an E. coli ATCC 15597 lawn using the double agar overlay method28 on tryptic soy agar (TSA) plates. MS2 was extracted from plates by applying ∼7 mL of phosphate-buffered saline (PBS) to each plate and allowing to sit for ∼2 hours at room temperature. PBS was collected from the plates and centrifuged at 3500 × g for 15 min to remove cellular debris. The supernatant was passed through a 0.22 μm filter (SteriFlip) and stored at 4 °C until use. Enumeration of MS2 was performed using the double agar overlay method with E. coli ATCC 15597 as the bacterial host, TSA plates, and soft agar composed of tryptic soy broth (TSB) supplemented with 0.8% agar.
B. subtilis spore stock solutions were prepared by transferring a single colony of B. subtilis ATCC 3366 maintained on a TSA plate into ∼50 mL TSB, which was then incubated overnight at 37 °C. Overnight cultures were washed twice in 50 mL of PBS, with centrifugation at 3500 × g for 10 min. Several TSA plates were then inoculated with the resulting PBS solution and incubated for 5–7 days at 37 °C to induce sporulation. B. subtilis spores were extracted from plates by applying ∼7 mL of PBS to each plate and allowing to sit for ∼2 hours at room temperature. PBS was collected from each plate and washed twice, with centrifugation at 3500 × g for 10 min. The final spore suspension in PBS was heat treated (80 °C for 20 min) to inactivate any vegetative cells. The heat treatment was also repeated immediately prior to spiking experiments. B. subtilis spores were enumerated after additional heat treatment (80 °C for 20 min) and subsequent spreading on TSA plates.
2.4 Coagulation/flocculation
Coagulation was performed with ferric chloride on 1.5 L wastewater aliquots following methods described in Wert et al. (2011).14 An 80 g Fe per L working solution of ferric chloride was prepared from a 40% ferric chloride stock (Kemira PIX-311). Coagulant doses varied by experiment (0–50 mg Fe per L) and are described in Fig. 1. Coagulant was added to secondary effluent immediately preceding jar testing with a six-paddle programmable jar tester (Phipps & Bird PB900, VA, USA). Sample mixtures were rapidly mixed for 2 min at 100 rpm (G = ∼130 s−1), followed by 20 min of flocculation at 20 rpm (G = ∼13 s−1) and settling for 30 min. A portion of settled water was carefully decanted to avoid disturbing or resuspending the settled floc for subsequent analysis (microbial analysis, turbidity), while the remainder of the settled water was filtered sequentially through 1.5 and 0.7 μm glass microfiber filters (Whatman GF/A and GF/F filters, respectively) for the remainder of the analyses and also for subsequent ozone treatment. Filtration was not evaluated for microbial removal as part of this study. For MS2 and B. subtilis spiking tests, organisms were spiked into each individual jar to achieve a concentration of 106 PFU per mL and 105 CFU per mL, respectively, prior to ferric chloride addition. Microorganisms were spiked in small volumes, 100 to 1000 μL for MS2 and B. subtilis spores, respectively, resulting in an added DOC of <1 mg C per L.
2.5 Batch ozonation
Bench-scale ozone tests were performed by generating a high concentration ozone stock solution using methods described in Wert et al. (2011).14 Briefly, gaseous ozone is diffused through a 4 L reactor filled with Milli-Q water chilled to 4 °C to achieve final aqueous ozone concentrations ranging from 60–70 mg L−1. Aliquots from the stock ozone solutions were added to 1 L batch reactors containing either secondary effluent without C/F pretreatment or secondary effluent pretreated with 30 mg Fe per L ferric chloride. All secondary effluents (with and without C/F pretreatment) were filtered prior to ozonation and re-spiked with MS2 and B. subtilis spores. Ozone aliquots were selected to target specific ozone doses of 0.5, 0.75, and 1.0 mg O3 per mg DOC (Fig. 1). Dissolved ozone residual was measured after contact times of 0.5, 1, 1.5, 2, 4, 6, 8, and 10 minutes, and an ozone residual profile was generated to calculate ozone exposure (Ct) according to the extended log integration method,29 although simplified due to not having to account for hydraulic implications when using batch reactors. Briefly, ozone decay rates were calculated from the slope of the natural log (ln) transformed ozone residuals vs. time, and Ct was calculated with the following equation:
, where C0 is initial ozone residual (mg L−1), k is the ozone decay rate constant (min−1), and t is the amount of time until ozone was fully dissipated (min). The time (t) for ozone to fully dissipate was estimated based on the ozone demand/decay curve, and samples were allowed to sit beyond this time to assure that all ozone had been depleted prior to sample collection. Applied specific ozone doses (i.e., O3/DOC) were calculated after accounting for the dilution caused by ozone stock addition (∼4–8%) as well as any increase in DOC due to spiked organisms (≤1 mg C per L). Hydroxyl radical exposure was estimated during batch ozone tests through the addition of the probe compound para-chlorobenzoic acid (pCBA).30 Hydroxyl radicals formed during ozone reaction and decomposition contribute to micropollutant abatement and to the formation of DBPs (i.e., bromate), although they play little to no role in disinfection.31
2.6 Analytical methods
Indigenous total coliforms and E. coli were enumerated using Colilert-24 with Quanti-Tray/2000 (IDEXX). TCC and ICC were determined by FCM in units of events per μL using the techniques described in Gatza et al. (2013).32 Briefly, samples were stained with either 1× SYBR Green (Invitrogen) for the TCC or a combination of 1× SYBR Green and 0.3 mM of the counterstain propidium iodide (Invitrogen) to isolate the ICC. The stained samples were incubated at 37 °C for 10 minutes prior to FCM using a BD Accuri C6 Plus with an FL1-H acquisition threshold of 800. Specific gating information can be found in Gatza et al. (2013).32 Total and free (i.e., extracellular) ATP (tATP and fATP) were measured using Hygiena Aquasnap Total and Free ATP test devices with a Hygiena SystemSURE luminometer (Camarillo, CA, USA) in relative light units (RLU) which are then converted to pg ATP using guidance from Hygiena (personal communication). Intracellular ATP (iATP) was calculated using the following formula: iATP = tATP − fATP.
UV254 absorbance (UVA254) was measured using a Hach DR 6000. pH was measured using an Accumet portable AP110 pH probe (Fisher Scientific). Turbidity was measured using a Hach 2100N Turbidimeter. DOC was measured following Standard Methods 5310B. Specific UV absorbance (SUVA254) was determined using the following equation:
Alkalinity was measured following Standard Methods 2320B. Ammonia and total phosphate were measured following Standard Methods 4500, and nitrite/nitrate was measured using EPA method 300. Ozone residuals were measured using the indigo method described in Standard Methods 4500-O3. pCBA was measured using the LC-MS/MS method described in Vanderford et al. (2007).33
2.7 Statistical analysis
Statistical analysis was performed using R (version 4.2.2) with α = 0.05 for all analyses. To evaluate if different wastewaters impacted treatment efficiency, a type III analysis of covariance (ANCOVA) was performed, using the R library car, with post hoc Tukey's analysis using the R library multcomp. To determine if there were significant differences in removal of microorganisms and other water quality parameters between the different coagulant doses, analysis of variance (ANOVA) with post hoc Tukey's analysis was implemented. Pearson's r correlation analysis was performed using the rcorr function from the R library Hmisc.
