DOI:
10.1039/D4IM00003J
(Review Article)
Ind. Chem. Mater., 2024,
2, 378-392
Multi-component liquid-infused systems: a new approach to functional coatings
Received
9th January 2024
, Accepted 23rd February 2024
First published on 23rd February 2024
Abstract
Antifouling liquid-infused surfaces have generated interest in multiple fields due to their diverse applications in industry and medicine. In nearly all reports to date, the liquid component consists of only one chemical species. However, unlike traditional solid surfaces, the unique nature of liquid surfaces holds the potential for synergistic and even adaptive functionality simply by including additional elements in the liquid coating. In this work, we explore the concept of multi-component liquid-infused systems, in which the coating liquid consists of a primary liquid and a secondary component or components that provide additional functionality. For ease of understanding, we categorize recently reported multi-component liquid-infused surfaces according to the size of the secondary components: molecular scale, in which the secondary components are molecules; nanoscale, in which they are nanoparticles or their equivalent; and microscale, in which the additional components are micrometer size or above. We present examples at each scale, showing how introducing a secondary element into the liquid can result in synergistic effects, such as maintaining a pristine surface while actively modifying the surrounding environment, which are difficult to achieve in other surface treatments. The review highlights the diversity of fabrication methods and provides perspectives on future research directions. Introducing secondary components into the liquid matrix of liquid-infused surfaces is a promising strategy with significant potential to create a new class of multifunctional materials.
Keywords: Active surfaces; Antimicrobial; Antifouling; Interfaces; Sensing surfaces.
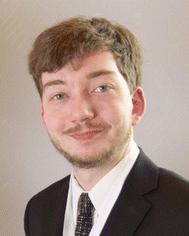 Zach Applebee | Zach Applebee is a PhD student in Biomedical Engineering in the Graduate School of Biomedical Science and Engineering at the University of Maine under the supervision of Associate Professor Caitlin Howell. His research interests include designing new and innovative surfaces to combat fouling as well as exploring new methods for drug transport and delivery. |
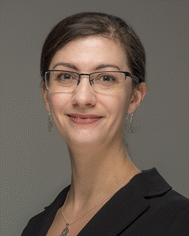 Caitlin Howell | Caitlin Howell is an Associate Professor of Bioengineering at the University of Maine and the leader of the Biointerface and Biomimetics Lab. Her research interests include the design and engineering of surfaces and interfaces for the control of biological systems, combining knowledge from biology, chemistry, physics, and materials science to create new solutions to a range of problems in both industry and medicine. |
1 Introduction
1.1 Liquid-infused surfaces
The addition of a liquid layer on the surface of a material to create a stable yet mobile coating is a relatively recent concept in materials science. These materials, referred to as liquid-infused surfaces (LIS),1–3 slippery liquid-infused porous surfaces (SLIPS),4–7 or infused polymers,8–11 depending on their particular form, leverage the fluid characteristics of liquids to attain unique properties that are difficult to achieve with solid materials, such as ultra-low adhesion,12,13 self-healing,4,14,15 and even self-replenishment.9 Several excellent reviews are available that cover the fundamental physics of liquid-infused surfaces in depth.16,17 However, to briefly orient the reader: in general, liquid-infused surfaces consist of a solid component that serves as the base and a liquid component that coats or otherwise covers the base.18 In the case of infused polymers, the liquid component also penetrates through the base.19 The surface of the solid component can vary in texture from flat to nano- or micro-scale roughness to help hold the liquid in place; however, with a few exceptions,20 it must have a surface chemistry that permits complete wetting of the infusing liquid and makes it energetically unfavorable for the infusing liquid to be displaced by a contaminant. Beyond these fundamental rules, there are few limits on what can be used as a solid or a coating liquid as long as insolubility with the desired contaminants and stability in the surrounding environment are considered.21
The large variety of potential liquid–solid combinations,2,16,18,21,22 and the demonstrated effectiveness in various challenging environmental conditions and against difficult contaminants,23–25 at least partially explains the increasing use of liquid-infused surfaces. Multiple studies have demonstrated the ability of various types of liquid–solid pairs to resist adhesion by bacterial and fungal pathogens successfully.8,26,27 Other works have shown the ability of liquid-infused surfaces to resist the adhesion of crude oil and ice,28–30 as well as sticky proteins such as fibrinogen and whey.31 Yet others have demonstrated that liquid coatings can effectively mitigate mineral fouling and prevent corrosion.32–35 Perhaps most importantly, the anti-fouling functionality of these coatings has been shown to persist even when challenged with highly complex foulants such as blood36–38 or when placed in highly complex settings such as the marine environment39–41 and within living systems (in vivo).23,36,42 The continued success of using liquids as coatings suggests that this strategy may be uniquely suited to solving problems that are difficult to address with solids alone.
However, it is notable that reports on liquid-infused surfaces in the literature overwhelmingly use a liquid component consisting of only a single chemical species. Although this strategy successfully achieves the primary technical aims in most reports—most often adhesion resistance—there is even further opportunity that has yet to be fully developed. Liquid-infused surfaces are unique in that they are mobile and dynamic in a way that solid surfaces can never be, opening the door to the potential of easily having multiple functionalities built into the same system. Integrating a secondary component or components into the liquid can achieve additive and even synergistic functionality. This review discusses how multi-component liquid coatings are being leveraged to enhance the utility of liquid-infused surfaces across a variety of applications. We highlight examples of molecular-scale secondary components, such as nitric oxide (NO), being added to liquids into liquid-infused catheters to reduce bacterial and platelet adhesion beyond what is achievable with a liquid coating alone. We discuss nanoscale secondary components such as nanobubbles, which enhance surface slip, and magnetic nanoparticles, which permit active control over the form of the liquid coating. We also explore applications of multi-component liquid coatings at the micro-scale, for example, adding defined regions of a hydrophilic liquid into a coating made primarily of a hydrophobic liquid to create a microarray. Various fabrication methods to produce multi-component liquid coatings are outlined beyond the standard mixing of a secondary component into a primary liquid, expanding the design space that can be used. Finally, we provide an overview of the field and identify promising future directions. Further leveraging the unique nature of liquid-infused surfaces by incorporating multiple components can yield some truly novel and paradigm-shifting surface strategies across a range of applications.
