Spiers Memorial Lecture: New horizons in nanoelectrochemistry
Received
1st October 2024
, Accepted 9th October 2024
First published on 15th October 2024
Abstract
This introductory lecture prefaces the 2024 New Horizons in Nanoelectrochemistry Faraday Discussion. A broad view of the previous Discussions related to nanoelectrochemistry is taken. Big ideas or concepts discussed at these previous meetings are identified, along with specific examples in each area. Closing comments aimed at a high level and related to where we are today and what is needed to continue to drive nanoelectrochemistry towards the horizon are considered.
Introduction
Nanoelectrochemistry, defined broadly as an electrochemical process or measurement where the critical dimension is nanometers in scale, has become an increasingly important topic in modern science and technology. This meeting represents the fourth in a series of Faraday Discussions to revolve around the idea or concept of electrochemistry at the nanoscale. Previous Faraday Discussions in this series – Single Entity Electrochemistry (2016), Electrochemistry at Nano-interfaces (2018), and Next Generation Nanoelectrochemistry (2022) – have been thought-provoking events which helped to define challenges and paradigm shifts in electrochemistry at the nanoscale. Concepts such as single entity measurements, correlative/multimodal imaging, and confinement effects have been introduced or refined at these meetings and have subsequently gained life in the community writ large. The introductory and concluding lectures for these meetings effectively present the evolution of the state-of-the-art in nanoscale electroanalytical and physical electrochemistry over the last decade.1–6 We now have a significant body of work from previous Discussions on the topic of nanoelectrochemistry. When this is coupled with the avalanche of opinion pieces (a small selection7–18) that are produced in this area, rediscussing or rehashing previous points seems a fool's errand. Instead in this introduction, we will try to identify some of the big ideas from previous meetings and the community, and provide a few key examples recently reported. With this backdrop in mind, we will attempt to set the stage for the New Horizons in Nanoelectrochemistry Faraday Discussion (2024) with consideration of the challenges we face today.
Previous Discussions mentioned above have proven to be cauldrons for seeding, refining and developing key concepts in the area of nanoelectrochemistry. This is one of the most important outcomes of the Discussions – shaping our view going forward. If we review the last three meetings, there is some repetition in the topics, and repetition again in what will be discussed here over the next few days. This is to be expected, as chronologically, we are looking at a span of eight years, which is a short segment of the grand arc of science. The scope of these meetings is both focused on the nanoscale and yet also diffuse in terms of area of enquiry. Further, we are tackling big challenges. One area in which I have personally worked – nanopore sensing – displays a dramatic historical arc that is part of the nanoelectrochemical story. Nanopore sensing, specifically sequencing nucleic acids, Fig. 1,19 was a lofty dream when I was a postdoctoral associate working in the laboratory of Charles Martin at the University of Florida, circa 2003. The project I was working on was led by Chuck, Hagan Bayley, Henry White and Paul Cremer as PIs and was tasked with sequencing by nanopore. Meetings of a larger group of ∼30 PIs working intensely in this area were exciting, high-pressure, and fast-paced. I left my postdoc in 2006 unsure if any real progress had been made towards sequencing and skeptical about the future. Mind you, this was after work in the late 1980s and early 1990s described the concept of sequencing by nanopore. The temporal length of this particular arc is even longer when one considers that the true foundation for nanopore sequencing resides in the Coulter counter, patented in 1949.20 Today, in 2024, almost 40 years after conceptualizing nanopore sequencing and ∼75 years after the invention of the Coulter counter, sequencing nucleic acids is a reality.21 Here today in this meeting, we will be reminded that 75 years is not the extent of the arc. We will see work that charts new paths, expanding into protein (peptide) sequencing by nanopore. With the arc of nanopore sequencing as an example, where 75 years has seen dramatic progress from a relatively simple foundation to functional real-world devices, many aspects we consider to be nanoelectrochemistry are still early in development, and the eight-year window defined by this set of Discussions is relatively short. Much of what we have and will discuss leans heavily toward the fundamental side of the development, and we might expect arcs to be taken up by other areas of science as we develop the foundations of new areas.
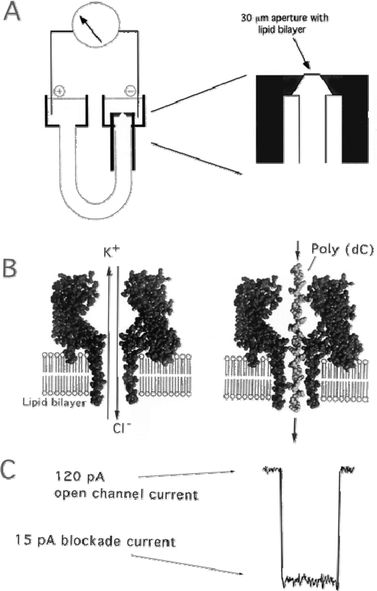 |
| Fig. 1 (A) Nanopore support device, in which a U-tube supports a lipid bilayer membrane bathed in 1.0 M KCl. Hemolysin subunits are added to the cis chamber facing the bilayer, and a voltage is applied (120 mV) positive on the trans side. When a single pore inserts into the bilayer, a characteristic current of ∼120 pA immediately appears. At that point the chamber is flushed so that no further pores can insert. An amplifier with picoampere sensitivity monitors current modulations and sends analog signals to an A/D converter which are stored in a computer for later processing. (B) The hemolysin nanopore is shown in cross section, based on the X-ray data of Song. An ionic current of KCl is driven by the applied voltage through the open pore on the left. Under these conditions, ionic polymers such as nucleic acids are captured by the standing electrical field and driven through the pore. A synthetic poly(dC) DNA strand traversing the pore is shown on the right. (C) When a single-stranded nucleic acid molecule traverses the pore, a transient blockade of ionic current results, during which the ionic current is reduced from 120 to 15 pA.19 | |
Horizons
The title of this meeting is New Horizons in Nanoelectrochemistry, which is at once both inspirational and daunting, as the concept of a horizon itself is enigmatic. Writing in Scientific American, astronomer Phil Plait considered a literal horizon as follows – “I get squinty and teary trying to focus on it, which is a fool's errand because, in a sense, the horizon doesn't exist. It's as real as a rainbow in that you can see it, but no curious sojourner can ever arrive there; as you approach the horizon, it always recedes out of reach”.22 What we seek at this meeting (and in all of science) is a glimpse of what is on the horizon, what can we see, define, dream, and as we approach that horizon of nanoelectrochemistry, what clarity can be achieved with our squinting eyes. Another way to think about this is we can't now see the true long-term impact of what we discuss at this meeting, because the horizon and exact path we might take to reach the horizon is always just out of reach. But importantly, steps towards the elusive horizon can be brought into view, and we can discuss and bring those steps into focus, acknowledging in the end that many of the steps may inevitably be realized as missteps. But we want to define and begin to take these steps at this meeting, regardless. The example of nanopore sequencing also makes this point in another more concrete way. I imagine that Wallace H. Coulter (inventor of the Coulter counter) would have been welcome at any of these nanoelectrochemistry Faraday Discussions and would have been warmly welcomed to a seat at the nanoelectrochemistry table. But in 1949, he was counting cells and microparticles, which were extremely important, meaningful, and even commercially viable goals. However, if we defined the horizon in 1949 as today – this exact point in time – could Coulter have been able to squint to see sequencing DNA samples based on the concept of his inventions on the horizon (remember the double-helix of DNA wasn't discovered until the early 1950s)? To be sure, Wallace H. Coulter is legendary and his genius as an inventor is evident, so if anyone could have that prescience, he might be that person. If he had, one thing is for sure, the post-lecture discussion would have been beyond lively. The point I want to underscore here is that we need to define what are new ideas, what is important to think about, and what areas are likely to be most important as we march towards the horizon even if we remain unsure of what or where the horizon actually resides.