3. Results and discussion
3.1 Coagulation of three secondary effluents (test 1)
3.1.1 General water quality changes.
DOC removal ranged from 17% to 54% for ferric chloride doses ranging from 10 to 50 mg Fe per L (Fig. 2a), in agreement with Wert et al. (2011).14 Because of the consistent linear increase in DOC removal, a point of diminishing return, as defined by the U.S. Environmental Protection Agency,34 was not apparent for any of the secondary effluents within this ferric dosing range. UVA254, which can be interpreted as an estimate of the aromatic portion of DOM, exhibited similar removal trends to that of DOC (Fig. 2b). There were no statistically significant differences in DOC removal efficiency between WWVA and WWCA (p > 0.05), however DOC removal was significantly lower for WWNV (p = 0.001). UVA254 removal from WWVA was significantly higher (p < 0.001) when compared to WWCA or WWNV. It is currently unclear what caused these differences, but they may be attributable to the effluent organic matter (EfOM) composition and susceptibility to removal via coagulation. As mentioned earlier, WWNV and WWVA had been previously treated with ferric chloride in the respective full-scale plants prior to sample collection. Additionally, there were differences in the upstream activated sludge treatment processes, with the extent of denitrification varying as follows: WWNV < WWCA < WWVA. It is worth noting that despite statistical significance, the differences in removal for DOC and UVA254 were relatively low between wastewaters. Because DOC and UVA254 were removed to the same relative extent, SUVA254 remained relatively constant across the various ferric doses (Fig. 2c). Raw values for DOC, UVA254, and SUVA254 are provided in Table S1.†
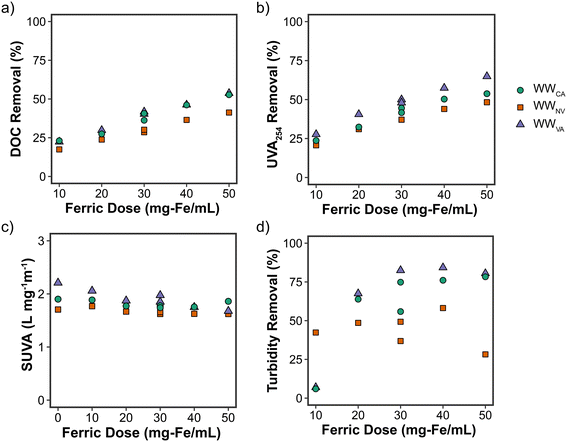 |
| Fig. 2 Percent removals of (a) dissolved organic carbon (DOC), (b) UV254 absorbance (UVA254), (c) specific UV254 absorbance (SUVA), and (d) turbidity during enhanced coagulation of different secondary effluents. | |
Turbidity removal (Fig. 2d) ranged from 6% to 84% and generally increased with increasing ferric dose for WWVA and WWCA. It is currently unclear why turbidity removal for WWNV appeared to establish a point of diminishing return at the lowest dose of 10 mg Fe per L before exhibiting a spike in turbidity at 50 mg Fe per L (Table S1†), particularly when considering the consistent removal of the other water quality parameters (DOC, UVA254, among others). This could potentially be due to WWNV already having been treated with ferric at the headworks, although this same effect was not exhibited in WWVA which also dosed ferric at full-scale. Additional water quality data related to coagulation (e.g., pH and alkalinity) are provided in Table S1;† as expected for enhanced coagulation with metal salts,35 higher ferric chloride doses resulted in lower pH values (total drop ranging from 0.6 to 1.6 pH units at 50 mg Fe per L, depending on the secondary effluent) and considerable consumption of alkalinity (91–95% removal at 50 mg Fe per L). The target pH post-coagulation for waters with alkalinities greater than 120 mg L−1 is 7.0,34 and each of the secondary effluents either met this criterion (WWCA, WWNV), or were slightly below it (WWVA) at a ferric dose of 30 mg Fe per L. WWVA was already at the target pH prior to coagulation.
Overall, effective removal of organics in secondary effluent was demonstrated with coagulation using ferric chloride, which has implications for TOC/DOC removal targets in potable reuse applications and downstream DBP formation during primary/secondary disinfection.36 As recommended in the Stage 1 D/DBPR, enhanced coagulation specifically targeting TOC removal (in addition to turbidity) can be implemented to minimize DBP formation during drinking water treatment. If a framework similar to the Stage 1 D/DBPR was applied to potable reuse, 30 mg Fe per L would have been sufficient for each secondary effluent to meet the specified TOC removal requirements based on the data from this study (i.e., at least 25% for WWNV and WWVA and 30% for WWCA due to its higher initial TOC).
3.1.2 Removal of total ATP and intact cells (via flow cytometry).
Removal trends for tATP were similar between each wastewater (p > 0.05), exhibiting increased LRVs with increasing ferric doses and ultimately achieving LRVs of 3–4 with 50 mg Fe per L (Fig. 3a). Removal of tATP was significantly (p < 0.05) correlated with removals of DOC and UVA254 for all three secondary effluents (Fig. S1a and b†). Removal of tATP was only significantly (p < 0.05) correlated with turbidity for WWCA (Fig. S1c†).
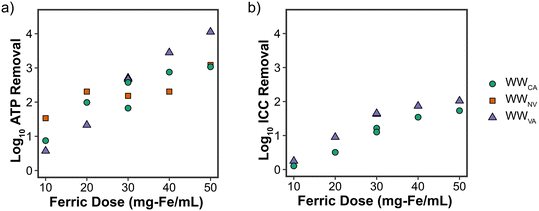 |
| Fig. 3 log10 removals of (a) total ATP (tATP) and (b) intact cell count (ICC) as determined by flow cytometry, during enhanced coagulation of different secondary effluents. Note: ICC was not measured in Nevada effluent. | |
LRVs based on ICC were positively correlated with ferric dose for WWVA and WWCA (Fig. 3b), albeit to a lesser magnitude when compared to tATP. ICC removal was not measured for WWNV. Strong (r > 0.9) and significant (p < 0.05) correlations between ICC and DOC, UVA254, and turbidity were observed (Fig. S1d and f†). ICC removal did exhibit minor tailing at ferric doses greater than 30 mg Fe per L, a deviation from tATP removal, which suggests a point of diminishing return specific to ICC removal. Despite the tailing, a strong (r > 0.9) correlation between tATP and ICC removal was determined (Fig. S2†). The slope of the line is greater than one, indicating that ICC was more conservatively removed than tATP, potentially making ICC more valuable for estimating removal of microbial constituents in full-scale applications.