1.2 Multi-component liquid-infused surfaces
Here, we define a multi-component liquid-infused surface as a liquid-infused surface in which the coating liquid contains more than one substance. The secondary substance (or substances) can be either molecules or particles dispersed throughout the primary liquid or discreet volumes of a secondary component, such as a second liquid type, interspersed throughout the primary liquid (Fig. 1). The presence of the secondary substance may be a permanent feature or transient in nature, located within the liquid bulk or organized at either the solid/liquid or liquid/air interfaces; however, it must be chemically distinct from the primary liquid and not covalently attached to the solid. The secondary component may come from direct addition to the primary liquid at the fabrication time, or it may slowly disperse into the liquid from either the bulk or the external environment over time. In the following sections, we present specific examples of each of these multi-component systems and an overview of how the multi-component liquid strategy has already been implemented across a range of scales and for various applications.
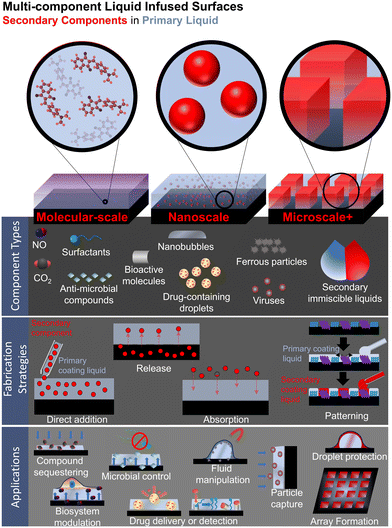 |
| Fig. 1 A schematic overview of this review. An illustration of multi-component liquid-infused surfaces with the secondary component at three different length scales: molecular scale, in which the secondary component is a molecule or group of molecules (left); nanoscale, in which the secondary component is a nanoparticle or other nanoscale entity (center); and microscale, where the secondary component is organized into microscale (or larger) discreet volumes within the primary liquid coating. The three rows underneath show examples of the secondary component types, fabrication strategies, and select applications at each scale. | |
2 Molecular-scale
Molecules are perhaps the easiest substances to incorporate within a coating liquid. For that reason, to date, most reports involving multi-component liquid-infused surfaces have secondary components at this scale. Although the specific molecules range from those that pass into or come from the environment to larger, more highly targeted bioactive compounds, nearly all take advantage of diffusion within the primary liquid to transport the active secondary component to the site where the latter can perform its function.
2.1 Secondary component: nitric oxide
Nitric oxide (NO) is a bioactive molecule with anti-inflammatory properties and plays a crucial role in diverse physiological processes, including neurotransmission, platelet adhesion, and wound healing.43,44 NO molecules are produced by endothelial cells to modulate the immune response45 and specific immune cells to disrupt microbial growth and proliferation.46 Mimicking this natural NO release is of interest in fabricating biomedical materials as a strategy to reduce inflammation and enhance antimicrobial properties without the danger of enhancing antimicrobial resistance.47,48 As NO is a gas under ambient temperature and pressure, it must be incorporated into materials via molecules that will release NO over time under physiological conditions. One such compound, S-nitroso-acetyl penicillamine (SNAP), has been infused directly into silicone rubber tubing, which then released NO at physiologically relevant concentrations for at least four hours.49 Handa and colleagues first combined this concept with liquid-infused surfaces by impregnating silicone tubing with SNAP, then infusing it with silicone oil to create a liquid-infused surface (Fig. 2a).50 Their goal was to create an easily manufactured material that would not only release NO but also prevent one of the most significant problems facing NO-doped materials: that fouling of the surface with proteins and other biological materials would mask the interface, slowing and eventually preventing NO release.51,52 Using a multi-component liquid-infused system to address this was successful. NO generated by both the leaching and degradation of the SNAP within the silicone solid slowly infused into the silicone liquid, releasing NO at consistent rates (0.35–0.42 × 10−10 mol cm−2 min−2) over seven days and significantly reducing platelet adhesion compared to surfaces with a liquid coating alone. Further work confirmed the increased consistency of the NO release rate over 60 days compared to controls53 and also showed that the rate could be modulated by changing SNAP infusion levels54 or using an alternative donor compound.52 Tests of NO-releasing liquid-infused materials in vivo demonstrated how this multi-component system could also reduce the encapsulation thickness of subcutaneous implants in a 21 day mouse model compared to a liquid-infused control without NO.55
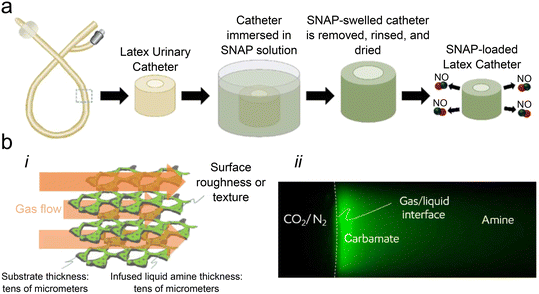 |
| Fig. 2 (a) Schematic showing latex catheters being infused with the NO donor S-nitroso-N-acetyl-penicillamine, adapted with permission from ref. 53, copyright 2022, American Chemical Society; (b) i: schematic showing how gas flows over the infused liquid amine to allow for the capture of CO2, ii: fluorescent image of the gas/liquid interface after a 28 min exposure to CO2/N2 (1 : 1) at 35 °C. Reproduced with permission from ref. 66, copyright 2022, Science Advances. | |
Research on including NO-releasing compounds into liquid coatings has demonstrated the synergy that can be achieved by incorporating bioactive gases into liquid-infused surfaces. Building off this, more can now be done to expand the applications of the approach even further. For example, other gases such as carbon monoxide (CO), which can modulate cell signaling, or hydrogen sulfide (H2S), which functions as a signaling molecule in multiple systems in the human body,56,57 could be incorporated. As with NO, the liquid layer in CO- or H2S-releasing systems could serve as both the means to keep the surface from being masked by biological molecules and the method of controlling the rate of diffusion of the bioactive gas out into the body. Further work with NO could explore the capacity to release on-demand, leveraging the ability of SNAP to respond to triggers such as thermal decomposition,58 ionic catalysis,59 or exposure to light.60 Finally, exploration of the effects of other NO donors, such as diazeniumdiolates and nitrosothiols, which have been synthesized and characterized for their capacity to release NO effectively,61,62 could provide further ways of modulating the NO release rate.