A final point to make in this introduction is that the temporal window of these meetings, 2016–2024, was pierced by a global pandemic, which has had meaningful consequences to continuity in progress as a community. The pandemic disrupted and rescheduled the 2020 (2022) meeting, although as evidenced by the present meeting, we seem to have recovered. Albeit we are not unchanged. We are different, more and less at the same time, a point I will return to later.
Big ideas and outcomes from Faraday Discussions on nanoelectrochemistry
A series of common themes or ideas have emerged from this set of Discussions, with acknowledgement that the Discussions might not always have been the explicit nucleation site for the idea but did prove important in shaping the overall idea. Further, the ideas don't always land squarely in nanoelectrochemistry, but bleed over into other areas at the forefront of science, much like the case of the Coulter counter. Similarly, at this point advances in instrumentation are a thread that runs through many of the big ideas, and that is often defined by where in development an area of instrumentation happens to be at present. If we look at scanning electrochemical cell microscopy (SECCM) as an example, at the 2016 meeting, SECCM was 5–6 years old at that point,23 and the instrument itself was the experiment. As we move through the subsequent two meetings and into this meeting, we see that there is a shift from instrument development to application, although instrument development in SECCM remains highly relevant. Previous introductions/summations have made this point very clear – instrument development and application in nanoelectrochemistry are comingled and often indistinguishable. We are working at the very front of the field and that means we are often required to develop a new way to measure what we are looking for on the nanoelectrochemistry horizon. Likewise, fabrication is comingled generally across the spectrum of experiments. Twenty years ago, making a nanoscale electrode was a challenge, but today, I would expect a majority of attendees at this meeting to have made a well-characterized nanoelectrode or could do so without heroic efforts. For these reasons, the critical areas of instrumentation and fabrication are ubiquitous in nanoelectrochemistry.
What I consider to be big ideas that have been discussed at these initial meetings are briefly summarized with select state-of-the-art examples below.
Single entity electrochemistry
The literal title of the first Faraday Discussion that could be considered on nanoelectrochemistry, single entity electrochemistry (SEE), involves the study of one “thing” at a time by electrochemical methods. This is not explicitly nanoscale in nature; however, the electrochemical toolbox is highly applicable to nanoelectrochemistry. When SEE was first defined, true nanoscale electrochemical imaging was still relatively new, which has since grown and provided a means to collect meaningful single entity electrochemical data. Nanoimpact experiments were a hot topic at the initial meeting, and continue, although the number of papers on this topic has abated. SEE remains an area of active enquiry, but also has many tendrils that feed into other aspects of nanoelectrochemistry.
An excellent recent example of SEE in the realm of nanoelectrochemistry is found in the work of Schuhmann and coworkers where nanoparticle catalysts for the nitrate reduction reaction (NO3RR) were carefully analyzed.24 In this work, Cu2O and Co3O4 catalysts were first studied individually at the single-entity level, and then at an electrode where only two particles were present at the electrode, one Cu2O nanocube catalyst and one Co3O4 nanocube catalyst. A relay catalyst was formed based on the interaction of these two single-entity catalysts, and the effects of the relay were investigated at each individual particle. In an especially impressive technical display, the relative position of the two individual nanoparticles was controllably placed with micromanipulators (Fig. 2a) in three different samples. One where the particles were next to each other, a second where there was partial overlap and a third where particles did not touch each other at all. In Fig. 2b–d electron micrographs are shown. Relative reduction currents for NO3RR are plotted over 5 cycles in Fig. 2e. Energy dispersive X-ray (EDX) mapping is shown in Fig. 2f–h before (top) and after (bottom) electrochemical cycling, where Co is purple and Cu is magenta. This elegant experiment was able to clarify the fate of Cu. NO3RR in the presence of both particles was assumed to undergo a two-step tandem process, where NO3− was reduced to NO2− at the Cu2O particle, followed by NO2− reduction to NH3 at the Co3O4 particle. Single particle experiments as operated here allowed spatially defined levels of interparticle interactions and accurate calculation of current densities based on precise geometric knowledge of each particle. As observed in Fig. 2f–h, after five electrochemical cycles, for the particle that is isolated (Fig. 2h), the Cu is leached by NO2 formed during reduction of NO3−. The Co3O4 particle close proximity (Fig. 2f and h) allows subsequent reduction to NH3, thereby lowering corrosion of Cu. This experiment is a tour de force that might not have been imagined at the first Discussion on SEE. The strides in this area have been tremendous. The once unimaginable has become routine in many cases.