3.2 Coagulation of secondary effluent with spiked microorganisms (test 2)
3.2.1 General water quality changes.
Ferric doses of 10, 30, and 50 mg Fe per L were used to treat WWVA-2 spiked with MS2 bacteriophage and B. subtilis spores. Removals for DOC and UVA254 diminished beyond ferric doses of 30 mg Fe per L (Fig. S3a†), contrary to the results from test 1 (Fig. 2). In fact, DOC reached a point of diminishing return (PODR) between 30 and 50 mg Fe per L based on the USEPA definition,34 and UVA254 actually increased when the ferric chloride dose increased from 30 to 50 mg Fe per L, although there was still an overall reduction in UVA254 relative to the untreated secondary effluent. Furthermore, turbidity levels increased by nearly 300% at 50 mg Fe per L (Fig. S3b†), which was unexpected. While there was some variability in turbidity removals between wastewaters during test 1 (Fig. 2c), all ferric chloride dosing conditions still resulted in overall reductions in turbidity. Direct comparison of DOC, UVA254, SUVA254, and turbidity between the two tests with WWVA and WWVA-2 highlights the discrepancy at 50 mg Fe per L (Fig. S4a–c†).
Based on these results, it appears that 50 mg Fe per L resulted in sub-optimal, or off-specification, coagulation during this particular test, despite successful coagulation at this dose during test 1 (Fig. 2). A significant drop in pH occurred at 50 mg Fe per L (pH = 3.4) during test 2 (Table S2†), which did not occur during the first test (pH = 6.3), despite similar initial pH (pH = 7). This may have been due to lower initial alkalinity in test 2 (107 vs. 127 mg L−1 as CaCO3), which caused alkalinity to drop below the limit of detection after dosing with ferric chloride at 50 mg Fe per L. In the absence of alkalinity, ferric chloride will produce hydrochloric acid during initial hydrolysis, which lowers solution pH.35 Extremely low pH and high ferric doses could potentially lead to re-stabilization of particles, which could in part explain the increased turbidity. Another potential explanation is that some of the dissolved ferric persisted through 0.7 μm filtration with 50 mg Fe per L in test 2, which may impact pH, turbidity (due to issues with floc settling), and UVA254.37 Regardless of the cause, a pH of 3.4 is well below the target pH for a water with alkalinity between 60 and 120 mg L−1 as CaCO3,34 and such an impractically low pH would undoubtedly cause problems for downstream treatment. These results highlight the necessity of preliminary jar testing as described in the USEPA Coagulation and Softening Manual to determine optimal doses for the water of interest.34
3.2.2 Removal of spiked and naturally occurring microorganisms.
Removals of spiked MS2, spiked B. subtilis spores, and naturally occurring total coliforms and E. coli during test 2 are summarized in Fig. 4a. Both spiked microorganisms were effectively removed with increasing ferric doses. Despite off-specification coagulation at 50 mg Fe per L, removals greater than 3
log10 for each organism were observed. One-way ANOVA indicated that B. subtilis spore removal at a ferric dose of 50 mg Fe per L (LRV ≈ 3.5) was significantly higher than at 10 and 30 mg Fe per L (LRV ≈ 1.6 and 2.5, respectively). MS2 removal at 30 mg Fe per L (LRV ≈ 2) was significantly higher than at 10 mg Fe per L (LRV ≈ 1) but similar to 50 mg Fe per L (LRV ≈ 2). As a point of reference, the LRVs of 2 to 3 for MS2 and B. subtilis spores achieved with 30 mg Fe per L would be comparable to or greater than the typical LRV credits awarded to RO for viruses, Giardia, and Cryptosporidium in potable reuse applications, at least when RO membrane integrity is evaluated based on electrical conductivity or TOC.3,38
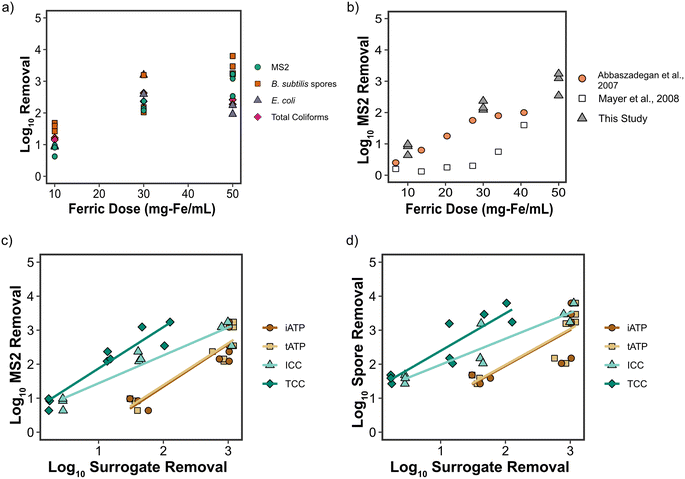 |
| Fig. 4 log10 removals of spiked microorganisms (a) MS2 bacteriophage, B. subtilis spores, E. coli, and total coliforms with increasing ferric chloride dose; (b) MS2 bacteriophages with increasing ferric chloride doses compared to other bench-scale spiking studies; and (c) MS2 bacteriophage and (d) B. subtilis spore removal with respect to removals of rapid microbial surrogate parameters. | |
Based on the data available, it is unclear whether the MS2 and B. subtilis spore LRVs at 50 mg Fe per L were due solely to physical removal, or potentially due to the low pH condition (i.e., pH = 3.4). A pH of 3.4 is near the isoelectric point for MS2 (pI = 3.1–3.9), which could cause significant aggregation of virus particles and thus lower perceived PFUs during plating.39,40 The low pH may also impact the viability of MS2, although there is limited evidence demonstrating the impact of pH on MS2 decay, particularly within the timeframe of this test (<4 hours at low pH).41 Given the short contact times at low pH in this study, it is more likely that the decrease in PFU at pH 3.4 would be due to aggregation and physical removal (via coagulation) than inactivation. For B. subtilis spores, which have a lower isoelectric point (pI < 3.0) than MS2 and a similar ability to withstand gastric conditions,42–44 it is unlikely that low pH is a contributing factor in B. subtilis spore removal/inactivation. In other words, coagulation is likely the primary contributor to spore removal even at 50 mg Fe per L.
MS2 removals demonstrated in this study were consistent with Abbaszadegan et al. (2007) and greater than those determined in Mayer et al. (2008) (Fig. 4b), both of which focused on ferric chloride coagulation but for surface water rather than secondary effluent.45,46 While virus removal is lower in Mayer et al. (2008), it appears that DOC removal is similarly lower in that study when compared to Abbaszadegan et al. (2007) and this study, indicating that DOC removal may be a useful surrogate for virus removal during coagulation.45,46 There are few published studies on B. subtilis spore removal during coagulation with ferric salts. Rice et al. (1996) was able to demonstrate LRVs of 1.5 to 2.0 for B. subtilis spores in pond water using ferric doses ranging from 20–40 mg Fe per L, which is consistent with the results in the current study.47 In a pilot study conducted by Dugan et al. (2001), ferric chloride coagulation of raw river water with ∼5 mg Fe per L achieved an LRV of ∼1.0 for aerobic endospores (i.e., Bacillus and other aerobic endospore-forming bacteria).10 Therefore, it appears that coagulation with ferric salts can effectively remove spores from a wide range of environmental water matrices.
