DOI:
10.1039/D3FO03673A
(Paper)
Food Funct., 2024,
15, 569-579
Effects of pectin's degree of methyl esterification on TLR2-mediated IL-8 secretion and tight junction gene expression in intestinal epithelial cells: influence of soluble TLR2†
Received
31st August 2023
, Accepted 19th December 2023
First published on 20th December 2023
Abstract
This study investigates the anti-inflammatory effects of pectins with different degrees of methyl esterification (DM) on intestinal epithelial cells (IECs) expressing low and high levels of TLR2. It also studies the influence of soluble TLR2 (sTLR2) which may be enhanced in patients with inflammatory bowel syndrome on the inflammation-attenuating effects of pectins. Also, it examines the impact of pectins on tight junction gene expression in IECs. Lemon pectins with DM18 and DM88 were characterized, and their effects on TLR2-1-induced IL8 gene expression and secretion were investigated in low-TLR2 expressing Caco-2 and high-TLR2 expressing DLD-1 cells. The results demonstrate that both DM18 and DM88 pectins can counteract TLR2-1-induced IL-8 expression and secretion, with more pronounced effects observed in DLD-1 cells expressing high levels of TLR2. Furthermore, the presence of sTLR2 does not interfere with the attenuating effects of low DM18 pectin and may even support its anti-inflammatory effects in Caco-2 cells. The impact of pectins and sTLR2 on tight junction gene expression also demonstrates cell-type-dependent effects. Overall, these findings suggest that low DM pectins possess potent anti-inflammatory properties and may influence tight junction gene expression in IECs, thereby contributing to the maintenance of gut homeostasis.
Introduction
During recent years, it has become accepted that an increased intake of dietary fiber (DF) promotes health and is associated with a lower incidence and frequency of inflammatory diseases, including inflammatory bowel disease (IBD).1 This association is attributed to the impact of DFs on gut microbiota composition and its microbial metabolites, as well as direct stimulation of immune receptors, thereby enhancing gastrointestinal (GI) immunity.2
Pectin, a soluble DF, is well-studied and recognized for its health-promoting effects. Pectins are derived from various fruits and vegetables,3 and are heteropolysaccharides primarily composed of galacturonic acid (GalA) residues. Structurally, pectins consist of different components, such as homogalacturonan (HG), xylogalacturonan, arabinan, arabinogalactan I, arabinogalactan II, rhamnogalacturonan I (RG-I), and rhamnogalacturonan II (RG-II).4 Some pectins contain regions with a linear backbone of 1,4-linked α-D-GalA residues (HG), which are methyl-esterified at the C6 carboxyl group and acetylated at O-2 or O-3.2,4,5
An area of increasing research interest is the health impact of pectins with a low degree of methyl esterification (DM).5 Interestingly, pectins with DM values below 25 stimulate the growth of beneficial bacteria in the large intestine and contribute to small intestine health by directly binding to pattern recognition receptors (PRRs) such as Toll-like receptors (TLRs).2 The small intestine, characterized by a thinner mucus layer, is the primary site of direct PRR–pectin interactions, allowing pectins to activate the GI immune system. Notably, pectins with a DM below 25 inhibit the pro-inflammatory TLR2-1 receptor expressed on immune and gut epithelial cells (IECs).6 However, evidence suggests that TLR2 modulation is cell-type specific,7 exhibiting differences between immune cells and IECs. In homeostasis, diverse negative endogenous feedback mechanisms, including soluble TLR2 (sTLR2) suppress potential pro-inflammatory responses via TLR2 in IECs. The reduction or reversal of inflammation by pectin is often attributed to the inhibition of TLR2-1 signaling,6,8,9 as pectin administration does not induce short-chain fatty acid (SCFA) production in mice or humans.6,10 Currently, TLR2 signaling plays a critical role in inflammation in doxorubicin-induced ileitis,6 and the use of lower DM pectins has shown success in preventing gut inflammation in vivo.6 Mechanistically, low DM pectin suppresses TLR-induced inflammatory cytokine expression, as demonstrated in a mouse model of endotoxin-induced shock.11
Moreover, lower DM pectins have shown promise in reducing inflammatory events or extending remission periods between flares in IBD patients.6,8–10,12–14,14,15,15 However, evidence suggests that DF may be less effective in TLR2-1 signaling in IBD patients compared to other target groups.16 One possible reason is the increased content of sTLR2 in the mucosa of ulcerative colitis (UC) patients,17 which may interfere with the efficacy of lower DM pectins in attenuating TLR2-1-induced inflammation.
In this study, we aimed to achieve several objectives. Firstly, we investigated the anti-inflammatory effects of lemon pectins with different DM on IECs expressing low TLR2 levels (Caco-2) and high TLR2 levels (DLD-1). Secondly, we examined whether pectin could lower TLR2-1-induced inflammation in the presence of sTLR2. Finally, we determined the impact of DM18 pectin, DM88 pectin, and sTLR2 (individually or combined) on the expression of tight junction (TJ)-related genes in IECs, with or without exposure to sTLR2, to further elucidate the underlying mechanisms that contribute to gut homeostasis.
Materials and methods
Chemicals reagents
TLR2-ligand Pam3CSK4 was purchased from Invivogen. Recombinant human Toll-like receptor 2 (rhTLR2 ectodomain) was obtained from R&D Systems, USA. Pectin lyase (EC 4.2.2.10) from Aspergilus niger18 and endo-polygalacturonase (endo-PG, EC 3.2.1.15) from Kluyveromyces fragilis19 were used to degrade the lemon pectins. These chemicals were purchased from Sigma Aldrich (St Louis, MO, USA), VWE International (Radnor, PA, USA), or Merck (Darmstadt, Germany).
Pectin samples
Pectin from lemon origin DM18 and DM88 were obtained from CP kelco (Copenhagen, Denmark). Structural characteristics were determined as described below.
Determination of monosaccharide composition
Neutral sugar composition of pectins was analyzed after pre-hydrolysis with 72% (w/w) H2SO4 (1 h, 30 °C).20 Neutral sugars were reduced with sodium borohydride, forming their corresponding alditols and then acetylated to yield their volatile derivates. These alditol acetates were separated and quantified by gas–liquid chromatography equipped with a flame-ionization detector (FID) and a 15 m DB-225 column (Agilent J&W, Santa Clara, CA, USA), as previously described.20 Inositol was used as internal standard. The uronic acid content was determined by the automated colorimetric m-hydroxydiphenyl method.21
Enzymatic hydrolysis
The enzymes used in this study were pectin lyase (EC 4.2.2.10)18 and endo-polygalacturonase from Kluyveromyces fragilis.19 All lemon pectins were dissolved in 50 mM sodium acetate buffer pH 5.2 (5 mg mL−1). The hydrolysis was performed at 40 °C by incubation of the pectin solution with pectin lyase for 6 hours followed by addition of endo-PG and incubated for another 18 hours. Enzyme doses were sufficient to degrade the pectin backbone within 24 hours. Inactivation of enzymes was performed at 100 °C for 10 min, and the digests were centrifuged (20
000g, 20 °C, 15 min). The supernatants obtained were analyzed by high-performance size exclusion chromatography (HPSEC), high-performance anion ex-change chromatography (HPAEC-PAD/UV) and Ultra-High Pressure Liquid Chromatography HILIC-ESI-IT-MSn.
High performance size exclusion chromatography (HPSEC)
Pectin before and after enzymatic digestion were analyzed using an Ultimate 3000 system (Dionex, Sunnyvale, CA, USA). A set of four TSK-Gel super AW columns (Tosoh Bioscience, Tokyo, Japan) was used in series: guard column (6 mm ID × 40 mm) and the columns TSK super AW 4000, 3000 and 2500 (6 mm × 150 mm). The column temperature was set to 55 °C. Samples (10 μL, 2.5 mg mL−1) were eluted with filtered 0.2 M NaNO3 at a flow rate of 0.6 mL min−1. The elution was monitored by refractive index detection (Shodex RI 101; Showa Denko K.K., Tokyo, Japan). The HPSEC system was calibrated using polydisperse pectin standards having molecular weights ranging from 10 to 100 kDa as estimated by viscometry.22 To clearly display the molecular weight of pectins larger than 100 kDa, 150 kDa was calculated from the standards. Molecular weights presented were estimated at the top of the curve, despite slight differences in elution patterns of the various pectins indicating differences in polydispersity.