 |
| Fig. 2 (a) Schematic diagram of the fabrication process of two particles nanoelectrode assemblies. (b)–(d) TEM, STEM images, and corresponding EDX line scans of Cu2O + Co3O4_CNE-1 (b), Cu2O + Co3O4_CNE-2 (c), Cu2O + Co3O4_CNE-3 (d). (e) Plots showing the change in the ratio of the reduction current of Cu2O, Co3O4, Cu2O + Co3O4 nanoelectrode assemblies at −0.35 V (vs. RHE) compared to the 1st CV. (f)–(h) EDX mapping of Cu2O + Co3O4_CNE-1 (f), Cu2O + Co3O4_CNE-2 (g), Cu2O + Co3O4_CNE-3 (h) before (top) and after 5 CV cycles (bottom).24 | |
Correlative/multimodal measurements
In the closing paper of the first meeting, Crooks stated – “Many of the systems described in this Discussion volume were characterized only by electrochemical methods, and this inevitably results in some mechanistic guesswork. Coupling spectroscopy or microscopy with electrochemistry in operando can address this issue, but that is often difficult or impossible to do. New analytical methods are needed for coupling to electrochemistry”.2 This point was well taken and is being addressed by the community in a variety of forms. Identical location microscopy is the straightforward example of this where a microscopy technique, for example scanning electron microscopy, is used to interrogate exactly the same place on a sample before and after an electrochemical process, such as electrodissolution, electrodeposition, or electrocatalysis. Measurements that take this a step further are correlative/multimodal measurements, where multiple microscopy techniques (multimodal) are applied to identical locations (correlative) on a sample. In some instances, measurements can even be collected concurrently, preserving temporal information relevant to the electrochemical process of interest.
Fig. 3 shows an example of correlative SECCM and atomic force microscopy (AFM) to investigate the structure–function relationship governing proton transport through two-dimensional (2D) crystals reported by Unwin.25 The devices under study comprised graphene and hexagonal boron nitride (hBN) membranes which are suspended over SiNx apertures back-contacted with Nafion® and Pt substrate. An HCl-filled SECCM probe serves as proton reservoir and localized proton transport through the 2D membrane is detected at the Pt electrode. The SECCM maps (Fig. 3a and b) reveal spatially inhomogeneous proton currents across the monolayer graphene, with notable regions of high proton conductivity. The corresponding AFM adhesion force maps (Fig. 3c and d) indicate that these high-conductivity areas correlate with nanoscale wrinkles, edges of the SiNx aperture, and less prominent nano-ripples, where tensile strain accumulates. Similarly, on the hBN membranes (Fig. 3e and f), a significant proton transport current is observed throughout the monolayer, with further enhancement at wrinkled and strained regions, reinforcing the role of strain accumulation in facilitating proton permeation. Density functional theory calculations (Fig. 3g and h) support these experimental findings, suggesting that strain and curvature lower the energy barriers for proton transport by altering the electron-density distribution within the crystal lattice. This study highlights the effectiveness of correlative multi-microscopy to help demystify intricate structure–function relationships at the nanoscale, crucial for understanding and modulating the properties of complex electromaterials.
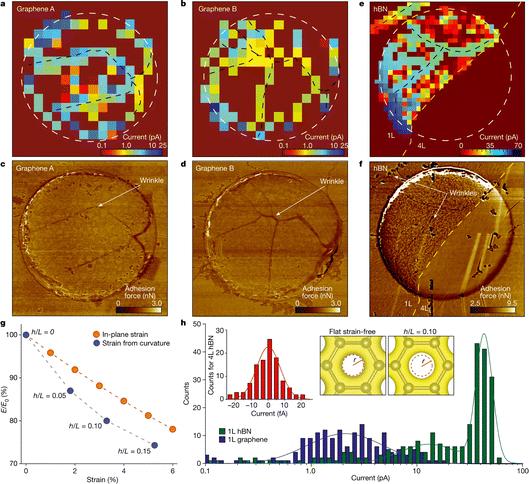 |
| Fig. 3 (a) and (b) SECCM maps for two graphene devices. The white dashed circles mark the rim of the 2 μm-diameter apertures in SiNx. (c) and (d) AFM force maps for the devices in the panels above. Wrinkles and edges are clearly visible in the AFM maps and correlate with high-conductivity areas in the SECCM maps. For easier comparison, the black dashed curves in (a) and (b) mark wrinkles' positions. (e) Proton currents through an hBN device. Yellow dashed curve, border between monolayer (1L; left) and tetralayer (4L; right) hBN. (f) AFM force map for the device in (e). Apparent wrinkles are indicated by the arrows and marked by the black dashed curves in (e). A particular feature of this device is notable proton currents in the top left corner in (e), away from the aperture in SiNx. (g) Strain lowers the energy barrier E for proton permeation (E0 is the barrier for unstrained graphene). Blue symbols, the effect of strain arising from curvature; values of h/L are specified next to each point. Red data, E/E0 due to purely in-plane strain. (h) Statistics of proton currents for graphene and hBN monolayers (data from a, b and e). Left inset, statistics collected from the tetralayer region. Solid curves, best Gaussian and double-Gaussian fits for graphene and monolayer hBN, respectively (accuracy of about 10% in determining the modes of the normal distributions). The right two-panel inset shows the calculated electron density provided by the crystal lattice for unstrained (left) and strained (right) graphene; the latter calculations are for strain arising from curvature with h/L = 0.10. To make changes in the electron density evident, the dashed red circle in the left panel marks the boundary between regions with densities above and below 0.2 e Å−3 (the latter region is shown in white). The same circle is projected onto the right panel and emphasizes that the low-density region expanded in the strained lattice.25 | |
Confinement effects
Depending on electrolyte concentration, the electrochemical double layer provides a sort of nanoconfinement in the direction orthogonal to an electrode surface. In recent years, there has been rediscovery of these effects, for instance studies of electrocatalysis have realized that the interface of the electrode – where the action is at usually – behaves differently than the rest of the solution. As such, ligands and co/counter ions can have significant chemical or electrostatic effects in the double layer, and subsequently on catalytic properties. These interactions seem obvious at some level but are not appreciated or taken into full account in all electrochemical applications. Essentially the double layer requires that every electrochemistry experiment should think about confinement effects! Nanoelectrochemistry confinement effects are often taken to include an additional dimensionality beyond the double layer. For example, the droplet at the tip of an SECCM cell, the critical volume of a nanopore sensor, the space between two nanogap electrodes, the tip of a solution filled nanopipette, all present unique three-dimensional configurations where the distribution of ions, electric fields, and concentrations can differ dramatically from even the two-dimensional double layer effects, with significant effects realized in nanoelectrochemical function.