In addition to spiked microorganisms, removal of naturally occurring E. coli and total coliforms was also analyzed (Fig. 4a). In contrast to MS2 and B. subtilis spore removal, total coliforms and E. coli appeared to follow UVA254/turbidity removal trends more closely, with diminished removals at 50 mg Fe per L. Because only duplicate total coliform and E. coli tests were performed, statistical significance between treatments was not determined. Nonetheless, LRVs of between 2 and 3 were achieved for a ferric chloride dose of 30 mg Fe per L. Pritchard et al., (2010) reported E. coli LRVs of less than 1 for a ferric dose of 10 mg L−1, similar to the current study, although it is unclear if the coagulant was dosed on a mass-Fe basis.48 Furthermore, bench-scale data from Chalapati Rao et al. (1988) demonstrated LRVs of 0.7 to 1.1 for total coliforms with 8 mg Fe per L.7 Samples taken from a full-scale drinking water treatment facility applying a coagulant dose of 3 mg Fe per L were able to demonstrate an LRV of 2.2 for total coliforms,8 higher than anticipated from the current study, though pH and other water quality characteristics could impact results. Overall, comparison of microbial removals during coagulation from different studies can be difficult due to differences in coagulant type, dosing amount and nomenclature, method of application, matrix pH, and other water quality characteristics that may interfere with removal capabilities. Despite this, removals from this study are generally consistent with other studies for each of the observed microorganisms.
In the USEPA's Surface Water Treatment Rule (SWTR), higher LRVs are awarded for treatment trains that utilize filtration after coagulation/flocculation/sedimentation (C/F/S).5 Evaluations of ferric C/F/S combined with subsequent microfiltration have repeatedly demonstrated higher removals for viruses and protozoan pathogens when compared to C/F/S alone.9,10,12,49,50 This is due to microfiltration achieving better removal of smaller flocs that do not settle efficiently under typical sedimentation conditions. This is illustrated by Zhu et al. (2005) and Meyn et al. (2011), in which greater removals of MS2 were demonstrated at lower ferric doses when compared to results from this study.49,50 Nasser et al. (1995) compared MS2 removal from filtration with and without prior coagulation with alum and polyelectrolytic cations.9 Poor removal was determined from filtration alone, whereas C/F with filtration resulted in an LRV of 2—one log10 higher than with C/F alone. Dugan et al. (2001) and States et al. (1997) both demonstrated lower occurrences of protozoan pathogens or endospores in the resulting filtrate following C/F/S and filtration than from the settled supernatant following C/F/S.10,12 Removal values demonstrated in this study may potentially be enhanced when followed by filtration processes, in line with the SWTR.
3.2.3 Rapid surrogate removal.
ATP and cell counts via flow cytometry were analyzed to compare their removals against the target microorganisms, as well as to compare against corresponding data from test one. tATP and iATP (Fig. S5†) approached their limits of detection (LoD) at 30 mg Fe per L, resulting in LRVs of ∼3, and they were removed beyond their LoDs at 50 mg Fe per L. Overall, ATP removal values were consistent with previous experiments (Fig. 3a) and trend similarly to MS2 and B. subtilis spore removal for 10 and 30 mg Fe per L (Fig. 4a, c and d). Removals based on FCM data, particularly ICC, increased significantly (p < 0.05) with increasing ferric doses. In contrast with DOC, turbidity, and indigenous coliform bacteria, but consistent with spiked MS2 and B. subtilis spores, ICC and TCC removals were less affected by the off-specification event at 50 mg Fe per L. At 50 mg Fe per L, ICC removal was approximately 1
log10 greater in test 2 relative to test 1 (Fig. 3b). One possibility is potential cellular inactivation, via membrane damage, occurring at this dose, which is supported by a shift in intact cell to dead cell log10 ratio (Fig. S6†). This could potentially be due to intolerance of certain bacterial communities to low pH, as the composition of secondary effluent bacterial communities would likely be a mixture of gastrointestinal organisms (tolerant to low pH) and environmental organisms from the sewer collection and wastewater treatment systems (potentially more sensitive to low pH). This may also explain the deviation from E. coli and total coliform results (Fig. 4a). Coliforms, including E. coli, are tolerant to low pH due to the enteric nature of the organism51 and therefore significant inactivation at low pH would not be expected.
3.2.4 Use of surrogate parameters to predict microorganism removal.
Beyond its ability to consistently achieve pathogen removal, another important criterion for coagulation to be recognized as a valid CCP is the potential for LRV verification. For this reason, various surrogate parameters that are amenable to rapid, on-line monitoring were evaluated via Pearson's correlation (r).
Correlation analysis was performed on the full range of ferric doses in test 2, despite off-specification coagulation at 50 mg Fe per L (Fig. 5a). MS2 removal exhibited strong (r ≥ 0.8) and significant (p < 0.05) Pearson's correlation with the removal of ICC (r = 0.95), TCC (r = 0.95), DOC (r = 0.94), tATP (r = 0.93), and iATP (r = 0.92). B. subtilis spore removal exhibited strong and significant Pearson's correlation to ICC (r = 0.92), TCC (r = 0.90), DOC (r = 0.84), tATP (r = 0.84), and iATP (r = 0.84). Total coliforms were strongly and significantly correlated with tAPT (r = 0.97), iATP (r = 0.97), DOC (r = 0.95) and TCC (r = 0.84), whereas E. coli only correlated strongly with both iATP and tATP (r = 0.85 and r = 0.84, respectively). DOC, or TOC, is a critical parameter in water reuse systems, particularly for CBAT, and is already integrated as an on-line parameter in most systems. On-line monitoring capabilities of FCM (ICC/TCC) have been successfully demonstrated,19,20 and on-line ATP monitors already exist on the market (e.g., Hach EZ7300 Microbial Activity ATP Analyzer). Despite this, neither on-line FCM nor ATP have been broadly implemented.