High performance anion exchange chromatography (HPAEC)
The pectin digests were analyzed and subsequently quantified using an ICS5000 High Performance Anion Exchange Chromatography system with Pulsed Amperometric detection (ICS5000 ED) (Dionex Corporation, Sunnyvale, CA, USA) equipped with a CarboPac PA-1 column (250 mm × 2 mm i.d.) and a CarboPac PA guard column (25 mm × 2 mm i.d.). The two mobile phases were (A) 0.1 M NaOH and (B) 1 M NaOAc in 0.1 M NaOH and the column temperature was 20 °C.23 GalA DP 1–3 (Sigma–Aldrich, Steinheim, Germany) were used as standards for quantification. UV-monitoring of the eluent at 235 nm allowed the identification of unsaturated oligoGalAs as released by the action of pectin lyase through the presence of a double bond. Before the analysis pectin digests were diluted using ultra-pure water to 0.5 mg mL−1. They were injected (10 μL) and eluted at a flow rate of 0.3 mL min−1. The gradient profile for elution was as follows: 0–55 min, linear 20–65% B; 55.1–60 min column washing with 100% B; finally, column re-equilibration with 20% B from 60.1–75 min.
Ultra-high pressure liquid chromatography HILIC-ESI-IT-MSn
Pectin digests were analyzed using UHPLC in combination with ESI-IT-MSn on a Hydrophilic Interaction Liquid Chromatography (HILIC) BEH amide column 1.7 μm, 2.1 × 150 mm (Thermo Scientific). Pectin digests were centrifuged (15
000g, 10 min, room temperature) and diluted (with 50% (v/v) acetonitrile containing 0.1% formic acid, to a final concentration of 1 mg mL−1). The eluents used were (A) 99
:
1% (v/v) water/acetonitrile (water/ACN); (B) 100% ACN, both containing 0.1% formic acid with a flow rate of 400 μL min−1. The following elution profile was used: 0–1 min, isocratic 80% B; 1–46 min, linear from 80% to 50% B; followed by column washing: 46–51 min, linear from 50% to 40% B and column re- equilibration; 51.1–60 min isocratic 80% B with a flow rate of 400 μL min−1. Oven and tray temperatures were kept at 40 °C. Mass spectra were acquired over the scan range m/z 300–2000 in the negative mode. Heated Electrospray Ionization Ion Trap ionized the separated oligomers in an LTQ Velos Pro Mass Spectrometer (UHPLC-ESI-IT-MSn) coupled to the UHPLC. The ratio of triGalA with or without methyl esterification was calculated from the peak area.
Determination of degree of methyl-esterification and degree of blockiness
Pectin samples (5 mg) were saponified using 1 mL of 0.1 M NaOH for 24 hours (1 hour at 4 °C, followed by 23 hours incubation at room temperature). To the pectin blank, 1 mL of ultra-pure water was added. The head-space vials were immediately sealed with a Teflon lined rubber septum. To determine the degree of methyl-esterification (DM) a gas chromatography method was used as previously described.24 The degree of blockiness (DB) was determined as previously described.25
Cell culture
The human colonic adenocarcinoma cell line, Caco-2 and DLD-1 were obtained from ATCC (ATCC HTB-37) and were used between passages 10–20 for all experiments. Caco-2 cells were cultured in DMEM (Gibco, Invitrogen, MA USA) supplemented with 10% heat-inactivated fetal calf serum (Sigma-Aldrich, MO USA), 1% Penicillin/Streptomycin, and 1% MEM nonessential amino acid solution (Corning, Mediatech Inc. NY USA). DLD-1 were cultured in RPMI 1640 medium (Gibco, Invitrogen, MA USA) with 10% fetal bovine serum (Sigma-Aldrich, MO USA), 2 mM L-glutamine (Lonza, Belgium), 60 μg mL−1 gentamicin sulfate (Lonza, Belgium). Cells were maintained at 37 °C, 5% CO2, trypsinized until they reached near confluency and were used for cell incubation experiments.
RNA isolation and cDNA synthesis
After the assays, cells were washed two times with cold Dulbecco's phosphate buffered saline (DPBS) (Gibco, Gaithersburg, MD, USA). Then, the cells were treated with Trizol® Reagent (Invitrogen) for RNA isolation as described by the manufacturer. RNA concentration and purity were measured with a NanoDrop ND-100 UV-Vis spectrophotometer (NanoDrop Technologies, Wilmington, DE, USA). Aliquots of 200 ng of total RNA were used for reverse transcriptase cDNA synthesis. The reverse transcription (RT) reaction was performed in total RNA following a standard protocol. Briefly, the isolated RNA was treated with DNAse I (Thermo Fisher Scientific Inc., Landsmeer, The Netherlands) following the protocol described by the manufacturer. Then DNAse-treated RNA aliquots were incubated with 300 ng of random hexamer primers and 10 mmol L−1 dNTP for 5 minutes at 65 °C and followed by cooling down the samples on ice. After this, 5× First-Strand buffer, 0.5 μL (20 U) of RiboLock RNase Inhibitor (#EO0381), 2 μL (1 mM) of dNTP mix (10 mM each; #R0191) and 1 μL (200 U) of RevertAid Reverse Transcriptase (Thermoscientific, #EP0441) were added obtaining a final volume of 20 μL. The mix was then incubated using a T100™ Thermal Cycler (Bio-Rad, California, USA) at 25 °C for 10 minutes, 42 °C for 60 minutes and 70 °C for 10 minutes. The cDNA product was stored at −20 °C until use.
Quantitative polymerase chain reaction (qPCR)
Total cDNA was diluted 20 times with nuclease-free water and then 5 μL was mixed with FastStart Universal SYBR Green Master (Roche Diagnostics, Basel, Switzerland). SYBR green forward and reverse primers (6 μmol L−1) were used at a final concentration of 300 nmol L−1. qPCR for TLR2, IL8, CLDN1, CLDN2, CLDN, OCLN 3 and ZO-1 genes was performed in ViiATM 7 Real-Time PCR System (Applied Biosystems, Foster City, CA, USA) with a cycling protocol as follows: 40 cycles of 15 seconds at 95 °C for denaturation, 30 seconds at 60 °C for primer annealing and 30 seconds of extension at 72 °C also including a melting curve. For detailed primer sequence see Table 1. Mean Ct of Glyceraldehyde-3-phosphate dehydrogenase (GAPDH) was used as housekeeping gene. Changes in expression were analyzed using 2-ΔΔCt.