2.2 Secondary component: carbon dioxide
With the rising concerns around climate change, much effort has been put into developing new ways of extracting carbon dioxide (CO2) from the air and sequestering it long-term.63–65 Recent work has explored the ability of highly porous solids coated in reactive liquids to increase the synergistic capture of CO2 from the air. Yeganeh and colleagues66 used additive manufacturing to create highly porous metallic hexagonal structures, then coated them with liquid amines—compounds currently used commercially to capture CO2 emitted from power plants.67 The mechanism was simple: when a CO2/N2 air mixture was passed through the liquid-coated metallic framework, the CO2 reacted with the amine liquid to form carbamate sequestered within the liquid, creating a multi-component liquid-infused system (Fig. 2b). Tests with this system showed that the uptake of CO2 by the micrometer-thin liquid layer was comparable to other solid materials, such as carbon molecular sieves and metal–organic frameworks, with no adverse effects in the presence of water vapor. Furthermore, the system was found to perform better at higher temperatures, in contrast to other amine-impregnated or -grafted materials, due to the ability of the carbamate to diffuse away from the surface deeper into the liquid coating. Importantly, diffusion away from the surface effectively allowed the entire bulk of the reactive amine liquid to be used in the capture process instead of only a surface layer, increasing the filter's lifetime.
The use of liquid layers to capture CO2 demonstrates the unique use of a multi-component liquid-infused system, which may find utility elsewhere. Pollution of both air and water is of growing concern around the world,68–72 and the use of high surface-area liquid coatings as capture mediators may offer benefits over traditional solid filters, such as reduced clogging, increased use time, greater capture capacity, or reduced power requirements. Future work on multi-component liquid-infused surfaces in this direction could greatly benefit our efforts to achieve a more sustainable future.
2.3 Secondary component: antimicrobial compounds
The continuous and increasing development of antimicrobial resistance is one of the most significant challenges facing global public health.73,74 Although many different strategies are likely required to combat this problem, developing materials that can prevent microbial attachment to surfaces—effectively stopping microbial colonization and growth before it starts—is a promising approach.74 However, surface-based antimicrobial strategies often fail in complex environments due to the tendency of the surfaces to become covered with contaminants such as proteins and other complex molecules, creating a masking layer that limits the functionality of the surface. Concerningly, when masking occurs, sub-lethal doses of antimicrobial compounds can be released, further promoting the development of antimicrobial resistance.75 Liquid-infused surfaces have been shown to prevent this layer from forming by preventing the adhesion of protein and other biological molecules.42 Some work has taken this one step further, incorporating antimicrobial compounds into the liquid layer to create a synergistic multi-component system that can influence microbial growth on the surface and in the surrounding environment while remaining free of a masking layer.
Triclosan is a synthetic, broad-spectrum antimicrobial compound that was first introduced in the 1960s and subsequently incorporated into a wide range of consumer products due to its ability to kill both bacterial and fungal cells and its solubility in oils.76,77 Lynn et al. explored the ability of this compound to be incorporated into silicone liquid-infused polymers to create a multi-component system that could both resist microbial adhesion to the surface and kill organisms in the surrounding environment.19 The multi-component liquid coatings were created by fabricating a porous multilayer on the surface of test materials,78 followed by loading the triclosan dissolved in acetone onto the multilayer. Once the acetone had evaporated and the surface was dried, the porous surface was coated with silicone oil. When put into use, the triclosan diffused into the silicone oil and out into the environment, significantly reducing the metabolic activity of the yeast Candida albicans both on the material surface and in the surrounding medium over 24 hours compared to controls without triclosan. Although triclosan itself is being phased out of use in consumer products due to concerns over safety and contamination of aquatic environments,79 the results from this study demonstrate how the incorporation of an antimicrobial compound into a liquid surface coating can provide a system in which multiple mechanisms are used (e.g., adhesion resistance and antimicrobial functionality mediated by diffusion) to achieve a common goal.
2.4 Secondary component: microbial quorum-sensing inhibitors
One response to the rise in antimicrobial resistance has been identifying or developing compounds that influence microbial growth and behavior without killing cells. Quorum sensing inhibitors (QSIs) are one such class of compounds that prevent communication among bacterial cells, diminish the pathogenicity of bacteria, and reduce the rate of biofilm formation on surfaces.80,81 After demonstrating the potential of creating multi-component systems using triclosan, Lynn and colleagues further used their porous multilayer coating approach to load QSIs into materials with liquid layers.19,82 As with triclosan, the QSIs were immobilized onto the porous solid and then coated with silicone oil. The compounds then diffused from the solid, through the liquid, and out into the environment where they affected the bacterium Pseudomonas aeruginosa both on the surface and in the surrounding medium (Fig. 3ai). Notably, the samples were loaded with multiple different QSIs to target several quorum sensing pathways simultaneously. Each type of QSI was found to diffuse out of the liquid at a different rate, most likely due to differences in the interactions of the compounds with both the porous surface and the liquid coating. While the presence of QSIs did not significantly change the amount of biofilm present on the surface compared to a similar liquid-infused surface without QSIs, it did significantly decrease the amount of biofilm adhered to the surrounding surfaces, demonstrating the capacity of these multi-component systems to influence the environment around the material (Fig. 3aii).