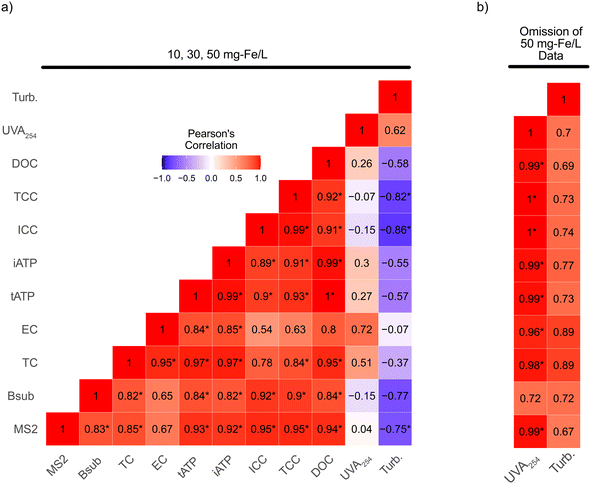 |
| Fig. 5 Pearson's correlation values between (a) different measured variables after coagulation of secondary effluent at ferric doses ranging 10–50 mg Fe per L and (b) UVA254 and turbidity with the omission of 50 mg Fe per L. Correlations with red values indicate positive correlations, blue values indicate negative correlations, color intensity indicates the strength of the correlation value, and Pearson's r is provided within each box. Values with * indicate a significant correlation at p < 0.05. UVA254 = absorbance at 254 nm, DOC = dissolved organic carbon, TCC = total cell count, ICC = intact cell count, iATP = intracellular ATP, tATP = total ATP, EC = Escherichia coli, TC = total coliforms, and Bsub = Bacillus subtilis spores. | |
UVA254 and turbidity removals were either weakly or inversely correlated to microbial parameters for test 2, contrary to test 1 and several previous drinking water studies which found both turbidity8,10,11 and UVA254
11 removal to be correlated to removal of viruses or protozoan pathogens. To determine if the poor correlations in test 2 were caused by the off-specification event at 50 mg Fe per L, a second correlation analysis was performed, excluding this particular dosing condition (Fig. 5b). Correlative relationships between UVA254 and turbidity increased for all parameters, indicating that the off-specification event likely impacted the ability of UVA254 and turbidity to accurately predict microbial removal. While these parameters may not provide accurate prediction of microbial removal during adverse conditions, they were able to detect what might be considered an off-specification or sub-optimal condition (i.e., particle re-stabilization or over-dosing of ferric), which is critically important in the context of public health protection.
Based on test 2, FCM (ICC, TCC), ATP (iATP, tAPT), DOC, and potentially UVA254 appear to be strong candidates to serve as pathogen removal surrogates for coagulation in water reuse applications that require LRV verification. However, further testing, including more wastewaters, direct correlation with pathogen removal (i.e., enteric viruses, Giardia cysts, and Cryptosporidium oocysts), or at least a larger suite of potential indicator organisms, would be necessary to fully validate this approach. ICC was the only rapid microbial measurement that was consistently conservative in predicting the removals of MS2 bacteriophage, B. subtilis spores, and E. coli (Fig. S7†). MS2 has often been used as a surrogate for human enteric virus removal, particularly in reuse applications,52 but the addition of bacteriophages with different characteristics (isoelectric point, hydrophobicity, capsid structure, genome structure, etc.), or enteric viruses themselves, would strengthen the case for applying these surrogates as a means of crediting C/F treatment for virus LRVs. There are fewer studies evaluating the use of B. subtilis spores as an indicator of protozoan pathogen fate during potable reuse or even water treatment in general. There appear to be differences in the surface chemistry (isoelectric point, hydrophobicity) between B. subtilis spores and Cryptosporidium oocysts,53 which could potentially impact the utility of B. subtilis spores to predict protozoan pathogen removal for processes such as coagulation. Despite this, Dugan et al. (2001) found that aerobic endospores were strongly correlated to Cryptosporidium oocyst removal during coagulation.10 Further research is necessary to explore the ability of B. subtilis spores to accurately predict removal of protozoan pathogens such as Cryptosporidium oocysts.
3.3 Downstream inactivation by ozone
Inactivation of MS2 and B. subtilis spores by ozone following coagulation with 30 mg Fe per L of ferric chloride was evaluated at O3/DOC ratios ranging from 0.48–1.18 (after accounting for dilution from the ozone spike and DOC additions from the microbial stocks). Significant inactivation of MS2 (i.e., >4
log10) was demonstrated at all specific ozone doses, with and without upstream coagulation (Fig. 6a). O3/DOC ratios greater than 0.75 often achieved MS2 concentrations less than 1 PFU per mL and LRVs >6. The effect of upstream coagulation was more apparent for B. subtilis spore inactivation, with LRVs <1 for all ozone dosing conditions without pre-coagulation and LRVs up to 4 with pre-coagulation (Fig. 6b). Negligible inactivation of B. subtilis spores at an O3/DOC of 1.0 in secondary wastewater effluent was similarly demonstrated in Gamage et al. (2013).54
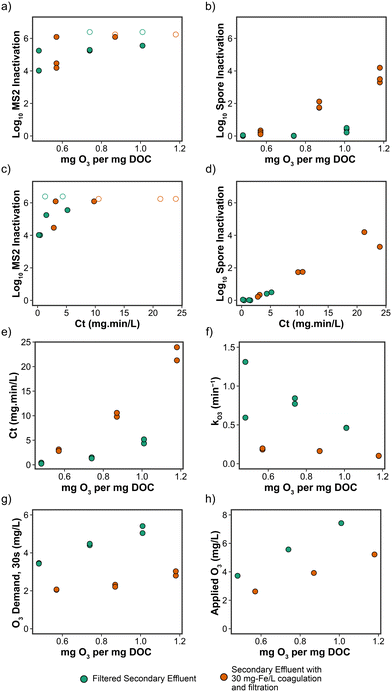 |
| Fig. 6 Ozone treatment of secondary effluent with and without pre-treatment by enhanced coagulation (30 mg L−1 FeCl3). Inactivation as a function of specific ozone dose is provided for (a) MS2 and (b) B. subtilis spores. Inactivation as a function of ozone exposure (Ct) is provided for (c) MS2 and (d) B. subtilis spores. The effects of pre-treatment on (e) ozone Ct, (f) ozone decay rate constant (kO3), (g) ozone demand at 30 s, and (h) applied ozone dose are provided as a function of specific ozone dose. Open points indicate removal beyond LOD (1 PFU or CFU per mL). | |
Based on this study and other published literature, ozone exposure (Ct) appears to be a primary driver of microbial inactivation during ozone treatment, particularly for bacterial spores. For example, Gamage et al. (2013) observed negligible levels of inactivation of B. subtilis spores when ozone was combined with hydrogen peroxide to drive advanced oxidation conditions (i.e., ozone conversion to hydroxyl radicals), which results in minimal ozone Ct.54 Similarly, Fig. 6c and d demonstrate that inactivation of MS2 and B. subtilis spores is directly dependent on ozone Ct, so any water quality changes that result in higher ozone Ct would be expected to yield improved ozone disinfection performance. Interestingly, samples with upstream coagulation achieved higher ozone Ct for similar O3/DOC ratios (Fig. 6e), which was driven by the lower ozone decay rate constants with upstream coagulation (Fig. 6f). Additionally, pre-coagulation with ferric chloride also significantly reduced the 30 s ozone demand for comparable O3/DOC ratios (Fig. 6g). Improvements in ozone stability in pre-coagulated and filtered secondary effluent could be due to the slight decrease in pH,30 or potentially due to selective removal of ozone-reactive organics during coagulation.14 Despite ozone exposure (or Ct) potentially being both a driver and reliable predictor of microbial inactivation, other metrics such as O3/DOC ratio (Fig. 6a and b) might still be useful surrogates for LRV crediting, particularly for viruses.