Pan et al. recently described a functionalized nanopipette that could operate as an electrochemical trap, affording the chance to study enzymes at low concentrations from a single cell.26 To realize the electrochemical trap, the inner tip and outer wall of the nanopipette were coated with Pt. Initially, the potential in the system was controlled such that the potential between the electrode inside the pipette was controlled relative to the electrode in the bath solution. In the example in Fig. 4, the pipette was lowered into a solution of liposomes that contained glucose oxidase. Application of −0.5 V resulted in translocation of liposomes into the tip of the pipette, as monitored by resistive pulses in the current–time profile (not shown). After loading the tip, the pipette was withdrawn from solution and a potential of 10 V was applied inside of the pipette, to drive electroosmotic flow toward the tip of the pipette, effectively trapping enzymes in the tip region near the Pt electrode. After waiting a set amount of time, the applied voltage was switched from the electrode inside the pipette to a value of 0.6 V at the Pt layer at the tip, which served to oxidize hydrogen peroxide produced from turnover of glucose by glucose oxidase. This clever control of electric fields and applied potentials realizes electrochemical confinement to effectively detect low concentrations of enzymes from a solution or even the cytosol of a cell. The opportunity to confine reactants and reagents at the near-electrode region is a tool we are making good use of in nanoelectrochemistry.
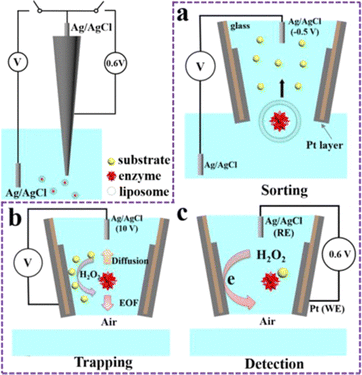 |
| Fig. 4 Schematic setup for the electrochemical molecule trap and the following detection of enzymatic activity. (a) Sorting process. (b) Trapping process. (c) Detection process. WE stands for the working electrode and RE stands for the reference electrode. The continuous Pt coating layer is present both inside the tip of the nanopipette as the electrochemical detector and on the whole outer wall of the nanopipette for connection with a copper wire.26 | |
Imaging
Electrochemical imaging has advanced dramatically. Initially, in the late 1980s/early 1990s, electrochemical imaging was focused almost exclusively on scanned probe microscopies (SPMs), especially scanning tunneling microscopy (STM), AFM and scanning electrochemical microscopy (SECM). STM presents the highest resolution (sub-angstrom) technique and both STM and AFM found new applications in tip-enhanced electrochemistry where Raman or surface-enhanced Raman signals can be collected to measure molecular species at surfaces under potential control.
For several decades true nanoscale electrochemical imaging with SECM proved challenging, primarily due to hardware limitations. Recent advances have allowed select labs to routinely realize nanoscale SECM. Scanning ion conductance microscopy (SICM) and SECCM have more recently become significant alternatives for nanoelectrochemistry (especially SECCM). One particularly appealing draw of SICM and SECCM is the simplicity in fabricating nanoscale probes. Almost any lab can routinely generate nanoscale pipettes that enable access to nanoelectrochemistry with both techniques. This has been coupled with the adoption of electronics and hardware advances. In addition to SPMs, optical techniques, including spectroelectrochemistry, have gained popularity. Experiments involving electrogenerated chemiluminescence have long realized possible advantages of incorporating optical microscopy with electrochemistry. In recent years, and as will be discussed at the meeting more, advanced approaches to optoelectrochemistry are especially appealing. The appeal resides in the fact that optical measurements are fast relative to SPMs and the spatial resolution can be quite high, especially with super-resolution techniques.
A very recent report from Gooding and coworkers describes a highly intriguing approach to electrochemical super-resolution microscopy (Fig. 5).27 In this work, microtubules present in a cell sample were stained with Alexa647. Application of an alternating potential to the sample was shown capable of modulating the fluorescence intensity of the dye, presumably through reduction of the dye in the presence of thiol species, followed by oxidation back to the starting material. The process was highly repeatable, creating a switchable fluorescent signal suitable for stochastic optical reconstruction microscopy (STORM) in a format relabeled EC-STORM. The advantages of this technique are numerous and include the ability to operate without a UV laser to generate dye switching, and possibly more subtle control over density of fluorophores, a problem that often occurs if labeling is too efficient. This approach promises sub-diffraction-limited imaging through electrochemical control.
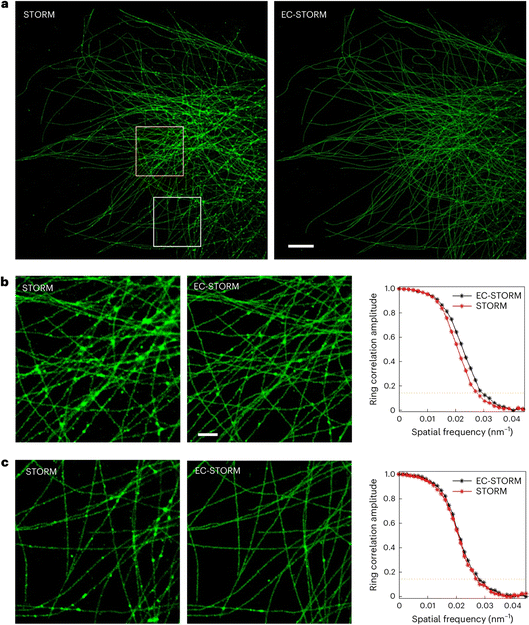 |
| Fig. 5 (a) Comparison of STORM and EC-STORM images was conducted for the same region of a cell. The ‘STORM’ condition refers to a 2 kW cm−2 of 642 nm laser and no UV laser was applied; the ‘EC-STORM’ condition refers to a 2 kW cm−2 of 642 nm laser and applying a negative potential from −0.6 to −0.4 V during data acquisition. Scale bar, 5 μm. (b) and (c) Zoomed-in regions highlighted by applying a negative potential; fewer blurred regions at intersecting microtubules were observed in the EC-STORM image. A zoomed-in view of the area highlighted by the yellow square in part a (b), and a zoomed-in view of the white square in part a (c). Scale bar, 1 μm. Fourier ring correlation curves corresponding to the zoomed-in regions on the left. The imaged COS-7 cells were labelled for microtubules with Alexa 647. The STORM and EC-STORM images were reconstructed from the same number of localization events under each condition.27 | |
Nanoelectrochemical imaging based on electron microscopy has also gained significant traction in recent years. Commercial electrochemical cells integrated with transmission electron microscopes have become much more widely available. Of note, especially in the case of electron-based imaging, special attention must be paid to the fundamental limits of the electrochemical system being studied, especially with respect to current densities, electric fields and electrochemical cell design. Ultimately, electrochemical imaging might find the most important applications in correlative/multimodal techniques described previously.