Table 1 Primer sequences
Gene |
Symbol |
Forward (5′ → 3′) |
Reverse (5′ → 3′) |
Glyceraldehyde-3-phosphate dehydrogenase |
GAPDH
|
AGCCACATCGCTCAGACAC |
GCCCAATACGACCAAATCC |
Toll-like receptor 2 (TLR2) |
TLR2
|
GCCTCTCCAAGGAAGAATCC |
TCCTGTTGTTGGACAGGTCA |
Interleukin-8 (IL-8) |
IL8
|
CTTTCAGAGACAGCAGAGCA |
ACACAGAGCTGCAGAAATCA |
Claudin-1 |
CLDN1
|
GATGAGGTGCAGAAGATGAG |
GGACAGGAACAGCAAAGTAG |
Claudin-2 |
CLDN2
|
CTGCTCATCCAGAGAAATC |
CTGTCAGGCTGTAGGAATTG |
Claudin-3 |
CLDN3
|
CTGCTCTGCTGCTCGTGTC |
CGTAGTCCTTGCGGTCGTAG |
Occludin |
OCLN
|
CTATAAATCCACGCCGGTTC |
TATTCCTGTAGGCCAGTGTC |
Zonula occludens-I |
ZO-1
|
CGGTCCTCTGAGCCTGTAAG |
GGATCTACATGCGACGAGACAA |
Immunoblotting
Caco-2 and DLD-1 cells were rinsed with ice-cold PBS and lysed using RIPA buffer supplemented with a protease inhibitor (Ameresco, USA). The cell lysates were then homogenized by sonication (2 × 5 s, power at 30%) and centrifuged at 12.000 rpm at 4 °C for 15 min. Protein levels were quantified using the Pierce BCA assay kit (Thermo Scientific, USA) according to the manufacturer's instructions. The lysate containing 60 μg protein was mixed with 5× loading buffer (containing glycerol and 1 M Tris-HCl, pH 6.8), boiled for 7 min, and subjected to SDS-PAGE gel electrophoresis (10% resolving gel and 4% stacking gel). The separated proteins were then electrotransferred to a nitrocellulose membrane (BioRad). To confirm the complete transfer of the blot, Ponceau S staining was performed. Non-specific binding sites were blocked by incubating the membranes with 5% BSA in TBS-T (2 mM Tris-HCl, pH 7.6, 13.7 mM NaCl, 0.1% Tween 20) for 1 hour at room temperature. The membranes were then probed with specific primary antibodies, which were diluted in 5% BSA in TBS-T. The primary antibodies used were rabbit monoclonal anti-TLR2 (1
:
500 dilution; #EPR2078Y; IgG; Abcam) and mouse monoclonal anti-β-actin (1
:
1000 dilution; #8H10D10; IgG2b; Cell Signaling Technology). After washing with TBS-T, the membranes were incubated with the secondary antibodies polyclonal goat anti-rabbit-HRP (1
:
1000 dilution; #P0448; IgG; Dako) and polyclonal rabbit anti-mouse-HRP (1
:
10
000 dilution; #P0260; Dako). Immunoreactive bands were visualized using a ChemiDoc imaging system by adding a mixture of luminol/enhancer solution and stable peroxide solution (SuperSignal™ West Pico PLUS Chemiluminescent Substrate) and analyzed using ImageJ software.
Cytokine levels in cell supernatant
Interleukin-8 (IL-8) from cell supernatants is a marker of inflammatory response in both Caco-2 and DLD-1 cells after a challenge with the disruptor molecule Pam3CSK4.26–28 Therefore, a possible mitigation of pectin induced attenuation of cytokine release was studied by pre-treating IECs with sTLR2 and the pectins for 1 hour, followed by treatment with TLR2 agonist. Cell supernatants were collected and centrifuged, and IL-8 was further quantitated by ELISA according to the manufacturer's instructions (R&D Systems, Biotechne, Minneapolis, USA). Cells without any treatment served as controls.
TLR2-1 inhibition and activation by pectins
Both Caco-2 and DLD-1 cells were seeded (2 × 105 cells per ml in 6-well plates) and grow for 48 hours. As these cell lines showed different sensitivities for pectin, we first tested in a pilot study the inhibitory capacity on TLR2-induced IL-8 secretion using 1 and 2 mg ml−1 DM18 pectin (ESI†). As shown in Fig. S1,† at 1 mg ml−1 we saw inhibition on IL-8 secretion by DM18 pectin on Caco-2, while not in DLD-1 cells, although with 2 mg ml−1, we saw inhibition of IL-8.
Subsequently, to study whether DM18 and DM88 pectins activate or inhibit TLR2, cells were incubated at a concentration of pectin 1 mg mL−1 for Caco-2 cells and at 2 mg mL−1 for DLD-1 cells or with Pam3CSK4 (5 μg mL−1). Cells without any pectin exposure served as controls. Inhibition of TLR2-1 was studied by pre-treating Caco-2 or DLD-1 cells with pectins for 1 hour at the indicated concentrations, followed by TLR2-1 agonist treatment for 24 hours. Afterwards, cells were collected for determining expression of TLR2, TJs-related genes and cytokines by qPCR. Medium was collected for quantifying cytokine production by ELISA.
Possible interference of soluble TLR2 with pectin attenuate cytokine production in IECs
Caco-2 and DLD-1 cells were pretreated with soluble TLR2 (sTLR2) in the presence and absence of DM18 or DM88 pectin to study possible inhibiting effects of soluble TLR2 (sTLR2) on pectin induced IL-8 production. To this end, Caco-2 and DLD-1 cells were seeded at 2 × 105 cells per ml in 6-well plates. Cells were allowed to grow for 48 hours. The following day, cells were incubated in the presence and absence of either low DM18 or high DM88 pectins in combination with sTLR2. This sTLR2 contain the extracellular domain of TLR2 (sTLR2; 150 ng mL−1)29 or the TLR2 agonist as control (Pam3CSK4; 5 μg mL−1).28 Possible mitigation of pectin induced attenuation of cytokine release was studied by pre-treating Caco-2 or DLD-1 cells with sTLR2 and the pectins for 1 hour, followed by treatment with TLR2 agonist. The relative expression and the production of cytokines were determined by qPCR and ELISA, respectively.
Statistical analysis
GraphPad Prism version 10.0.0 was used for statistical analyses. Normal distribution of data was examined by the Shapiro–Wilk test. The data were normally distributed. Therefore, data are expressed as mean ± standard error of the mean (SEM) from at least five independent assays. In all experiments statistical significance was determined using one-way ANOVA with Sidák's multiple comparison test. A p-value < 0.05 was considered significant. * (p < 0.05), ** (p < 0.01), ***(p < 0.001), **** (p < 0.0001).
Results
Pectin characterization
Pectins obtained from lemon were characterized for the DM, molecular weight, and sugar composition, as shown in Table 2. The degree of blockiness was calculated as previously described.15 The methyl-esterification of two pectins, were DM18 and DM88, whereas the blockiness was DB86 and DB91 for DM18 and DM88 pectin, respectively, (DB representing charge density, rather than total pectin charge).
Table 2 Structural characteristics of pectins. Pectin
|
Origin |
DB (%) |
M
w (kDa) |
Sugar composition (mol%) |
Carbohydrate content (w/w%) |
Rha |
Ara |
Gal |
Glc |
UA |
Degree of methyl-esterification (DM): mol of methanol per 100 mol of total GalA in the sample. Degree of blockiness (DB): the amount of mono-, di- and triGalA per 100 mol of the non-esterified GalA in the sample. Molecular weight (Mw) as measured by HPSEC. Rha = rhamnose, Ara = arabinose, Gal = Galactose, Glc = Glucose, UA = Uronic Acid. These or similar pectins have also been used.9,14,15,30,31 |
DM18 |
Lemon |
86 |
78 |
1 |
0 |
2 |
0 |
97 |
62 |
DM88 |
Lemon |
91 |
91 |
1 |
3 |
5 |
0 |
91 |
67 |
Pectins inhibit both TLR2-induced IL-8 gene expression and secretion in IECs in a DM-dependent manner
To assess the ability of pectin to stimulate or inhibit TLR2-1-induced IL-8 secretion, we applied both Caco-2 and DLD-1 IECs cell lines. We consider Caco2 cells to be low TLR2-expressing cells and DLD-1 as high TLR-2 expressing cells as we found that DLD-1 cells have a 6.4-fold higher relative TLR2 expression (p < 0.0001) compared to Caco-2 cells (Fig. 1A). In addition to mRNA expression, we also confirmed the higher expression on a protein level. Immunoblotting analysis demonstrated that DLD-1 cells exhibited a 2.1-fold higher TLR2 protein levels than Caco-2 cells (p < 0.05) (Fig. 1B).