Hydroxyl radical exposure was lower in coagulated samples (Fig. S8a†), indicating that less ozone was being transformed to hydroxyl radicals, which would be expected with increased ozone stability. Hydroxyl radical exposures of non-coagulated samples are in agreement with those determined in Lee et al. (2013), however hydroxyl radical exposures for pre-coagulated samples are lower than those with a similar O3/DOC.55 The ratios of hydroxyl radical to ozone exposure (RCT) for each condition are provided in Fig. S8b.† As expected, RCT values decrease when secondary effluent is pre-coagulated, indicating an increase in ozone exposure/decrease in hydroxyl radical exposure. A decrease in RCT could have implications for DBP (i.e., bromate) formation during ozone treatment for potable reuse, as the interplay between hydroxyl radicals and ozone play an important role in bromate formation during ozonation of wastewater.56 Several bromate minimization strategies involve lowering the RCT, such as pH depression, monochloramine addition, and the upstream addition of HOCl during the chlorine–ammonia process.56 Therefore, it is probable that upstream coagulation with ferric chloride could aid in the minimization of bromate during ozone treatment.
Additionally, lowered hydroxyl radical exposures following C/F have resulted in lowered oxidation of ozone recalcitrant micropollutants,14 which should be taken into consideration. Furthermore, ozone treatment more generally can contribute to the formation of biodegradable organic transformation products,57 which is one of the reasons biofiltration is integrated into CBAT trains.58,59 Further studies of the impact of upstream C/F on DBP formation, micropollutant oxidation, and the formation of transformation products during ozonation are warranted.
Because of the decrease in DOC following coagulation, the amount of applied ozone necessary to achieve specific ozone doses also decreased (Fig. 6h). Therefore, similar ozone Ct and microbial inactivation can be achieved with lower applied ozone doses following C/F treatment, or greater ozone Ct and microbial inactivation for the same applied ozone dose. One potential drawback of utilizing low ozone doses for disinfection in potable reuse applications is the difficulty in measuring Ct due to rapid ozone decay (i.e., subresidual dosing applications). C/F pretreatment may result in sufficient dissolved ozone stabilization to allow for easier and more reliable monitoring of ozone Ct in low-dose applications. This might facilitate LRV crediting in the absence of alternative crediting frameworks.
4. Conclusion
Overall, this study demonstrates the ability of ferric chloride coagulation and flocculation (C/F) to improve the overall quality of treated wastewater effluent based on common water quality parameters, including turbidity, DOC, and UV254. In particular, the ability of C/F to remove DOC is critically important for potable reuse systems seeking to avoid reverse osmosis, and this also translates to ancillary benefits for downstream treatment process performance, including ozonation. Consistent with prior literature focusing on conventional drinking water applications, C/F treatment of secondary wastewater effluent also achieves significant removal of microorganisms. These removals are also correlated with rapid microbiological surrogates, which is beneficial for regulatory LRV crediting.
Coagulation with ferric chloride consistently reduced total ATP and intact cell counts with increasing ferric doses (10–50 mg Fe per L) across three wastewaters. LRVs of up to 3 for MS2 bacteriophage and B. subtilis spores were demonstrated for the same range of ferric doses, and their removals were highly correlated to tATP, total and intact cell counts (TCC and ICC) via flow cytometry, and DOC removal, even during off-specification coagulation conditions. UVA254 removal was correlated to microbial removal during nominal coagulation conditions and had the added benefit of being able to detect off-specification coagulation conditions. Additionally, treatment with enhanced coagulation improved downstream ozone performance based on ozone Ct and microbial inactivation, although this greater ozone stability resulted in slightly reduced hydroxyl radical exposure. Specific ozone doses between 0.8 and 1.0 achieved LRVs >5 for MS2 with and without coagulation, while LRVs for B. subtilis spores increased from ≤0.5 LRV without coagulation to ∼2.0 with coagulation. This was driven by the significant increase in ozone Ct for similar specific ozone doses. Thus, C/F treatment, particularly when coupled with downstream granular media filtration, could warrant LRV credits similar to those awarded to reverse osmosis in potable reuse applications, and consistent with those awarded in conventional drinking water treatment systems. This would be highly valuable for inland communities seeking to implement CBAT and could potentially broaden adoption of potable reuse.
Data availability
Most raw data are provided in the ESI.† Additional data are available upon request.
Author contributions
CMM, AJA, DG, ECW contributed to the design and implementation/conducting of the research. CMM conducted microbial analyses, analyzed data, and wrote the manuscript with input from authors. DG, AJA, ECW extensively revised the manuscript. DG and ECW secured funding. ECW conceptualized and managed the project.
Conflicts of interest
The authors do not declare conflict of interest.
Acknowledgements
This project was funded as a part of Water Research Foundation Project # 5035. The project team would like to acknowledge Janie Holaday, Rebecca Trenholm, Oscar Quiñones, and Brett Vanderford at SNWA for their support with non-microbial water quality analyses; Gabriella Portillo-Chamul at SNWA for assisting in conducting coagulation and batch ozone tests; and Samantha Hogard (formerly Hampton Roads Sanitation District), Aleks Pisarenko at Trussell Technologies, and Mack Pearce and Hampton Roads Sanitation District for assisting in sample collection and sharing information on wastewater treatment at the different sampling locations.
References
- M. Bourgin, B. Beck, M. Boehler, E. Borowska, J. Fleiner and E. Salhi,
et al., Evaluation of a full-scale wastewater treatment plant upgraded with ozonation and biological post-treatments: Abatement of micropollutants, formation of transformation products and oxidation by-products, Water Res., 2018, 129, 486–498 CrossRef CAS PubMed.
- D. Gerrity, K. Crank, E. Steinle-Darling and B. M. Pecson, Establishing pathogen log reduction value targets for direct potable reuse in the United States, AWWA Water Sci., 2023, 5(5), 1–16 Search PubMed.
-
California Division of Drinking Water, DPR Framework 2nd edition Addendum – Early Draft of Anticipated Criteria for Direct Potable Reuse, 2021, pp. 1–40 Search PubMed.
-
USEPA, Long Term 2 Enhanced Surface Water treatment Rule, United States Environ Prot Agency, 2006, pp. 1–375, Available from: http://www.gpo.gov/fdsys/pkg/FR-2006-01-05/pdf/06-4.pdf Search PubMed.
-
USEPA, Interim Enhanced Surface Water Treatment Rule, Federal Register, 1998 Search PubMed.
- J. Heffron and B. K. Mayer, Emerging investigators series: Virus mitigation by coagulation: Recent discoveries and future directions, Environ. Sci.: Water Res. Technol., 2016, 2(3), 443–459 RSC.
- V. Chalapati Rao, J. M. Symons, A. Ling, P. Wang, T. G. Metcalf and J. C. Hoff,
et al., Removal of Hepatitis a Virus and Rotavirus By Drinking Water Treatment, J. - Am. Water Works Assoc., 1988, 80(2), 59–67 CrossRef.