Big data and high-throughput electrochemistry
Generating large data sets has become a focal point for many analytical applications. Electrochemistry has lagged in terms of true high throughput experimentation, possibly because of the complexity of integrating a high density of electrodes or of creating many independent electrochemical cells. SECCM provides an interesting route to address high throughput measurements as each time the tip engages the surface, a unique electrochemical cell and experiment can be created, in effect allowing for serial high throughput nanoelectrochemistry. Nanoelectrochemical measurements are also advantageous for high throughput measurements, as small electrodes provide access to faster timescales which is important as the number of measurements in a large dataset increases. Big data also can take advantage of advances in statistical treatments (artificial intelligence, machine learning), but electrochemical data can sometimes prove difficult to parameterize. Take for instance a mass spectrum relative to a cyclic voltammogram. The information density in the mass spectrum (MS) is nominally higher (especially if MS/MS approaches are used) and the signal-to-noise is typically higher in MS. Still, a number of innovative examples have shown how big data can be used to drive discovery in electrochemistry and nanoelectrochemistry.
As an example from recent studies from the groups of Wang and Liu,28Fig. 6 outlines the process of constructing a database for an artificial neural network (ANN) to understand and predict the electrocatalytic performance of materials based on input properties. This model processed several thousands of published experimental results, and used a set of material properties, including atomic mass, atomic number, period and group, ionization potential, electronegativity, number of d electrons, and atomic radius, as input features. These are represented through 50 one-hot encoded variables, creating a versatile input vector characterized by 114 dimensions. The material properties are thus organized into a structured format that helps the model learn from the data. To minimize overfitting, dropout layers, and regularization techniques are integrated into the ANN architecture. The model was tested on a separate set of data to check predictive performance metrics such as overpotential for hydrogen evolution and oxygen evolution reaction (HER/OER) at 10 mA cm−2. Additionally, the model employs a method called SHAP (SHapley Additive exPlanations) to explain how different features influence output predictions. The study achieved substantial data-driven insights for electrocatalyst design for HER/OER applications. This workflow could potentially be adapted for nano-electrochemistry to process the vast datasets from high-throughput and electrochemical imaging experiments, and facilitate reliable, comprehensible conclusions. Processes like this may also someday enable intelligent automated nanoelectrochemistry experimentation. How close we are to ChatGPT for nanoelectrochemistry remains an open question.
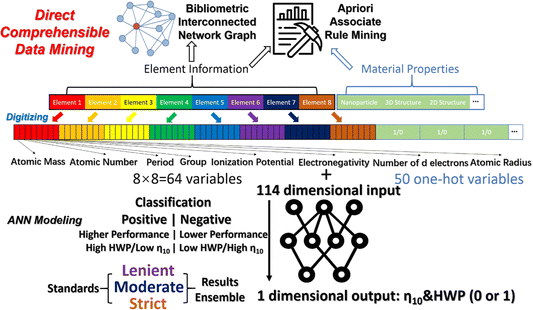 |
| Fig. 6 Illustration of the digitization process for constructing a database for ANN modeling.28 | |
Nanopore electrochemistry
Nanopore electrochemistry broadly covers synthetic and biological nanopores. Confinement effects that are manifested in both types of pores can lead to exceptionally high charge densities and/or electric fields that can then manifest unique nanoelectrochemical properties. Synthetic pores continue to evolve and find integration into ever complex fabrication protocols. Biological nanopores, such as the CsgG bacterial amyloid secretion channel, on which the Oxford Nanopore sequencing system referred to previously are based, continue to find interesting utility. Peptide sequencing by nanopore is one of the most exciting possible applications of biological nanopores.
Nanopores also find interesting applications as nanoscale reactors. In a recent example from Long's group,29 aerolysin nanopores were used to identify point mutations in amyloid peptides. The really impressive aspect of this work is the subtle signal analysis necessary to realize analytical utility – this aspect, careful data analysis and understanding of analytical figures of merit of the measurement are hallmarks of nanoelectrochemistry. Where typical nanopore measurements focus on dwell time or amplitude of current blockage, the approach taken here is altogether different. In fact, peptides with the same length and charge are differentiated, properties that would not be identified through standard analysis. Instead, fluctuations, or dynamics in the current blockades were analyzed carefully to differentiate analyte. A simple schematic of this paradigm is shown in Fig. 7. The aerolysin protein is shown with bilayer and electrode configuration set up for nanoelectrochemical sensing. The current–time trace shown in Fig. 7b represents two molecules that present signals difficult to resolve by normal descriptors. Instead, fluctuations in the signal at the base of the current pulses are fit to normal distributions to determine the fluctuation factor (σb), which is capable of differentiating molecules based on subtle molecule–pore interactions. This is a keen example of the promise of nanopore analysis of peptides, an area ripe for exploration beyond what can presently be achieved.