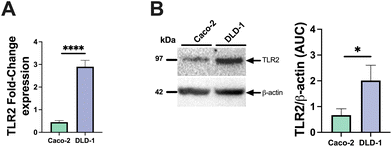 |
| Fig. 1 Putative TLR2 expression in Caco-2 and DLD-1 cells. TLR2 levels mRNA (A) and protein TLR2 levels as determined by immunoblotting (B). At least 3 independent biological and technical replicates were done. Significant differences are indicated by * (p < 0.05), ** (p < 0.01), *** (p < 0.001) or by **** (p < 0.0001). | |
Next, we studied IL-8 expression by both cell-types after exposure to the TLR2-1 specific Pam3CSK4 stimuli. As expected, Pam3CSK4 exposure significantly increased IL8 in both epithelial cell lines. As shown in Fig. 2, Pam3CSK4 induced a stronger increase in IL8 gene expression in DLD-1 cells (29.0-fold change) (Fig. 2B) compared to Caco-2 cells (14.0-fold change) (Fig. 2A). We conducted experiments to evaluate the stimulating and attenuating effects of low DM18 and high DM88 pectin on IL8 gene expression in the absence or presence of Pam3CSK4. Pectin alone did not induce any stimulating effect on IL8 expression in either Caco-2 or DLD-1 cells. However, as depicted in Fig. 2, both DM18 and DM88 pectins demonstrated the ability to counteract the TLR2-1-induced IL8 gene expression when stimulated by Pam3CSK4. The effects were more pronounced in DLD-1 cells compared to Caco-2 cells. In Caco-2 cells, both DM18 and DM88 pectins reduced the TLR2-1-induced IL8 gene expression by 12.0-fold (from 14 to 2.6, p < 0.0001) and 12.1-fold (from 14 to 2.4, p < 0.0001), respectively (Fig. 2A). In DLD-1 cells, DM18 pectin attenuated the TLR2-1-induced IL8 gene expression by 28.2-fold (from 29.2 to 1.1, p < 0.0001), while DM88 pectin exhibited a less pronounced but still significant attenuation of TLR2-1-induced IL8 gene expression by 20.1-fold (from 29.2 to 6.1, p < 0.0001) (Fig. 2B).
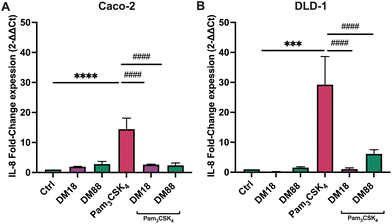 |
| Fig. 2
IL8 gene expression in Caco-2 (A) and DLD-1 (B) cells. IL8 gene expression was measured by qPCR after cells pre-treatment with DM18 and DM88 pectins, with or without Pam3CSK4 for 24 hours. Pectins downregulate IL8 mRNA levels in a DM-dependent manner in both cell lines. At least six independent experiments were performed. Data are expressed as mean ± sem. * (p < 0.05), ** (p < 0.01), *** (p < 0.001), **** (p < 0.0001) vs. control; # (p < 0.05), ## (p < 0.01), ### (p < 0.001), #### (p < 0.0001) vs. Pam3CSK4. | |
Next, we tested the stimulating and attenuating effects of low DM18 and high DM88 pectin on IL-8 secretion in absence or presence of Pam3CSK4. As shown in Fig. 3, pectin also had no stimulating effect on IL-8 secretion, in either Caco-2 or DLD-1 cells. This was different in the presence of Pam3CSK4, with effects being more pronounced on high TLR2-expressing DLD-1 cells than in Caco-2 cells. In Caco-2 cells, DM18 pectin attenuated TLR2–1-induced IL-8 secretion 4.8-fold, down from 371.6 pg mL−1 to 76.9 pg mL−1 (p < 0.0001). This was less pronounced but still significant with DM88 pectins which attenuated TLR2-1 induced IL-8 secretion 1.4-fold, down from 371.6 pg mL−1 to 262.1 pg mL−1 (p < 0.001) (Fig. 3A). In DLD-1 cells, DM18 pectin attenuated TLR2-1 induced IL-8 secretion 13.0-fold, down from 530.7 pg mL−1 to 40.5 pg mL−1 (p < 0.0001). This was less pronounced with DM88 pectins which attenuated TLR2-1 induced IL-8 secretion 1.3-fold, from 530.7 pg mL−1 to 414.0 pg mL−1 (p > 0.05) (Fig. 3B).
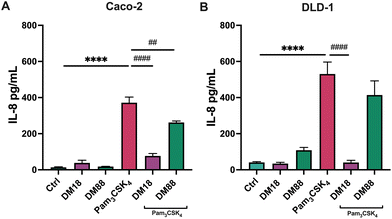 |
| Fig. 3 IL-8 production by Caco-2 (A) and DLD-1 (B) cells. Both cell lines were pre-treated with either DM18 or DM88 pectins with or without Pam3CSK4 for 24 hours, followed by IL-8 quantification in cell supernatants. Pectins decreased IL-8 secretion in a DM-dependent manner in both cell lines. At least six independent experiments were performed. Data are expressed as mean ± sem. * (p < 0.05), ** (p < 0.01), *** (p < 0.001), **** (p < 0.0001) vs. control; # (p < 0.05), ## (p < 0.01), ### (p < 0.001), #### (p < 0.0001) vs. Pam3CSK4. | |
sTLR2 does not affect pectin inhibitory effect on TLR2-1-induced IL-8 production
As in some diseased individuals, such as in colitis patients, it has been described that TLR2 might be spliced from the cell membrane and increase in the systemic circulation.30 Consequently, we also investigated whether sTLR2 might interfere with the inflammation attenuating effects of pectins. To this end, we repeated the foregoing experiment and exposed either Caco-2 or DLD-1 cells to Pam3CSK4. As shown in Fig. 4, effects were DM and cell-type-dependent. sTLR2 downregulated Pam3CSK4 induced IL8 relative expression approximately 10.8-fold and 12.6-fold in Caco-2 and DLD-1 cells, respectively. We also tested the attenuating effect of DM18 and DM88 pectin on IL8 relative expression in both cell lines in the presence or absence of sTLR2. As shown in Fig. 4A, in Caco-2 cells, sTLR2 did not reduce the attenuating effect of low DM18 pectin (2.8-fold down from 14.4-fold). Also, DM88 pectin reduced Pam3CSK4 induced IL8 in presence of sTLR2 (p < 0.05), but this attenuation was less pronounced than with sTLR2 alone. In contrast, in DLD-1 cells sTLR2 did not reduce and even supported the attenuating effect of low DM18 pectin, from 1.1-fold change in DM18 pectin alone to 0.6-fold change in presence of sTLR2 (p < 0.0001), without interfering with the attenuating effect of DM88 on Pam3CSK4 induced IL8 expression (p < 0.0001) (Fig. 4B).