- A. H. Havelaar, M. van Olphen and J. F. Schijven, Removal and Inactivation of Viruses by Drinking Water Treatment Processes Under Full Scale Conditiond, Water Sci. Technol., 1995, 31(5–6), 55–62 CrossRef CAS.
- A. Nasser, D. Weinberg, N. Dinoor, B. Fattal and A. Adin, Removal of hepatitis A virus (HAV), poliovirus and MS2 coliphage by coagulation and high rate filtration, Water Sci. Technol., 1995, 31(5–6), 63–68 CrossRef CAS.
- N. R. Dugan, K. R. Fox, J. H. Owens and R. J. Miltner, Controlling Cryptosporidium Oocysts Using Conventional Treatment, J. - Am. Water Works Assoc., 2001, 93(12), 64–76 CrossRef CAS.
- J. D. Plummer, J. K. Edzwald and M. B. Kelley, Removing Cryptosporidium by dissolved-air flotation, J. AWWA, 1995, 87(9), 85–95 CrossRef CAS.
- S. States, K. Stadterman, L. Ammon, P. Vogel, J. Baldizar and D. Wright,
et al., Protozoa in river water: Sources, occurrence, and treatment, J. - Am. Water Works Assoc., 1997, 89(9), 74–83 CrossRef CAS.
-
USEPA, Stage 1 Disinfectants and Disinfection Byproduct Rule, 1998 Search PubMed.
- E. C. Wert, S. Gonzales, M. M. Dong and F. L. Rosario-Ortiz, Evaluation of enhanced coagulation pretreatment to improve ozone oxidation efficiency in wastewater, Water Res., 2011, 45(16), 5191–5199, DOI:10.1016/j.watres.2011.07.021.
- M. S. Elovitz, U. von Gunten and H. P. Kaiser, Hydroxyl radical/ozone ratios during ozonation processes. II. The effect of temperature, pH, alkalinity, and DOM properties, Ozone: Sci. Eng., 2000, 22(2), 123–150 CrossRef CAS.
- X. Yang, C. Shang, W. Lee, P. Westerhoff and C. Fan, Correlations between organic matter properties and DBP formation during chloramination, Water Res., 2008, 42(8–9), 2329–2339 CrossRef CAS PubMed.
- N. Rockey, H. N. Bischel, T. Kohn, B. Pecson and K. R. Wigginton, The utility of flow cytometry for potable reuse, Curr. Opin. Biotechnol., 2019, 57, 42–49, DOI:10.1016/j.copbio.2018.12.009.
- H. R. Safford and H. N. Bischel, Flow cytometry applications in water treatment, distribution, and reuse: A review, Water Res., 2019, 151, 110–133, DOI:10.1016/j.watres.2018.12.016.
- M. D. Besmer and F. Hammes, Short-term microbial dynamics in a drinking water plant treating groundwater with occasional high microbial loads, Water Res., 2016, 107, 11–18, DOI:10.1016/j.watres.2016.10.041.
- M. D. Besmer, J. Epting, R. M. Page, J. A. Sigrist, P. Huggenberger and F. Hammes, Online flow cytometry reveals microbial dynamics influenced by concurrent natural and operational events in groundwater used for drinking water treatment, Sci. Rep., 2016, 6, 1–10 CrossRef PubMed.
- F. Hammes, T. Broger, H. U. Weilenmann, M. Vital, J. Helbing and U. Bosshart,
et al., Development and laboratory-scale testing of a fully automated online flow cytometer for drinking water analysis, Cytometry, Part A, 2012, 81(6), 508–516 CrossRef PubMed.
- M. Vital, M. Dignum, A. Magic-Knezev, P. Ross, L. Rietveld and F. Hammes, Flow cytometry and adenosine tri-phosphate analysis: Alternative possibilities to evaluate major bacteriological changes in drinking water treatment and distribution systems, Water Res., 2012, 46(15), 4665–4676 CrossRef CAS PubMed.
- Y. Lee, S. Imminger, N. Czekalski, U. von Gunten and F. Hammes, Inactivation efficiency of Escherichia coli and autochthonous bacteria during ozonation of municipal wastewater effluents quantified with flow cytometry and adenosine tri-phosphate analyses, Water Res., 2016, 101, 617–627, DOI:10.1016/j.watres.2016.05.089.
- B. Michen and T. Graule, Isoelectric points of viruses, J. Appl. Microbiol., 2010, 109(2), 388–397 CrossRef CAS PubMed.
- J. F. Schijven and S. M. Hassanizadeh, Removal of viruses by soil passage: Overview of modeling, processes, and parameters, Crit. Rev. Environ. Sci. Technol., 2000, 30(1), 49–127 CrossRef CAS.
- C. P. White, J. Popovici, D. A. Lytle, N. J. Adcock and E. W. Rice, Effect of pH on the electrophoretic mobility of spores of Bacillus anthracis and its surrogates in Aqueous Solutions, Appl. Environ. Microbiol., 2012, 78(23), 8470–8473 CrossRef CAS PubMed.
- K. M. Wiencek, N. A. Klapes and P. M. Foegeding, Hydrophobicity of Bacillus and Clostridium spores, Appl. Environ. Microbiol., 1990, 56(9), 2600–2605 CrossRef CAS PubMed.
-
M. H. Adams, Bacteriophages, Interscience Publishers, INC., New York, 1959, vol. 4, pp. 122–129 Search PubMed.
- K. L. Rakness, I. Najm, M. Elovitz, D. Rexing and S. Via, Cryptosporidium log-inactivation with ozone using effluent CT10, geometric mean CT10, extended integrated CT10 and extended CSTR calculations, Ozone: Sci. Eng., 2005, 27(5), 335–350 CrossRef CAS.
- U. von Gunten, Ozonation of Drinking Water: Part I. Oxidation Kinetics and Product Formation, Water Res., 2003, 37, 1443–1467 CrossRef CAS PubMed.
- U. von Gunten, A. Bruchet and E. Costentin, Bromate formation in advanced oxidation processes, J. - Am. Water Works Assoc., 1996, 88(6), 53–65 CrossRef CAS.
-
E. Gatza, F. Hammes and E. Prest, Assessing Water Quality with the BD AccuriTM C6 Flow Cytometer: White Paper. BD Biosciences, 2013, Available from: http://classtap.pbworks.com/f/SkillSoft+-+Blended+Elearning.pdf.
- B. J. Vanderford, F. L. Rosario-Ortiz and S. A. Snyder, Analysis of p-chlorobenzoic acid in water by liquid chromatography-tandem mass spectrometry, J. Chromatogr. A, 2007, 1164(1–2), 219–223 CrossRef CAS PubMed.
-
USEPA, Enhanced Coagulation and Enhanced Precipitate Softening Guidance Manual. EPA 814-R-99-012, United States Environmental Protection Agency, 1999.
- J. Duan and J. Gregory, Coagulation by hydrolysing metal salts, Adv. Colloid Interface Sci., 2003, 100–102(SUPPL), 475–502 CrossRef CAS.