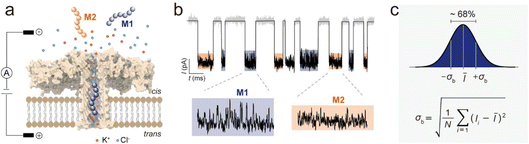 |
| Fig. 7 Single-molecule sensing with aerolysin nanopore based on the standard deviation of current blockade fluctuations (σb). (a) Single-channel recording of Molecule 1 (M1) and Molecule 2 (M2) using an aerolysin nanopore. M1 and M2 were forced to enter and translocate the nanopore from the cis solution by the voltages applied via a pair of Ag/AgCl electrodes. (b) The analytes produced signals with different current blockade characteristics, and the zoomed segments of two typical M1 and M2 signals show distinct current blockade fluctuations. (c) Calculation of σb for individual current blockade events. As the residual currents collected from every sampling point of a blockade signal are usually fitted into a normal distribution, σb was used to present the variations of the current blockade segment, where ∼68% of the recorded values are placed within one standard deviation from the mean residual current. The calculated equation for σb is shown at the bottom, where N, Ii and Ī represent the number of sampling points, the residual current at sampling point i, and the average value of the residual currents, respectively.29 | |
Theory and fundamentals
The 2018 introductory lecture by White focused on an important consideration for nanoelectrochemistry, the convolution of time and length scale,5 especially when the length scale becomes very short (nanoelectrochemistry). This type of fundamental consideration is critical if we are to make full use of nanoelectrochemical devices and open data to full analysis. In this same lecture, the stochastic nature of small populations and challenges in instrument design were highlighted. The closing remarks by Unwin in 2022, rightly recognized the gap we presently face between theory and models.1 Meaning continuum finite element models used ubiquitously now in nanoelectrochemistry don't fully incorporate fundamental theory that could be used to fit or predict nanoelectrochemical experiments. These gaps remain, yet there are clear examples of how fundamental theory can help expand our understanding of nanoelectrochemical systems.
For instance, Pendergast et al. developed a kinetic model to describe electric potential-driven proton transfer at acid/base self-assembled monolayer (SAM)-modified electrodes, as illustrated in Fig. 8.30 The model outlines the fundamental interactions that govern proton transfer, conceptualizing (de)protonation as a two-step process: first, the reversible transfer of protons at the SAM surface, followed by their transport through the diffuse electrical double layer to the bulk solution. With finite element simulations, the authors estimated rate constants for proton transport across various conditions of electric potential and supporting electrolyte concentrations. Their findings indicated that the rate-determining step in the overall process could shift between proton transfer and transport, influenced by factors such as the acidity of the functional groups and the applied electric potential. This model, therefore, offers a comprehensive framework to understand the influence of electric potential on acid/base chemistry at interfaces, as well as to guide the optimization of conditions for efficient proton or ion transfer reactions in electrochemical applications, and at length scales highly relevant to nanoelectrochemistry.
 |
| Fig. 8 Schematic representation of acid/base processes at an electrode modified with carboxylic acid-terminated alkanethiol SAMs. The electric potential distribution is represented by the red curve extending linearly from the metal (ϕM) to the plane of acid dissociation (ϕPAD) and subsequently decaying to the bulk solution potential (ϕS). Reversible protonation/deprotonation of the terminal carboxylic acid groups is shown by the equilibrium arrows labeled with H+ transfer. The equilibrium between a proton at the PAD and a proton in bulk solution is shown by the arrow labeled with H+ transport. Schematic is not drawn to scale.30 | |
The horizon – nanoelectrochemical bridges
The preceding discussion attempted to highlight the history of Faraday Discussions on nanoelectrochemistry, both highlighting areas of interest that have grown beyond the Faraday Discussion, and hoping to underscore points raised in previous meetings that we still struggle to address. In the remaining portion of this introduction, we will highlight the major challenges/opportunities that remain to be addressed. Some of these challenges have been considered in previous meetings but remain significant to moving toward the horizon. We describe these challenges/opportunities as bridges that we have yet to build or realize.
Bridges of scale
Nanoelectrochemistry is defined by scale, with space and time linked through the diffusion coefficient. In a sense we have been hyper focused on reaching ever smaller scales. We should be careful not to lose sight of what is important. We are in danger of the streetlight effect, described effectively as follows: “A police officer is patrolling a neighborhood when he sees a man … crawling around underneath a streetlight. The officer walks over to the man and asks if there is a problem. The drunkard turns to the officer and conveys that he dropped a quarter and was trying to find it. The officer peruses the area and after observing nothing in the light emanating from the streetlight, he asks the man where exactly he dropped it. With this, the drunken man replies that he dropped it two blocks away. When the police officer asks him why he is looking for his money all the way over here, the man replies, ‘Because the light is better here’.”31 We must be careful that our light – clever nanoelectrochemical tools – doesn't keep us from logical approaches to discovery that really address the question we hope to answer. For instance, if we want to justify better catalyst production, a few measurements on single nanoparticles might not tell us anything about what is driving effects seen in ensemble measurements or at larger scales. If we want nanoelectrochemistry to find greater impact, we need to link observations made with state-of-the-art tools and phenomena to what is relevant to more common larger-scale studies, i.e. is what we are measuring or studying important?
A recent example of an attempt to bridge nanoelectrochemical measurements to larger scales was described by Kang et al.32 They used different sizes of SECCM probes (120 nm, 440 nm, 5 μm, and 50 μm) to achieve a literal bottom-up study of OER at β-Co(OH)2 particles, all within the same experimental configuration (Fig. 9). This approach gave an expanded insight that combines the consideration of nanoscale defects and edges at the sub-entity scale; particle size and shape at the single-particle level; as well as the physical arrangement of particles in ensemble measurements. Consequently, the study revealed that particle shapes and sizes are less influential compared to critical factors such as intrinsic electrical conductivity, direct contact between particles and the support electrode; and the density of particle arrangements. Notably, these conclusions could not have been derived from nanoelectrochemistry alone and further make a convincing case for multiscale electrochemical measurements to build a comprehensive understanding of factors influencing electrochemical activity. The style of studies is akin to asking for “systems nanoelectrochemistry” and has been raised previously. Now seems like the right time to start thinking about how we deliver on this need.