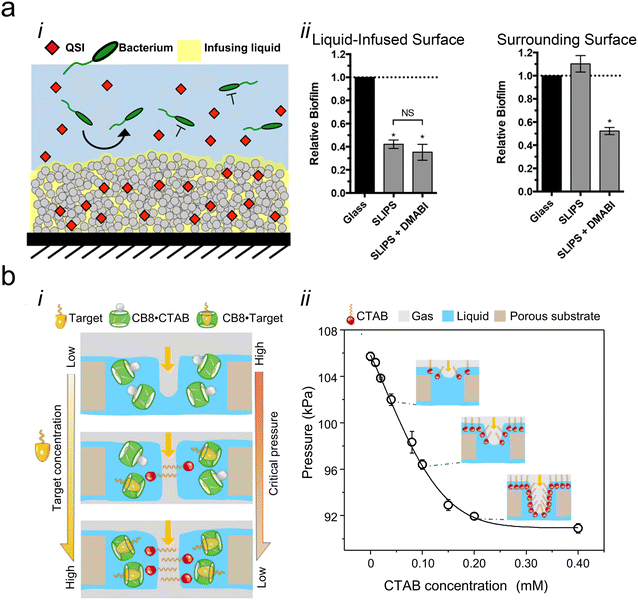 |
| Fig. 3 (a) i: Schematic showing how loaded slippery liquid-infused porous surfaces (SLIPS) gradually release quorum sensing inhibitors (QSI) into the infusing liquid to prevent bacterial colonization, ii: graph showing the relative biofilm formation on a QSI-loaded liquid-infused surface and the surrounding surface, adapted with permission from ref. 82, copyright 2016, American Chemical Society; (b) i: illustration showing the gating behavior of the target molecules and how different concentrations of the target change the critical gas pressure, ii: graph showing how the critical pressure of the gas changes with cetyltrimethylammonium bromide (CTAB) concentration, adapted with permission from ref. 83, copyright 2022, Springer Nature. | |
2.5 Secondary components: surface-tension-modulating molecules
Although the field of multicomponent liquid-infused systems is still in its infancy, more complex examples that demonstrate the power of this approach are already beginning to emerge. One example is a liquid-infused system developed by Hou and colleagues, in which a secondary component is used to adaptively change the surface tension of the liquid coating.83 The system has been used to provide a means of visually assessing the amount of a target molecule present in the coating liquid. Briefly, the system relies on a liquid-gating mechanism in which a liquid coats a porous membrane. When pressure is applied, the gating liquid is displaced, and the air (or other fluid) moves through the membrane.84 In the molecular-scale multi-component version of this system, the aqueous coating liquid contains a host molecule coupled to a surfactant. When a target molecule is present, it will displace the surfactant from the host molecule, driving the surfactant to the surface of the coating liquid, changing the surface tension, and making it easier for a gas to flow through the membrane (Fig. 3bi). An increase in surfactant concentration from 0–0.40 mM decreased the pressure needed to displace the liquid coating from 106 kPa to a minimum of ∼91 kPa (Fig. 3bii), which could be correlated to both the concentration of the target molecule as well as the visible color of the bulk solution.
In an alternative version of the multi-component adaptive pressure-sensitive system, the molecular photoswitch azobenzene was added to a perfluorinated coating liquid and used to coat a porous membrane to create a liquid-gated system.85 When the system was exposed to a particular wavelength of light, the headgroup of the azobenzene would change from a cis- to a trans-isomer, significantly reducing the pressure required to displace the liquid from the pore. Hou and team demonstrated how this approach could be used to control the specific location at which a fluid could flow through a liquid-gated membrane.
2.6 Outlook on molecular-scale components
The addition of molecular-scale components to liquid-infused surfaces can be a relatively simple way to create a multi-functional or responsive surface. While applications such as influencing a biological system (e.g., stopping or encouraging the growth of cells) or chemical system (e.g., changing properties upon exposure to a particular chemical) may be among the ones that most immediately come to mind, expansion of this concept into other more out-of-the-box applications has the potential to lead to new paradigms in materials science. Taking advantage of the unique properties of liquids to permit diffusion or flow of molecules as well as change their shape could be leveraged to develop new types of surfaces that respond in ways that would be impossible to achieve with solids. The wide variety of molecules that can function in different ways, combined with the ability to make liquid-infused surfaces with nearly any liquid, further opens doors for creativity and innovation.
3 Nanoscale
Although most reports on multi-component liquid-infused surfaces use molecular-scale compounds as the secondary component, many have also explored the use of nano-scale compounds. Nanoscale compounds offer a unique set of properties to liquid-infused systems while also being small enough to take advantage of the increased diffusion and mobility that liquid coatings provide, leading to some powerful synergistic effects.
3.1 Secondary component: nanoemulsions
Solubility in oil is common among nearly all secondary components in multi-component liquid-infused systems due to the ease with which oil-soluble compounds can be incorporated into oil-based coating liquids. To date, oils are most often used in coating liquids due to the fact that most applications of liquid-infused surfaces involve resisting fouling by water-soluble contaminants. However, many bioactive or otherwise interesting potential secondary components are not soluble in oils. Recognizing this, Lynn and co-workers developed a method to incorporate water-soluble compounds into oil-based liquid coatings by creating nanoemulsions of water in oil.86 As a proof of concept, a surfactant mixture in n-hexadecane was added to water either with or without water-soluble compounds such as fluorescein isothiocyanate (FITC)-dextran to create a water-in-oil nanoemulsion with an average particle diameter of ∼134 ± 20 nm. The emulsion (or n-hexadecane alone as a control) was then coated on a polytetrafluoroethylene (PTFE) membrane to create a nanoscale multi-component liquid-infused surface (Fig. 4a). Test of systems loaded with FITC-dextran showed a continuous release of the fluorinated compound at rates between ∼0.1 and ∼0.004 μg cm−2 over 45 days, with 80% of the compound released from the samples over 90 days.