- M. Arnold, J. Batista, E. Dickenson and D. Gerrity, Use of ozone-biofiltration for bulk organic removal and disinfection byproduct mitigation in potable reuse applications, Chemosphere, 2018, 202, 228–237, DOI:10.1016/j.chemosphere.2018.03.085.
- R. C. Turner and K. E. Miles, The Ultraviolet Absorption Spectra of the Ferric Ion and Its First Hydrolysis Product in Aqueous Solutions, Can. J. Chem., 1957, 35, 1002–1009 CrossRef CAS.
-
Texas Water Development Board, Direct potable reuse resource document, 2015, 1, 1248321508, pp. 1–178, Available from: http://www.twdb.texas.gov/publications/reports/contracted_reports/doc/1248321508_Vol1.pdf Search PubMed.
- C. P. Gerba and W. Q. Betancourt, Viral Aggregation: Impact on Virus Behavior in the Environment, Environ. Sci. Technol., 2017, 51(13), 7318–7325 CrossRef CAS PubMed.
- J. Langlet, F. Gaboriaud and C. Gantzer, Effects of pH on plaque forming unit counts and aggregation of MS2 bacteriophage, J. Appl. Microbiol., 2007, 103(5), 1632–1638 CrossRef CAS PubMed.
- Y. Y. Feng, S. L. Ong, J. Y. Hu, X. L. Tan and W. J. Ng, Effects of pH and temperature on the survival of coliphages MS2 and Qβ, J. Ind. Microbiol. Biotechnol., 2003, 30(9), 549–552 CrossRef CAS PubMed.
- K. Amoah, X. H. Dong, B. P. Tan, S. Zhang, F. K. A. Kuebutornye and S. Y. Chi,
et al., In vitro Assessment of the Safety and Potential Probiotic Characteristics of Three Bacillus Strains Isolated From the Intestine of Hybrid Grouper (Epinephelus fuscoguttatus♀ × Epinephelus lanceolatus♂), Front. Vet. Sci., 2021, 8, 1–16 Search PubMed.
- S. Ceuppens, M. Uyttendaele, K. Drieskens, A. Rajkovic, N. Boon and T. Van De Wiele, Survival of bacillus cereus vegetative cells and spores during in vitro simulation of gastric passage, J. Food Prot., 2012, 75(4), 690–694, DOI:10.4315/0362-028X.JFP-11-481.
- T. Clavel, F. Carlin, D. Lairon, C. Nguyen-The and P. Schmitt, Survival of Bacillus cereus spores and vegetative cells in acid media simulating human stomach, J. Appl. Microbiol., 2004, 97(1), 214–219 CrossRef CAS PubMed.
- M. Abbaszadegan, B. K. Mayer, H. Ryu and N. Nwachuku, Efficacy of removal of CCL viruses under enhanced coagulation conditions, Environ. Sci. Technol., 2007, 41(3), 971–977 CrossRef CAS PubMed.
- B. K. Mayer, H. Ryu and M. Abbaszadegan, Treatability of U.S. Environmental Protection Agency contaminant candidate list viruses: Removal of coxsackievirus and echovirus using enhanced coagulation, Environ. Sci. Technol., 2008, 42(18), 6890–6896 CrossRef CAS PubMed.
- E. W. Rice, K. R. Fox, R. J. Miltner, D. A. Lytle and C. H. Johnson, Evaluating plant performance with endospores, J. - Am. Water Works Assoc., 1996, 88(9), 122–130 CrossRef CAS.
- M. Pritchard, T. Craven, T. Mkandawire, A. S. Edmondson and J. G. O'Neill, A comparison between Moringa oleifera and chemical coagulants in the purification of drinking water - An alternative sustainable solution for developing countries, Physics and Chemistry of the Earth, Parts A/B/C, 2010, 35(13–14), 798–805, DOI:10.1016/j.pce.2010.07.014.
- T. Meyn, T. O. Leiknes and A. Konig, MS2 Removal from High NOM Content Surface Water by Coagulation - Ceramic Microfiltration, for Potable Water Production, AIChE J., 2011, 58(7), 2270–2281 CrossRef.
- B. Zhu, D. A. Clifford and S. Chellam, Virus removal by iron coagulation-microfiltration, Water Res., 2005, 39(20), 5153–5161 CrossRef CAS PubMed.
- H. T. Richard and J. W. Foster, Acid Resistance in Escherichia coli, Adv. Appl. Microbiol., 2003, 52, 167–184 CAS.
- J. Heffron, M. Samsami, S. Juedemann, J. Lavin, S. Tavakoli Nick and B. A. Kieke,
et al., Mitigation of viruses of concern and bacteriophage surrogates via common unit processes for water reuse: A meta-analysis, Water Res., 2024, 252, 121242, DOI:10.1016/j.watres.2024.121242.
- B. Headd and S. A. Bradford, Use of aerobic spores as a surrogate for cryptosporidium oocysts in drinking water supplies, Water Res., 2016, 90, 185–202, DOI:10.1016/j.watres.2015.12.024.
- S. Gamage, D. Gerrity, A. N. Pisarenko, E. C. Wert and S. A. Snyder, Evaluation of Process Control Alternatives for the Inactivation of Escherichia coli, MS2 Bacteriophage, and Bacillus subtilis Spores during Wastewater Ozonation, Ozone: Sci. Eng., 2013, 35(6), 501–513 CrossRef CAS.
- Y. Lee, D. Gerrity, M. Lee, A. E. Bogeat, E. Salhi and S. Gamage,
et al., Prediction of micropollutant elimination during ozonation of municipal wastewater effluents: Use of kinetic and water specific information, Environ. Sci. Technol., 2013, 47(11), 5872–5881 CrossRef CAS PubMed.
- C. M. Morrison, S. Hogard, R. Pearce, A. Mohan, A. N. Pisarenko and E. R. V. Dickenson,
et al., Critical Review on Bromate Formation during Ozonation and Control Options for Its Minimization, Environ. Sci. Technol., 2023, 57(47), 18393–18409 CrossRef CAS PubMed.
- E. C. Wert, F. L. Rosario-ortiz, D. D. Drury and S. A. Snyder, Formation of oxidation byproducts from ozonation of wastewater, Water Res., 2007, 41, 1481–1490 CrossRef CAS PubMed.
- J. Reungoat, B. I. Escher, M. Macova, F. X. Argaud, W. Gernjak and J. Keller, Ozonation and biological activated carbon filtration of wastewater treatment plant effluents, Water Res., 2012, 46(3), 863–872 CrossRef CAS PubMed.
- R. A. Tackaert, A. N. Pisarenko, E. C. Chen, A. Kolakovsky, B. M. Pecson and J. E. Drewes,
et al., Demonstrating process robustness of potable reuse trains during challenge testing with elevated levels of acetone, formaldehyde, NDMA, and 1,4-dioxane, J. Water Supply: Res. Technol.--AQUA, 2019, 68(5), 313–324 CrossRef.
|
This journal is © The Royal Society of Chemistry 2024 |
Click here to see how this site uses Cookies. View our privacy policy here.