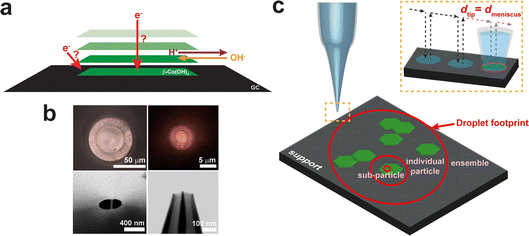 |
| Fig. 9 (a) Schematic illustrating the possible electron-transfer and ion transport pathways during OER catalysis at the β-Co(OH)2 particle supported on GC. (b) Two optical (top), one scanning electron microscopy (SEM) (bottom left), and one scanning transmission electron microscopy (STEM) (bottom right) images of SECCM probes that were used for SECCM. (c) Multiscale SECCM and how the size of the probe determines the length scale of the measurement: ensemble, individual particle, or subparticle. The diameter of the tip (dtip) corresponds approximately to the diameter of the meniscus (dmeniscus) in the hopping mode (inset).32 | |
Bridges in science
The “hype cycle” in technology development is always a concern.33 Where overpromised expectations lead to hyperinflated expectations. When those expectations are not met, a “trough of disillusionment” takes over and the field decays. The breadth of nanoelectrochemistry would likely insulate a complete collapse of the field, but on the other hand, nanoelectrochemistry can be really hard to implement relative to traditional measurements, which can dampen uptake if real gains and advantages are not realized. To make sure this doesn't happen we need to make direct connections – bridges – to other areas of science. This seems obvious, but as a community, we must work to underscore the importance of nanoelectrochemistry outside of our own circles, not just our own research, but that of the larger community. In my own research, I've started much more intense collaborations with materials scientists who are mostly interested in using electrochemistry, but not in developing it. This takes a lot of effort, but to be honest is sometimes really rewarding. We meet weekly and I enjoy getting a chance to explain fundamental electrochemistry. This bridge needs to conduct traffic in two directions and allow nanoelectrochemistry to also enhance our ability to draw from other areas, in particular in areas such as data science and programming, this is described in more detail below. In short, we need to broaden the circle, build outside of our immediate areas and highlight opportunities to cross populate research opportunities.
Bridges in education
Unwin highlighted the need for new paradigms in education for nanoelectrochemistry previously.1 This doesn't seem to have been adequately addressed – with the possible exceptions of examples provided by Unwin – and remains an outstanding problem. The problems being tackled in nanoelectrochemistry often require a fundamental grounding in chemistry, math, programming, data science, well beyond what typical undergraduate programs provide (in the US at least). We are asking a lot of students who pursue research in nanoelectrochemistry. How can we tackle this need globally? Meetings like Faraday Discussions are important but are limited in scope and further hit students late in the path of an education. Electrochemical societies – the International Society for Electrochemistry, The Electrochemical Society – might be avenues to address the present shortcomings. The importance of nanoelectrochemistry in the technological development for the energy sector cannot be understated. In my experience, students with fundamental training in electrochemistry are in high demand, and nanoelectrochemistry is an excellent venue to provide this training. How we build the bridge that leads students to that training path is a question we need to consider more fully.
Bridges to people and places
In the introduction, I alluded to the idea that post-pandemic we, meaning the scientific discipline in total, seem different, more and less at the same time. This bridge goes beyond nanoelectrochemistry specifically but is timely and worth discussion. We seem to be more in that we are more connected than ever before through technology. The connectivity of the internet and the pressure to adapt forced upon us by the pandemic have made it possible to meet with almost anyone, anywhere. At this meeting we have attendees participating wholly electronically, this has a chance to really open up science and democratize participation. But are we opening things up? Professional societies must consider ways to make electronic attendance at meetings and conferences more affordable and more accommodating for undergraduate and early graduate students. The new norm of video conferencing, I believe has also made us less. Previous Faraday Discussions I attended were instrumental in helping me to forge personal connections that last to this day. And they were not all manufactured by the physical meeting. We know that sitting down and eating a meal and socializing with colleagues and diverse scientists is important to intellectual development. We aren't getting that with virtual meetings. I think this is even more important for early career faculty and senior graduate students/postdocs. An additional aspect, which I do not care to discuss at this Discussion, but should be noted, is the polarized nature of the political climates in which scientists must operate today. We need to work, within the parameters dictated by our institutions, to keep exchange of ideas in fundamental science alive. The bridges we need to build and repair here are between people and places. Doing this in a conscientious manner should be high on our list of priorities.
Conclusion
Nanoelectrochemistry is a healthy, vibrant area of scientific enquiry and has incredible importance in society today. This introduction has tried to highlight some of the important outcomes of previous meetings in this series, hopefully providing salient examples when possible. We have repeated and revisited some of the key challenges in nanoelectrochemistry through this series, and an attempt has been made to frame these challenges as opportunities where we can build bridges to travel beyond where we stand today. To those seeking the horizon in nanoelectrochemistry, fear not, the sun has not set and is unlikely to do so in the near future.
Data availability
Data are available on request from the authors.
Conflicts of interest
There are no conflicts to declare.
Acknowledgements
Authors wish to acknowledge support for our work in nanoelectrochemistry, which includes the National Science Foundation Division of Chemistry Center for Chemical Innovation Program (award 2221062, Center for Single-Entity Nanochemistry and Nanocrystal Design), NSF CMI award 2220852, the US Army Research Office (W911NF2210051) and the Welch Foundation (award A-2091-20220331).
References
- P. Unwin, Faraday Discuss., 2022, 233, 374–391 RSC.
- R. M. Crooks, Faraday Discuss., 2016, 193, 533–547 RSC.
- Y. Wang, X. Shan and N. Tao, Faraday Discuss., 2016, 193, 9–39 RSC.
- Y. Wu, S. Jamali, R. D. Tilley and J. J. Gooding, Faraday Discuss., 2022, 233, 10–32 RSC.
- M. A. Edwards, D. A. Robinson, H. Ren, C. G. Cheyne, C. S. Tan and H. S. White, Faraday Discuss., 2018, 210, 9–28 RSC.
- P. W. Bohn, Science and technology of electrochemistry at nano-interfaces: concluding remarks, Faraday Discuss., 2018, 210, 481–493 RSC.
- D. Martín-Yerga, P. R. Unwin, D. Valavanis and X. Xu, Correlative co-located electrochemical multi-microscopy, Curr. Opin. Electrochem., 2023, 42, 101405 CrossRef.
- M. Jaugstetter, N. Blanc, M. Kratz and K. Tschulik, Electrochemistry under confinement, Chem. Soc. Rev., 2022, 51, 2491–2543 RSC.