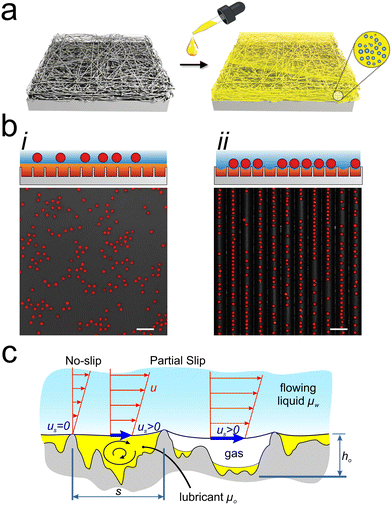 |
| Fig. 4 (a) Schematic showing how a water/oil nanoemulsion (yellow) can be infused into a porous PTFE membrane (gray) to create slippery nanoemulsion-infused porous surface, reproduced with permission from ref. 86, copyright 2021, Springer Nature; (b) schematic showing fluorescent microparticles on an un-actuated (i) and actuated (ii) ferrofluid surface illustrating how the particles become confined when the ferrofluid has been depleted using a magnet, reproduced with permission from ref. 99, copyright 2018, Springer Nature; (c) schematic showing how nanobubbles increase slip of a fluid flowing over a liquid layer, reproduced with permission from ref. 110, copyright 2022, Springer Nature. | |
3.2 Secondary component: magnetic nanoparticles
Perhaps the most well-known multi-component liquid-infused systems involve the use of ferrofluids, or stable suspensions of nanometer-sized ferromagnetic particles in a base fluid,87 as the coating liquid. Initially introduced in the 1960s for applications in rocket engines operating in microgravity,88 ferrofluids have found utility in applications ranging from dynamic loudspeakers89 to the treatment of tumor cells90,91 and more.92–94 Varanasi and colleagues were the first to report the use of an oil-based ferrofluid as a liquid coating on a textured surface.95 The resulting magnetically actuated surface allowed them to direct the movement and control the shape of water droplets on the surface with only the relatively small magnetic field generated by permanent magnets. Further work on droplet control using ferrofluid liquid-infused surfaces demonstrated how the tendency of the ferrofluid coating to create ridges around base aqueous droplets could be used to control droplets of a range of different challenging materials, including fetal bovine serum and dried cells of E. coli.96 In addition to aqueous droplets, ferrofluid multi-component systems have also been used to control the precise movement of gas bubbles.97 In another application, ferrofluid coatings were found to increase the energy barrier for ice nucleation on a surface by creating a reversible, textured liquid–liquid interface between a water drop and the ferrofluid.98 All of these examples are steps toward more efficient and complex manipulation of droplets on surfaces,27 which can open new doors in the fields of biosensing, micro-fabrication, and droplet computing.93
Beyond fluid droplet control, Aizenberg et al. demonstrated how a combination of well-defined surface textures and ferrofluid coatings could be used to reversibly organize microparticles at interfaces. Briefly, by reducing the thickness of the ferrofluid at the surface through the introduction of a magnetic field, they could reveal an underlying surface texture, which would push disorganized particles into a defined arrangement, including into straight lines (Fig. 4b) or groups of a particular size.99 In both this and the droplet/bubble manipulation applications mentioned previously, the deformable nature of the liquid coating the surface, combined with its return to an equilibrium in close association with the surface, are what permit the secondary component—the magnetic nanoparticles—to function as an adaptive coating. One promising direction for future work may be in exploring the incorporation of nanoparticles that respond to stimuli other than magnetic fields, such as light (e.g., liposomes),100 heat (e.g., polycaprolactone[N-isopropyl acrylamide] nanoparticles),101 or ultrasound (e.g., mesoporous silica nanoparticles [MSNPs]).102 The use of other types of responsive nanoparticles may reveal additional synergistic effects that can occur in nano-scale multicomponent liquid-infused systems.
3.3 Secondary component: fluorescent nanoparticles
Visualization and quantification of the liquid coating in liquid-infused systems can be challenging, particularly in systems that use omniphobic fluorinated liquids such as perfluoropolyethers (PFPEs). PFPEs are excellent at resisting both hydrophobic and hydrophilic contaminants but are unfortunately also resistant to the incorporation of nearly all dyes that could be used to facilitate visualization. To overcome this limitation, calcium carbonate (CaCO3) nanoparticles, fluorinated and labeled with a fluorescent molecule, have been added as a secondary component that allows for the visualization of fluorinated liquid layers.103 Briefly, CaCO3 particles approximately 70 nm across with surface carboxyl groups were incubated with a fluorophore (rhodamine B), which created an ionic bond between the two. The labeled particles were then extracted into a fluorinated surfactant with a carboxylic acid group present on its terminal fluoromethane.104 The labeled nanoparticles in the fluorinated surfactant were then added to either PFPE or perfluorodecalin coating liquids used to coat solid surfaces. The nanoparticles dispersed evenly throughout the liquid, allowing clear visualization of the thickness of the liquid coating undergoing shear stress.