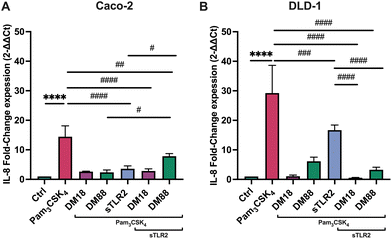 |
| Fig. 4 Impact of sTLR2 on attenuating effects of DM18 and DM88 pectin induced IL8 gene expression in Caco-2 (A) and DLD-1 (B) cells. IL8 gene expression was measured by qPCR after cells were pre-treated with DM18 and DM88 pectins in presence and absence of sTLR2 for 1 hour and after exposed to a Pam3CSK4 for 24 hours. sTLR2 did not alter IL8 reducing effects induced by pectins. At least six independent experiments were performed. Data are expressed as mean ± sem. * (p < 0.05), ** (p < 0.01), *** (p < 0.001), **** (p < 0.0001) vs. control; # (p < 0.05), ## (p < 0.01), ### (p < 0.001), #### (p < 0.0001) vs. Pam3CSK4. | |
As shown in Fig. 5, sTLR2 reduced Pam3CSK4-induced IL-8 secretion with approximately 16% and 9% in Caco-2 and DLD-1 cells, respectively, without reaching statistical significance. We also tested sTLR2 effect on the attenuating effect of DM18 and DM88 pectin on IL-8 secretion in both cell lines. Significant correlation was found between the IL-8 mRNA and the protein expression levels after the treatment with sTLR2 in DLD-1 cells. Interestingly, in the presence of sTLR2, we observed no direct correlation between IL-8 mRNA and IL-8 protein levels (Fig. 4A and 5A respectively). As shown in Fig. 5A, in Caco-2 cells sTLR2 did not affect the attenuating effect of low DM18 pectin on Pam3CSK4-induced IL-8 secretion (p < 0.05), although DM88 attenuation of IL-8 secretion was enhanced by sTLR2 (p < 0.0001). Also, in DLD-1 cells we found no interference of sTLR2 with low DM18 pectin (p < 0.001), however, on this cell line it was not observed by DM88 pectin (p > 0.05) (Fig. 5B).
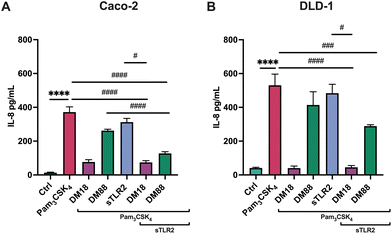 |
| Fig. 5 Impact of sTLR2 on attenuating effects of DM18 and DM88 pectin induced IL-8 production in Caco-2 (a) and DLD-1 (b) cells. Both cell lines were pre-treated with either DM18 or DM88 pectins with or without Pam3CSK4 for 24 hours, followed by quantification of IL-8 production in cell supernatants. sTLR2 did not alter IL-8 reducing effects induced by pectins, although it was DM-dependent in both cell lines. At least six independent experiments were performed. Data are expressed as mean ± sem. * (p < 0.05), ** (p < 0.01), *** (p < 0.001), **** (p < 0.0001) vs. control; # (p < 0.05), ## (p < 0.01), ### (p < 0.001), #### (p < 0.0001) vs. Pam3CSK4. | |
Pectins prevent TLR2-induced tight junction dysregulation in a DM esterification-dependent manner
To investigate whether DM18 or DM88 pectins had an impact on tight junction gene expression in Caco-2 and DLD-1 cells and whether this was influenced by sTLR2, real-time qPCR was performed on both cell lines exposed to pectins, in presence and absence of sTLR2. As shown in Fig. 6, effects were dependent on DM and cell-type.
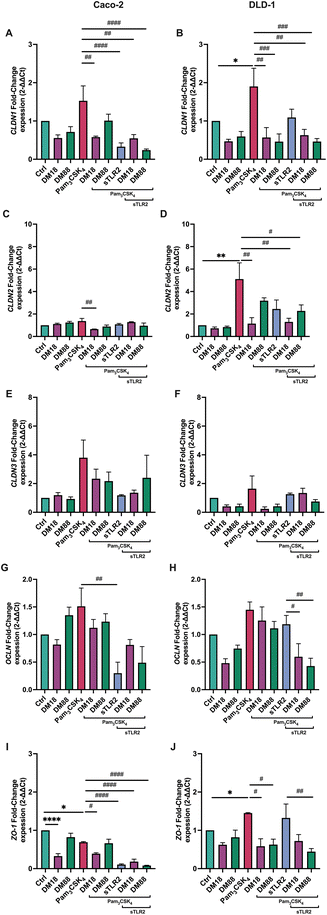 |
| Fig. 6
CLDN1 (A and B), CLDN2 (C and D), CLDN3 (e and f), OCLN (g and h) and ZO-1 (i and j) gene expression in Caco-2 and DLD-1 cells, in presence and absence of DM18 or DM88 pectin or sTLR2. Cells were tested with or without Pam3CSK4. CLDN1, CLDN2, CLDN3, OCLN and ZO-1 gene expression was measured by qPCR after cells were pre-treated with either DM18 or DM88 pectin, with or without sTLR2 for 1 hour, and after that exposure to Pam3CSK4 for 24 hours. At least six independent experiments were performed. Data are expressed as mean ± sem. * (p < 0.05), ** (p < 0.01), *** (p < 0.001), **** (p < 0.0001) vs. control; # (p < 0.05), ## (p < 0.01), ### (p < 0.001), #### (p < 0.0001) vs. Pam3CSK4. | |
Pam3CSK4 enhanced CLDN1 expression 1.5-fold (p > 0.05) in Caco-2 and 1.9-fold (p < 0.05) in DLD-1 cells. This enhancement was prevented in Caco-2 cells by DM18 pectin and sTLR2, but not by DM88 pectin. This contrasted with DLD-1 cells where DM18 and DM88 pectin (p < 0.001, and p < 0.0001, respectively) prevented CLDN1 enhancement and where sTLR2 was ineffective. The combination of DM18 or DM88 pectin and sTLR2 did not further attenuate CLDN1 expression, illustrating no synergistic or antagonistic effect (Fig. 6A and B).
For CLDN2 gene expression, results were very different in Caco-2 cells and DLD-1 cells. Pam3CSK4 did not enhance CLDN2 expression in Caco-2 cells (Fig. 6C) but did by 4.3-fold (p < 0.001) in DLD-1 cells (Fig. 6D). In Caco-2 cells there was only a statistically significant downregulation (p < 0.001) of CLDN2 by DM18 pectin when cells were exposed to Pam3CSK4. In DLD-1 cells, we observed a statistically significant prevention of Pam3CSK4-induced upregulation of CLDN2 by DM18 pectin (p < 0.001) (Fig. 6C) but not by DM88 pectin, nor by sTLR2. The combination of DM18 pectin and sTLR2 did not have any synergistic effect (p < 0.001). However, the combination of DM88 and sTLR2 (p < 0.05) prevented Pam3CSK4-induced CLDN2 upregulation, being stronger than DM88 alone (Fig. 6D).
Pam3CSK4 did enhance CLDN3 expression 3.8-fold in Caco-2 and 1.6-fold in DLD-1, but this did not reach statistical significance compared to controls. However, despite all interventions with DM18 or DM88 pectin or with sTLR2, we saw a tendency to downregulate CLDN3 expression after Pam3CSK4 challenge, although not statistically significant (Fig. 6E). Regarding DLD-1 cells, only low DM18 pectin induced a reduction of CLDN3 expression, although not statistically significant (p > 0.05) (Fig. 6F).
For OCLN gene expression, minor effects were found after the Pam3CSK4 challenge. However, some cell dependent effects were found for pectins or sTLR2 treatment. In Caco-2 cells, sTLR2 inhibited the Pam3CSK4-induced OCLN enhancement and even brought the expression under that of controls (p < 0.05) (Fig. 6G). In DLD-1 cells results were different, where although sTLR2 had no effect on Pam3CSK4-induced OCLN enhancement, the combination of DM18 or DM88 and sTLR2 reduced OCLN enhancement by Pam3CSK4 0.7-fold (p < 0.05) and 0.9-fold (p < 0.001), respectively (Fig. 6H).