- X. Li, Y.-H. Fu, N. Wei, R.-J. Yu, H. Bhatti, L. Zhang, F. Yan, F. Xia, A. G. Ewing, Y.-T. Long and Y.-L. Ying, Emerging Data Processing Methods for Single-Entity Electrochemistry, Angew. Chem., 2024, 136, e202316551 CrossRef.
- W. Shi, A. K. Friedman and L. A. Baker, Nanopore Sensing, Anal. Chem., 2017, 89, 157–188 CrossRef CAS PubMed.
- S. M. Oja, Y. Fan, C. M. Armstrong, P. Defnet and B. Zhang, Nanoscale Electrochemistry Revisited, Anal. Chem., 2016, 88, 414–430 CrossRef CAS PubMed.
- L. A. Baker, Perspective and Prospectus on Single-Entity Electrochemistry, J. Am. Chem. Soc., 2018, 140, 15549–15559 CrossRef CAS PubMed.
- L. Zhang, O. J. Wahab, A. A. Jallow, Z. J. O'Dell, T. Pungsrisai, S. Sridhar, K. L. Vernon, K. A. Willets and L. A. Baker, Recent Developments in Single-Entity Electrochemistry, Anal. Chem., 2024, 96, 8036–8055 CrossRef CAS.
- C. L. Bentley, Scanning electrochemical cell microscopy for the study of (nano)particle electrochemistry: From the sub-particle to ensemble level, Electrochem. Sci. Adv., 2022, 2, e2100081 CrossRef CAS.
- D. Polcari, P. Dauphin-Ducharme and J. Mauzeroll, Scanning Electrochemical Microscopy: A Comprehensive Review of Experimental Parameters from 1989 to 2015, Chem. Rev., 2016, 116, 13234–13278 CrossRef CAS PubMed.
- F. T. Patrice, K. Qiu, Y.-L. Ying and Y.-T. Long, Single Nanoparticle Electrochemistry, Annu. Rev. Anal. Chem., 2019, 12, 347–370 CrossRef.
- Y.-Y. Peng, R.-C. Qian, M. E. Hafez and Y.-T. Long, Stochastic Collision Nanoelectrochemistry: A Review of Recent Developments, ChemElectroChem, 2017, 4, 977–985 CrossRef CAS.
- X. Xu, D. Valavanis, P. Ciocci, S. Confederat, F. Marcuccio, J.-F. Lemineur, P. Actis, F. Kanoufi and P. R. Unwin, The New Era of High-Throughput Nanoelectrochemistry, Anal. Chem., 2023, 95, 319–356 CrossRef CAS PubMed.
- D. W. Deamer and D. Branton, Characterization of Nucleic Acids by Nanopore Analysis, Acc. Chem. Res., 2002, 35, 817–825 CrossRef CAS.
-
W. H. Coulter, Means for counting particles suspended in a fluid, US Pat., 2656508, 1949.
- D. Deamer, M. Akeson and D. Branton, Nat. Biotechnol., 2016, 34, 518–524 CrossRef CAS PubMed.
-
P. Plait, How Far Awayis
the Horizon?, https://www.scientificamerican.com/article/how-far-away-is-the-horizon/ (accessed 28 September 2024).
- M. E. Snowden, A. G. Güell, S. C. S. Lai, K. McKelvey, N. Ebejer, M. A. O'Connell, A. W. Colburn and P. R. Unwin, Scanning Electrochemical Cell Microscopy: Theory and Experiment for Quantitative High Resolution Spatially-Resolved Voltammetry and Simultaneous Ion-Conductance Measurements, Anal. Chem., 2012, 84, 2483–2491 CrossRef CAS PubMed.
- J. Zhang, W. He, T. Quast, J. R. C. Junqueira, S. Saddeler, S. Schulz and W. Schuhmann, Angew. Chem., Int. Ed., 2023, 62, e202214830 CrossRef CAS.
- O. J. Wahab, E. Daviddi, B. Xin, P. Z. Sun, E. Griffin, A. W. Colburn, D. Barry, M. Yagmurcukardes, F. M. Peeters, A. K. Geim, M. Lozada-Hidalgo and P. R. Unwin, Proton transport through nanoscale corrugations in two-dimensional crystals, Nature, 2023, 620, 782–786 CrossRef CAS PubMed.
- R. Pan, D. Wang, K. Liu, H.-Y. Chen and D. Jiang, Electrochemical Molecule Trap-Based Sensing of Low-Abundance Enzymes in One Living Cell, J. Am. Chem. Soc., 2022, 144, 17558–17566 CrossRef CAS.
- Y. Yang, Y. Ma, J. F. Berengut, L. K. Lee, R. D. Tilley, K. Gaus and J. J. Gooding, Nat. Photonics, 2024, 18 CAS.
- R. Ding, X. Wang, A. Tan, J. Li and J. Liu, ACS Catal., 2023, 13267–13281 CrossRef CAS.
- K.-L. Xin, Z.-L. Hu, S.-C. Liu, X.-Y. Li, J.-G. Li, H. Niu, Y.-L. Ying and Y.-T. Long, 3D Blockage Mapping for Identifying Familial Point Mutations in Single Amyloid-β Peptides with a Nanopore, Angew. Chem., Int. Ed., 2022, 61, e202209970 CrossRef CAS.
- A. D. Pendergast, K. J. Levey, J. V. Macpherson, M. A. Edwards and H. S. White, Electric Potential-Driven Acid/Base Chemistry: Kinetics of Electrochemical Interfacial Proton Transfer and Transport, J. Phys. Chem. C, 2024, 128, 7127–7136 CrossRef CAS.
- M. Battaglia and M. A. Atkinson, The Streetlight Effect in Type 1 Diabetes, Diabetes, 2015, 64, 1081–1090 CrossRef CAS.
- M. Kang, C. L. Bentley, J. T. Mefford, W. C. Chueh and P. R. Unwin, Multiscale Analysis of Electrocatalytic Particle Activities: Linking Nanoscale Measurements and Ensemble Behavior, ACS Nano, 2023, 17, 21493–21505 CrossRef PubMed.
- O. Dedehayir and M. Steinert, The hype cycle model: A review and future directions, Technol. Forecast. Soc. Change, 2016, 108, 28–41 CrossRef.
|
This journal is © The Royal Society of Chemistry 2025 |
Click here to see how this site uses Cookies. View our privacy policy here.