3.4 Secondary component: virus-containing aerosols
Viruses, non-living entities that range from tens to hundreds of nanometers, have an exceptional capacity to disrupt human lives. When airborne, they can be easily spread but difficult to detect.105 In 2021, Regan and colleagues106 reported on a liquid-infused HEPA membrane system designed to capture and sequester viruses from virus-containing aerosols filtered from large volumes of air. They hypothesized that the presence of the liquid coating would make it easier to recover and analyze the viruses in the aerosols to provide more information on the types and, importantly, the infectiousness of these pathogens. In a series of tests, commercial HEPA filters were coated with PFPE fluids and then used to filter infectious virus-containing aerosolized droplets with sizes ranging from 60 to 140 nm. Upon filtration, the viruses became at least partially incorporated into the liquid coatings, creating a multi-component liquid-infused surface in which the coating liquid could be dissolved off the HEPA filters and the viral particles contained within it quantified. Interestingly, the liquid coating was found to affect different types of viruses differently. In enveloped SARS-CoV-2, the results suggested that the PFPE fluid dissolved the envelope and spilled the viral RNA, increasing the amount of RNA recovered with the liquid but decreasing the amount of infectious virus. In a non-enveloped polyomavirus, the opposite appeared to occur: the viral particles remained intact, increasing the amount of infectious virus recovered from the coated filters compared to uncoated controls. The results suggest that the use of liquid coating layers to capture and sequester biological entities is possible. Furthermore, properly selecting a coating liquid could produce effects that target only one type or class of biological entity, such as being more reactive to one type of virus than another.
3.5 Secondary component: surface nanobubbles
Surface nanobubbles are gas-filled pockets that are known to form at solid/liquid interfaces where surface roughness is present. Generally between 10 and 100 nm in height, nanobubbles form when the liquid component is flowing, and gas from within it spontaneously nucleates at the interface.107–109 However, surface nanobubbles were thought to exist primarily at solid/liquid interfaces until Neto et al. reported their detection in wrinkled Teflon/silicone oil liquid-infused surfaces in 2022.110 They reported that the nanobubbles on the liquid-infused surfaces formed as pockets, often over the oil layer111 (Fig. 4c) and were responsible for the 50× higher-than-expected slip length observed when water flowed over the infused surface. Although initially an unintentional multi-component liquid-infused surface, intentionally designed and fabricated surface nanobubble-containing liquid layers may prove useful in even further reducing the slip length of flowing fluid and finding applications in new energy-saving techniques. Alternatively, storing bioactive or other reactive gases in nanobubbles for slow release into the coating liquid over time may be useful as a new drug-delivery technique, particularly for those gases that dissolve in coating liquids only at very low concentrations.
3.6 Outlook on nanoscale components
While adding nanoscale components to liquid-infused surfaces can be slightly more difficult and involved than adding molecular-scale components, they can offer benefits beyond what can be achieved by adding molecules. Nanoscale materials are known for their unique properties derived from their large surface area, ability to self-assemble, and/or quantum effects that can result in altered electrical or optical behavior. When combined with a liquid matrix that permits particle mobility, these properties could potentially be enhanced or targeted in ways yet to be explored.
4 Microscale+
Reports of the integration of secondary components larger than nanoscale are notably fewer, likely due to the fact that most liquid layers are themselves tens of micrometers in thickness at most.2,16,21,112 Nevertheless, there are several interesting studies in which incorporating a larger secondary component has proven useful. Importantly, in this section, we define a microscale+ component as something other than the primary liquid that is dispersed throughout the primary liquid and not covalently bound to the solid substrate. Although this type of multi-component system can take the form of larger particles dispersed randomly in a liquid, it also encompasses defined surface patterning of the primary liquid together with a second (or even third) immiscible liquid, resulting in microscale or larger features on a surface.
4.1 Secondary component: magnetic microparticles
The on-demand release of drugs from devices is an active area of research with applications in cancer treatment,21 tissue engineering,113–115 and chronic disease management.116 Hou et al.97,117 used a micro-scale multi-component system to achieve an on-demand drug release effect, which leveraged the ability of the liquid coating to reversibly deform while remaining associated with the solid substrate. The system made use of microscale magnetic colloids, which, when added as a secondary component to a non-magnetic fluid coating a membrane, resulted in a magnetically actuated liquid-gated system (Fig. 5a). Briefly, when a magnetic field was turned on, the ∼2.3 μm-diameter magnetic microparticles within the coating liquid filling the membrane pores ordered themselves orthogonally to the direction of flow. The combination of the coating fluid and the microparticles provided enough resistance to flow to block a drug-containing fluid from passing through the pore. When the field was removed, the particles became disordered, and the liquid containing them was more easily displaced, allowing the drug-containing fluid to pass through. In this multi-component liquid-infused system, the larger size of the secondary component was critical in stabilizing the coating liquid.