Finally, also for ZO-1 gene expression, a cell-type dependent effect was observed (Fig. 6I–J). In Caco-2 cells, Pam3CSK4 downregulated ZO-1 expression 0.3-fold (p < 0.05). In Caco-2 cells, DM18 pectin reduced ZO-1 expression (p < 0.05), remaining unaffected by Pam3CSK4. Also, DM88 pectin did not reduce ZO-1 gene expression. Also, ZO-1 gene expression in presence of DM88 pectin, remained unaffected by Pam3CSK4. sTLR2 had a stronger preventive and attenuating effect on ZO-1 gene expression, being 0.6-fold lower (p < 0.0001) than in presence of Pam3CSK4. This lower ZO-1 expression induced by sTLR2 was maintained by DM18 and DM88 pectin (p < 0.001 and p < 0.0001, respectively). In DLD-1 cells, results were different again, where pectins reduced ZO-1 expression, although this was maintained and unaffected by Pam3CSK4 treatment. Both DM18 and DM88 exposed cells in presence of Pam3CSK4 had a lower ZO-1 expression than cells with Pam3CSK4 only (p < 0.05). sTLR2, however, had no effect on Pam3CSK4 induced ZO-1 expression, while it strongly attenuated ZO-1 in Caco-2 cells (p < 0.0001). The combination of sTLR2 and pectins kept ZO-1 expression levels to that of individual pectins, being unaffected by sTLR2.
Discussion
The ability of DFs to prevent common inflammatory disorders has been widely recognized,2 but the underlying mechanisms and the dependency on the DF chemical structure have not been fully elucidated. Previous studies have mainly focused on examining the effects of pectin on immune cells, such as monocytes, PRR reporter cell lines, and peripheral blood mononuclear cells,6,13,25,31–33 while the impact on intestinal epithelial cells (IECs) has received less attention. In this in vitro study, we investigated the influence of pectin on gut inflammation by using IECs with different levels of TLR2 expression. TLR2 expression can vary between individuals,34 and has been implicated in the pathogenesis of IBD.35 Our findings demonstrate that lower DM pectins effectively inhibit TLR2-induced IL8 gene expression and secretion in gut epithelial cell lines, regardless of the TLR2 expression level. The inhibitory effects were more pronounced in high-TLR2 expressing DLD-1 cells but still present in lower-TLR2 expressing Caco-2 cells.
To determine whether circulating sTLR2, commonly observed in IBD patients,30 could interfere with the efficacy of lower DM pectins in attenuating inflammation, we designed this experiment. Even though sTLR2 as such downregulates IL8 gene expression in Caco-2, it may not prevent the release of the preformed, stored IL-8, which could explain the different response at protein levels. Interestingly, we found that the anti-inflammatory effects of low-DM pectins on IL-8 secretion were not affected by the presence of sTLR2. Therefore, our data suggest that pectins can play a beneficial role in preventing or reducing symptoms and complications of chronic inflammatory diseases involving IECs, such as IBD.
Furthermore, we observed a strong dependency of the DM of pectin on efficacy in attenuating IL-8 secretion in both high- and low-TLR2 expressing cell lines. DM18 pectin was more effective than high DM88 pectin, with more pronounced effects in high TLR2-expressing DLD-1 cells. Although the attenuating effect was lower in Caco-2 cells with lower TLR2 expression, both DM18 and DM88 significantly reduced IL-8 secretion. These findings may be explained by differential TLR2 expression levels and the ability of higher DM pectins to prevent TLR2-1 dimerization.6,15 Pectin binds and inhibits TLR2 by directly interacting with amino acids at the ligand binding site of TLR2.6 It therewith specifically inhibits the proinflammatory TLR2-1 pathway while the more tolerogenic TLR2-6 pathway remains unaltered. This effect is most pronounced with pectins having a low-DM.6 This could explain our more beneficial results using low DM18. Inflammation attenuating mechanisms are dependent on the DM of pectin as low DM18 pectin strongly inhibits TLR2-1 dimerization, while high DM pectins are less efficient.25 Docking studies revealed that long non-esterified GalA sequences in DM18 pectin bind to the TLR2-1 heterodimer interface,25 providing an explanation for the stronger inhibitory capacity of pectin in high-TLR2 expressing cells.
Higher sTLR2 production in patients is directly associated with UC severity30 and might interfere with pectin's ability to lower inflammation.29,30 We however did not find such an effect. sTLR2 did not interfere with the attenuating effects of lower DM pectins on IL-8 secretion. Instead, we even found that the attenuating effect of high DM88 pectin was significantly supported by sTLR2 in Caco-2 cells. Low-DM pectin can interact with TLR2 through electrostatic forces between non-esterified galacturonic acids on the pectin and positive charges of specific amino acids on the TLR2 ectodomain,6 which is virtually having the same conformation as that of sTLR2. Docking and experimental studies indicated that DM18 and DM88 pectins interact differently with TLR2-1.6,15,25 DM18 pectin could create steric hindrance, preventing the interaction of sTLR2 with the membrane-bound receptor. However, the interaction of DM88 pectin with TLR2-1 appears to be different. To gain a better understanding of these interaction differences, future docking studies specifically investigating the interaction with sTLR2 are required.
Pam3CSK4, an activator of TLR2-dependent pathways, has been shown to increase CLDN1 expression,36 which was associated with TLR2 inhibition by pectins and sTLR2. The observed enhancement of CLDN1 expression after Pam3CSK4 challenge in both epithelial cells corresponds to its expression pattern in active IBD.37 However, this effect was restored by pectin pre-treatment and sTLR2, suggesting that pectin administration may reduce or prevent CLDN1 alterations in inflammatory pathologies.
Also, CLDN2 expression is relevant in potential IBD treatment,30 as it is upregulated in small and large intestines in immune-mediated diseases, contributing to diarrhea induction and altered luminal antigen uptake, thereby promoting inflammatory processes.38 Our data demonstrate that low DM18 pectin can prevent TLR2-mediated CLDN2 induction. This effect was dependent on TLR2 expression levels and more pronounced in high TLR2 expressing DLD-1 cells compared to lower TLR2 expressing Caco-2 cells.
CLDN3 overexpression is associated with colorectal adenocarcinoma development and malignancy.39,40 Pam3CSK4-induced CLDN3 expression was observed in both cell lines, and it was prevented by both pectins and sTLR2 in Caco-2 cells and only by low DM18 pectin in DLD-1 cells. Thus, by reducing inflammation, pectins may have positive effects on IBD and potentially lower the incidence of colorectal cancer.41
Pam3CSK4 induced upregulation of ZO-1 expression without affecting OCLN expression in DLD-1 cells but not in Caco-2 cells. This was not corroborated, which contradicts the findings of Cario et al.42 This might be explained using different Caco-2 cell clones. Some clones have been reported to be hyperresponsive to Pam3CSK4,43 possibly explaining the different responses observed. Additionally, the differences in ZO-1 expression between Caco-2 cells and DLD-1 cells can be explained by the varying TLR2 protein expression levels, as Pam3CSK4-mediated ZO-1 increase was prevented by both low DM18 and DM88 pectins. Furthermore, in Caco-2 cells with lower TLR2 expression, sTLR2 downregulated both OCLN and ZO-1 induced by Pam3CSK4, whereas this effect was not observed in DLD-1 cells, suggesting that Caco-2 cells are more sensitive to sTLR2 than DLD-1 cells.
Conclusions
In summary, this study aimed to determine whether the presence of sTLR2, commonly found in IBD patients, interferes with the attenuating effects of pectin on TLR2-1-induced inflammation. Using different TLR2-expressing cells, we demonstrated that the attenuating effects of pectin are dependent on receptor expression levels, which is particularly relevant for human application due to the variation in TLR2 expression among healthy and diseased individuals. Our findings indicate that low DM pectins have strong anti-inflammatory effects in both high- and low-TLR2 expressing cells, with greater potency in high TLR2-expressing cells. Moreover, these effects remain unchanged in the presence of sTLR2, suggesting that low-DM pectins may reduce or prevent inflammation in IBD. Additionally, we provide evidence that pectins prevent dysregulation of TJ-related genes induced by TLR2, thus contributing to the restoration of gut homeostasis. Our data shows that strength of the effects of pectin on TJ-related genes strongly depends on TLR2 expression levels, the DM of the applied pectin and the presence of sTLR2 in low-TLR2 expressing cells. Therefore, further dedicated studies should be undertaken to determine the impact of low-DM pectins on barrier function and gut homeostasis in both healthy individuals and those with inflammatory conditions.