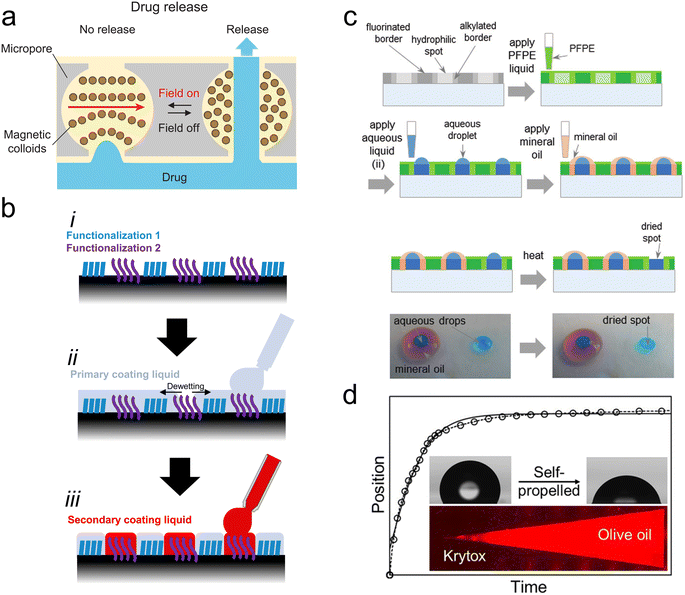 |
| Fig. 5 (a) Schematic showing the mechanism of drug release in a liquid-gated membrane with magnetic microparticles as a secondary component, reproduced with permission from ref. 117, copyright 2020, Oxford University Press; (b) schematic showing a manufacturing process of multicomponent liquid-infused surfaces consisting of two distinct liquids. i: Initially, the surface undergoes dual-functionalization with two distinct surface treatments. ii: Subsequently, a primary coating liquid is introduced, permeating the surface. iii: Finally, a secondary coating liquid with a greater affinity for one of the functionalized areas is applied, displacing the initial liquid; (c) (top) schematic showing the formation of a three-liquid liquid system via surface patterning; (bottom) image of a three-liquid system protecting a blue droplet from desiccation, reproduced with permission from ref. 122, copyright 2018, Advanced Materials Interfaces; (d) plot of the position of a droplet vs. time as it moves along a two-liquid pattern. Inset: image showing the change in droplet contact angle and patterning of the infused system that allows for self-propulsion of the droplet, reproduced with permission from ref. 123, copyright 2023, American Chemical Society. | |
4.2 Secondary component: immiscible liquids
In nearly all multi-component liquid-infused systems, the volume or concentration of the secondary component is much less than that of the primary liquid. However, there is one class of liquid-infused surfaces in which the secondary component can be equal to or even greater than the volume of the primary liquid: liquid–liquid patterns or arrays, in which the primary liquid serves as a barrier around the secondary liquid. Patterned liquid surfaces are generally fabricated by first applying a surface functionalization that matches one of the liquids, then masking the surface and converting the exposed area into a functionalization that matches the other liquid. The surface is coated with the first liquid, which then either dewets or is displaced when the second liquid is added in the areas that do not have matching functionalization (Fig. 5b).118
The first reports of patterning hydrophobic and hydrophilic liquids together on a surface involved creating simple shapes such as macroscale letters, demonstrating how using an immiscible liquid with high affinity for the surface made it possible to use the same sample to quickly generate a pattern using usually difficult-to-control secondary liquids such as octane and blood.118 Further development of the concept showed how precise patterning could be used to create microarrays, in which tiny volumes of liquid are used to carry out biological or chemical tests in a rapid, high-throughput format.119,120 Levkin and co-workers121 patterned a hydrophobic PFPE primary liquid with an aqueous solution and used it to demonstrate biofilm morphology differences among three strains of the bacterium Pseudomonas aeruginosa. They went on to demonstrate how their approach to creating liquid–liquid multi-component coatings could be used to fabricate patterns of arbitrary shapes using PFPE liquid and a variety of secondary liquids, achieving precision down to 50 μm (Fig. 5c).122 Importantly, in this work, they also demonstrated how patterning three separate liquids—PFPE, mineral oil, and an aqueous sample—could be used to create an array in which the PFPE liquid separated the aqueous samples and the mineral oil created a protective wrapping layer over the droplet which prevented desiccation.
Multiple liquids with differing surface tensions have also been leveraged to create surfaces on which droplets spontaneously move in a pre-defined direction. By creating a multi-component liquid-infused surface in which the surface liquid consisted of both a PFPE liquid and olive oil organized into macro-scale, wedge-shaped patterns (Fig. 5d), Kusumaatmaja et al.123 were able to demonstrate how droplets placed on the surface would spontaneously move from areas of lower wettability (PFPE-coated surface) to higher wettability (olive oil-coated surface). Such movement was made possible by the ultra-low-friction surface presented by the patterned liquids and would have been difficult to achieve on a solid surface.
4.3 Outlook on microscale+ components
The integration of either solids or immiscible liquids in microscale quantities into liquid coatings can be more challenging due to the thin nature of liquid coatings. However, the reports summarized here show that with proper design and fabrication, larger-scale secondary components integrated into liquid-infused systems can yield materials with new properties and diverse applications. Future work taking advantage of the natural ability of immiscible liquids to maintain boundaries while under flow or the ability of microparticles to assemble or disassemble under the right conditions as part of liquid coatings might open new doors in the application of microscale+ multi-component systems.
5 Conclusions, challenges, and future directions
Liquid-infused systems are widespread and versatile, leveraging the unique properties of liquids to provide surface functionality that goes beyond the capacity of traditional solid surface coatings. Multi-component liquid-infused systems are a growing sub-category of liquid-infused systems in which the liquid component consists of a primary infusing liquid and a secondary component incorporated within that liquid. In this review, we have assembled a wide variety of examples that demonstrate how adding a secondary component to a coating liquid can generate unique and synergistic systems across a range of applications. Secondary components such as NO, antibiotics, and bacterial QSIs slowly diffuse from the solid into the liquid, then the surrounding environment, maintaining a surface free from protein or bacterial fouling due to the slippery nature of the primary liquid while also providing a more predictable and consistent compound release rate as the surface is not becoming more heavily fouled with time. Active surfaces such as these can also potentially benefit from the inherent self-healing properties of liquid layers, returning to their initial form and level of activity after damage. In contrast, multi-component systems can be created when molecules such as CO2 or particles such as viruses are captured out of the air and sequestered within the liquid. More complex systems that involve multiple molecular-scale additives interacting synergistically with each other or the liquid to alter the characteristics of the overall coating, such as lowering the surface tension, changing the liquid shape via a magnetic field, or changing the properties by patterning the liquid, highlight the potential of a multi-component approach. Building on these early innovations could now expand the applications of multi-component systems. Further work exploring what can be achieved with new secondary components, or multiple secondary components that work together, is likely to yield both new insight and new approaches that leverage liquid coatings, facilitating the creation of unique and versatile adaptive surfaces.