Author contributions
N. G., M.A.H. and P.D.V designed the study. N. G. performed the experiments. X.T., X.C. assisted in pectin preparation. X.T contributed to the pectin composition analysis. S.B. assisted in the immunoblotting performance and analysis. M.A.H. and P.D.V revised and edited the manuscript. M.A.H. and P.D.V. supervised and administered the project. N.G., M.A.H. and P.D.V. wrote the manuscript. All authors have revised and approved the manuscript.
Conflicts of interest
The authors declare no conflict of interest.
Acknowledgements
This study was financed by the PhD Scholarship framed in the Joint Degree Program (JDP) in Biomedical Sciences at The Faculty of Medicine of the Universidad de Chile (UCH) and the Graduate School of Medical Sciences of the University of Groningen (UG). N. G. was financially supported by the National Agency for Research and Development (ANID)/Scholarship Program/DOCTORADO BECAS NACIONAL/2020/PhD Internship (#21200669), and de Cock-Hadders Grant (#O/931506).
References
- S. Carding, K. Verbeke, D. T. Vipond, B. M. Corfe and L. J. Owen, Dysbiosis of the Gut Microbiota in Disease, Microb. Ecol. Health Dis., 2015, 26, 26191 Search PubMed eCollection2015.
- M. Beukema, M. M. Faas and P. de Vos, The Effects of Different Dietary Fiber Pectin Structures on the Gastrointestinal Immune Barrier: Impact via Gut Microbiota and Direct Effects on Immune Cells, Exp. Mol. Med., 2020, 52, 364–1376 Search PubMed.
- B. R. Thakur, R. K. Singh and A. K. Handa, Chemistry and Uses of Pectin - A Review, Crit. Rev. Food Sci. Nutr., 1997, 37, 47–73 CrossRef CAS PubMed.
-
A. Tanhatan and N. J. Thibault, Citrus Pectin: Structure and Application in Acid Dairy Drinks, Chemistry, Agricultural and Food Sciences, 2015, Special issue 1, pp. 60–70 Search PubMed.
- A. G. J. Voragen, G. J. Coenen, R. P. Verhoef and H. A. Schols, A. Pectin, a Versatile Polysaccharide Present in Plant Cell Walls, Struct. Chem., 2009, 20, 263–275 CrossRef CAS.
- N. M. Sahasrabudhe, M. Beukema, L. Tian, B. Troost, J. Scholte, E. Bruininx, G. Bruggeman, M. van den Berg, A. Scheurink, H. A. Schols, M. M. Faas and P. de Vos, Dietary Fiber Pectin Directly Blocks Toll-Like Receptor 2–1 and Prevents Doxorubicin-Induced Ileitis, Front. Immunol., 2018, 9, 383 CrossRef PubMed.
- E. Cario, Barrier-Protective Function of Intestinal Epithelial Toll-like Receptor 2, Mucosal Immunol., 2008, 1, S62–S66 CrossRef CAS PubMed.
- M. Beukema, É. Jermendi, H. A. Schols and P. de Vos, The Influence of Calcium on Pectin's Impact on TLR2 Signalling, Food Funct., 2020, 11, 7427–7432 RSC.
- M. Beukema, É. Jermendi, T. Koster, K. Kitaguchi, B. J. Haan, M. A. Berg, M. M. Faas, H. A. Schols and P. Vos, Attenuation of Doxorubicin–Induced Small Intestinal Mucositis by Pectins Is Dependent on Pectin's Methyl–Ester Number and Distribution, Mol. Nutr. Food Res., 2021, 65, 2100222 CrossRef CAS PubMed.
- A. Pituch-Zdanowska, A. Banaszkiewicz and P. Albrecht, The Role of Dietary Fibre in Inflammatory Bowel Disease, Gastroenterol. Rev., 2015, 3, 135–141 CrossRef PubMed.
- K. Ishisono, T. Yabe and K. Kitaguchi, Citrus Pectin Attenuates Endotoxin Shock via Suppression of Toll-like Receptor Signaling in Peyer's Patch Myeloid Cells, J. Nutr. Biochem., 2017, 50, 38–45 CrossRef CAS PubMed.
- M. Beukema, K. Ishisono, J. de Waard, M. M. Faas, P. de Vos and K. Kitaguchi, Pectin Limits Epithelial Barrier Disruption by Citrobacter Rodentium through Anti-Microbial Effects, Food Funct., 2021, 12, 881–891 RSC.
- M. Beukema, R. Akkerman, É. Jermendi, T. Koster, A. Laskewitz, C. Kong, H. A. Schols, M. M. Faas and P. Vos, Pectins That Structurally Differ in the Distribution of Methyl–Esters Attenuate Citrobacter Rodentium –Induced Colitis, Mol. Nutr. Food Res., 2021, 65, 2100346 CrossRef CAS PubMed.
- M. Beukema, É. Jermendi, M. M. P. Oerlemans, M. J. Logtenberg, R. Akkerman, R. An, M. A. van den Berg, E. G. Zoetendal, T. Koster, C. Kong, M. M. Faas, H. A. Schols and P. de Vos, The Level and Distribution of Methyl-Esters Influence the Impact of Pectin on Intestinal T Cells, Microbiota, and Ahr Activation, Carbohydr. Polym., 2022, 286, 119280 CrossRef CAS PubMed.
- M. Beukema, É. Jermendi, M. A. van den Berg, M. M. Faas, H. A. Schols and P. de Vos, The Impact of the Level and Distribution of Methyl-Esters of Pectins on TLR2–1 Dependent Anti-Inflammatory Responses, Carbohydr. Polym., 2021, 251, 117093 CrossRef CAS PubMed.
- H. K. Armstrong, M. Bording-Jorgensen, D. M. Santer, Z. Zhang, R. Valcheva, A. M. Rieger, J. Sung-Ho Kim, S. I. Dijk, R. Mahmood, O. Ogungbola, J. Jovel, F. Moreau, H. Gorman, R. Dickner, J. Jerasi, I. K. Mander, D. Lafleur, C. Cheng, A. Petrova, T.-L. Jeanson, A. Mason, C. M. Sergi, A. Levine, K. Chadee, D. Armstrong, S. Rauscher, C. N. Bernstein, M. W. Carroll, H. Q. Huynh, J. Walter, K. L. Madsen, L. A. Dieleman and E. Wine, Unfermented β-Fructan Fibers Fuel Inflammation in Select Inflammatory Bowel Disease Patients, Gastroenterology, 2023, 164, 228–240 CrossRef CAS PubMed.
- E. Candia, D. Díaz-Jiménez, P. Langjahr, L. E. Núñez, M. de la Fuente, N. Farfán, F. López-Kostner, M. Abedrapo, M. Alvarez-Lobos, G. Pinedo, C. J. Beltrán, C. González, M. J. González, R. Quera and M. A. Hermoso, Increased Production of Soluble TLR2 by Lamina Propria Mononuclear Cells from Ulcerative Colitis Patients, Immunobiology, 2012, 217, 634–642 CrossRef CAS PubMed.
- J. A. M. Harmsen, M. A. Kusters-van Someren and J. Visser, Cloning and Expression of a Second Aspergillus Niger Pectin Lyase Gene (pelA): Indications of a Pectin Lyase Gene Family in A. Niger, Curr. Genet., 1990, 18, 161–166 CrossRef CAS PubMed.
- P. J. H. Daas, K. Meyer-Hansen, H. A. Schols, G. A. De Ruiter and A. G. J. Voragen, Investigation of the Non-Esterified Galacturonic Acid Distribution in Pectin with Endopolygalacturonase, Carbohydr. Res., 1999, 318, 135–145 CrossRef CAS.
- H. N. Englyst and J. H. Cummings, Simplified Method for the Measurement of Total Non-Starch Polysaccharides by Gas-Liquid Chromatography of Constituent Sugars as Alditol Acetates, Analyst, 1984, 109, 937 RSC.
- N. Blumenkrantz and G. Asboe-Hansen, New Method for Quantitative Determination of Uronic Acids, Anal. Biochem., 1973, 54, 484–489 CrossRef CAS PubMed.
- H. A. Deckers, C. Olieman, F. M. Rombouts and W. Pilnik, Calibration and Application of High-Performance Size Exclusion Columns for Molecular Weight Distribution of Pectins, Carbohydr. Polym., 1986, 6, 361–378 CrossRef CAS.
- S. E. Broxterman and H. A. Schols, Interactions between Pectin and Cellulose in Primary Plant Cell Walls, Carbohydr. Polym., 2018, 192, 263–272 CrossRef CAS PubMed.
- M. M. H. Huisman, A. Oosterveld and H. A. Schols, Fast Determination of the Degree of Methyl Esterification of Pectins by Head-Space GC, Food Hydrocolloids, 2004, 18, 665–668 CrossRef CAS.
- É. Jermendi, C. Fernández-Lainez, M. Beukema, G. López-Velázquez, M. A. van den Berg, P. de Vos and H. A. Schols, TLR 2/1 Interaction of Pectin Depends on Its Chemical Structure and Conformation, Carbohydr. Polym., 2023, 303, 120444 CrossRef PubMed.
- L. Eckmann, H. C. Jung, C. Schürer-Maly, A. Panja, E. Morzycka-Wroblewska and M. F. Kagnoff, Differential Cytokine Expression by Human Intestinal Epithelial Cell Lines: Regulated Expression of Interleukin 8, Gastroenterology, 1993, 105, 1689–1697 CrossRef CAS PubMed.
- L. Mazzucchelli, C. Hauser, K. Zgraggen, H. Wagner, M. Hess, J. A. Laissue and C. Mueller, Expression of Interleukin-8 Gene in Inflammatory Bowel Disease Is Related to the Histological Grade of Active Inflammation, Am. J. Pathol., 1994, 144, 997–1007 CAS.
- E. Latorre, E. Layunta, L. Grasa, J. Pardo, S. García, A. I. Alcalde and J. E. Mesonero, Toll-like Receptors 2 and 4 Modulate Intestinal IL-10 Differently in Ileum and Colon, United Eur. Gastroenterol. J., 2018, 6, 446–453 CrossRef CAS PubMed.
- P. Langjahr, D. Díaz-Jiménez, M. De La Fuente, E. Rubio, D. Golenbock, F. C. Bronfman, R. Quera, M. J. G. Lez, M. A. Hermoso and C. F. Benjamim, Metalloproteinase-Dependent TLR2 Ectodomain Shedding Is Involved in Soluble Toll-like Receptor 2 (sTLR2) Production, PLoS One, 2014, 9, 1–20 CrossRef PubMed.
- E. Candia, D. Díaz-Jiménez, P. Langjahr, L. E. Núñez, M. de la Fuente, N. Farfán, F. López-Kostner, M. Abedrapo, M. Alvarez-Lobos, G. Pinedo, C. J. Beltrán, C. González, M.-J. González, R. Quera and M. A. Hermoso, Increased Production of Soluble TLR2 by Lamina Propria Mononuclear Cells from Ulcerative Colitis Patients, Immunobiology, 2012, 217, 634–642 CrossRef CAS PubMed.
- K. Ishisono, T. Yabe and K. Kitaguchi, Citrus Pectin Attenuates Endotoxin Shock via Suppression of Toll-like Receptor Signaling in Peyer's Patch Myeloid Cells, J. Nutr. Biochem., 2017, 50, 38–45 CrossRef CAS PubMed.
- H. Salman, M. Bergman, M. Djaldetti, J. Orlin and H. Bessler, Citrus Pectin Affects Cytokine Production by Human Peripheral Blood Mononuclear Cells, Biomed. Pharmacother., 2008, 62, 579–582 CrossRef CAS PubMed.
- B. Busato, E. C. De Almeida Abreu, C. L. De Oliveira Petkowicz, G. R. Martinez and G. Rodrigues Noleto, Pectin from Brassica Oleracea Var. Italica Triggers Immunomodulating Effects in Vivo, Int. J. Biol. Macromol., 2020, 161, 431–440 CrossRef CAS PubMed.
- K. Sasaki, Y. Kokai, S. Atsumi, H. Tobioka, N. Sawada, K. Hirata and M. Mori, Difference in the Expression of Three Tight Junction Proteins, Barmotin, Occludin, and ZO-1, in Phenotypically Different Human Colon Cancer Cell Lines, Med. Electron Microsc., 1998, 31, 61–67 CrossRef CAS.
- M. Pierik, S. Joossens, K. Van Steen, N. Van Schuerbeek, R. Vlietinck, P. Rutgeerts and S. Vermeire, Toll-like Receptor-1, -2, and -6 Polymorphisms Influence Disease Extension in Inflammatory Bowel Diseases, Inflamm. Bowel Dis., 2006, 12, 1–8 CrossRef PubMed.
- I. Manoharan, Y. Hong, A. Suryawanshi, M. L. Angus-Hill, Z. Sun, A. L. Mellor, D. H. Munn and S. Manicassamy, TLR2-Dependent Activation of β-Catenin Pathway in Dendritic Cells Induces Regulatory Responses and Attenuates Autoimmune Inflammation, J. Immunol., 2014, 193, 4203–4213 CrossRef CAS PubMed.
- C. R. Weber, S. C. Nalle, M. Tretiakova, D. T. Rubin and J. R. Turner, Claudin-1 and Claudin-2 Expression Is Elevated in Inflammatory Bowel Disease and May Contribute to Early Neoplastic Transformation, Lab. Invest., 2008, 88, 1110–1120 CrossRef CAS PubMed.
- J. Luettig, R. Rosenthal, C. Barmeyer and J. Schulzke, Claudin-2 as a Mediator of Leaky Gut Barrier during Intestinal Inflammation, Tissue Barriers, 2015, 3, e977176 CrossRef CAS PubMed.
- W. F. De Souza, N. Fortunato-Miranda, B. K. Robbs, W. M. De Araujo, J. C. de-Freitas-Junior, L. G. Bastos, J. P. B. Viola and J. A. Morgado-Díaz, Claudin-3 Overexpression Increases the Malignant Potential of Colorectal Cancer Cells: Roles of ERK1/2 and PI3K-Akt as Modulators of EGFR Signaling, PLoS One, 2013, 8, e74994 CrossRef CAS PubMed.
- X. Lin, X. Shang, G. Manorek and S. B. Howell, Regulation of the Epithelial-Mesenchymal Transition by Claudin-3 and Claudin-4, PLoS One, 2013, 8, e67496 CrossRef CAS PubMed.
- J. A. Eaden, Risk of Colorectal Cancer in Ulcerative Colitis: A Meta-Analysis, Gut, 2001, 48, 526–535 CrossRef CAS PubMed.
- E. Cario, G. Gerken and D. K. Podolsky, Toll-like Receptor 2 Enhances ZO-1-Associated Intestinal Epithelial Barrier Integrity via Protein Kinase C, Gastroenterology, 2004, 127, 224–238 CrossRef CAS PubMed.
- G. Melmed, L. S. Thomas, N. Lee, S. Y. Tesfay, K. Lukasek, K. S. Michelsen, Y. Zhou, B. Hu, M. Arditi and M. T. Abreu, Human Intestinal Epithelial Cells Are Broadly Unresponsive to Toll-Like Receptor 2-Dependent Bacterial Ligands: Implications for Host-Microbial Interactions in the Gut, J. Immunol., 2003, 170, 1406–1415 CrossRef CAS PubMed.
|
This journal is © The Royal Society of Chemistry 2024 |
Click here to see how this site uses Cookies. View our privacy policy here.