While multi-component liquid-infused surfaces hold a great deal of potential to expand how we think about and use surfaces, they are also faced with several important limitations that should be taken into consideration. First, the thickness of the liquid coating in liquid-infused surfaces most often ranges from hundreds of nanometers to tens of micrometers.2 Such a thin layer is instrumental in keeping the liquid associated with the solid surface rather than flowing away; however, this thinness (and therefore, low volume) can also limit the quantity of a secondary component that can be present within the liquid at the surface. Strategies such as incorporating a vascular network into the solid to use as a means to deliver additional liquid and/or secondary components to124 or from125 the surface may help to mitigate this issue; however, designers of multi-component liquid-infused surfaces should be aware of this limitation and plan accordingly. Another limitation is the solubility and/or stability of the secondary components within the liquid. When designing liquid-infused surfaces, liquids are chosen that will be immiscible to the contaminants the designers wish to resist. The most common combinations are oil-based liquids, which resist water-based contaminants, or omniphobic liquids, which resist both oil- and water-based contaminants. In designing multi-component liquid-infused systems, this feature can be useful in that secondary components that are soluble in the coating liquid are unlikely to migrate to the contaminating liquid. However, this can be a problem if the goal of the secondary component is to migrate out of the coating to have an effect in the surrounding environment. Tailored approaches, such as creating emulsions86 which can release a compound at the interface, may be one solution to this issue; the development of other strategies would likely enable wider applications, particularly in the area of synergistic antifouling and drug delivery. Awareness of both the challenge of coating thickness and component solubility and stability, as well as the development of new approaches to mitigate these limitations, will help to facilitate innovation in applications of multi-component liquid-infused surfaces.
Conflicts of interest
The authors declare no conflicts of interest.
Acknowledgements
The authors thank Vinal Applebee, Adrian Arias Palomo, Liza White, Sandro Zier, Evan Leonard, and Jordan Miner for technical editing. This work was supported by the National Institutes of Health grant under award number R01DK128805 and National Science Foundation grant No. 1939710, 2029378, and 2234150.
References
- J. D. Smith, R. Dhiman, S. Anand, E. Reza-Garduno, R. E. Cohen, G. H. McKinley and K. K. Varanasi, Droplet mobility on lubricant-impregnated surfaces, Soft Matter, 2013, 9, 1772–1780 RSC.
- C. Howell, A. Grinthal, S. Sunny, M. Aizenberg and J. Aizenberg, Designing liquid-infused surfaces for medical applications: A review, Adv. Mater., 2018, 30, 1802724 CrossRef.
- S. B. Subramanyam, G. Azimi and K. K. Varanasi, Designing lubricant-impregnated textured surfaces to resist scale formation, Adv. Mater. Interfaces, 2014, 1, 1300068 CrossRef.
- T.-S. Wong, S. H. Kang, S. K. Y. Tang, E. J. Smythe, B. D. Hatton, A. Grinthal and J. Aizenberg, Bioinspired self-repairing slippery surfaces with pressure-stable omniphobicity, Nature, 2011, 477, 443–447 CrossRef CAS PubMed.
- P. W. Wilson, W. Lu, H. Xu, P. Kim, M. J. Kreder, J. Alvarenga and J. Aizenberg, Inhibition of ice nucleation by slippery liquid-infused porous surfaces (SLIPS), Phys. Chem. Chem. Phys., 2012, 15, 581–585 RSC.
- J. Yong, F. Chen, Q. Yang, Y. Fang, J. Huo, J. Zhang and X. Hou, Nepenthes inspired design of self-repairing omniphobic slippery liquid infused porous surface (SLIPS) by femtosecond laser direct writing, Adv. Mater. Interfaces, 2017, 4, 1700552 CrossRef.
- L. Xia, S. Zhang and Z. Guo, Multifunction of biomimetic liquid infused systems derived from SLIPS theory: A review, Adv. Mater. Interfaces, 2023, 10, 2202212 CrossRef CAS.
- J. Li, T. Kleintschek, A. Rieder, Y. Cheng, T. Baumbach, U. Obst, T. Schwartz and P. A. Levkin, Hydrophobic liquid-infused porous polymer surfaces for antibacterial applications, ACS Appl. Mater. Interfaces, 2013, 5, 6704–6711 CrossRef CAS.
- C. Howell, T. L. Vu, J. J. Lin, S. Kolle, N. Juthani, E. Watson, J. C. Weaver, J. Alvarenga and J. Aizenberg, Self-replenishing vascularized fouling-release surfaces, ACS Appl. Mater. Interfaces, 2014, 6, 13299–13307 CrossRef CAS PubMed.
- N. MacCallum, C. Howell, P. Kim, D. Sun, R. Friedlander, J. Ranisau, O. Ahanotu, J. J. Lin, A. Vena, B. Hatton, T.-S. Wong and J. Aizenberg, Liquid-infused silicone as a biofouling-free medical material, ACS Biomater. Sci. Eng., 2015, 1, 43–51 CrossRef CAS PubMed.
- S. Habib, S. Zavahir, A. E. Abusrafa, A. Abdulkareem, P. Sobolčiak, M. Lehocky, D. Vesela, P. Humpolíček and A. Popelka, Slippery liquid-infused porous polymeric surfaces based on natural oil with antimicrobial effect, Polymers, 2021, 13, 206 CrossRef CAS PubMed.
- D. Quéré, Non-sticking drops, Rep. Prog. Phys., 2005, 68, 2495 CrossRef.
- P. Zhang, G. Liu, D. Zhang and H. Chen, Liquid-infused surfaces on electrosurgical instruments with exceptional antiadhesion and low-damage performances, ACS Appl. Mater. Interfaces, 2018, 10, 33713–33720 CrossRef CAS.
- V. Singh, Y.-J. Sheng and H.-K. Tsao, Self-healing atypical liquid-infused surfaces: Superhydrophobicity and superoleophobicity in submerged conditions, J. Taiwan Inst. Chem. Eng., 2019, 97, 96–104 CrossRef CAS.
- Q. Rao, Y. Lu, L. Song, Y. Hou, X. Zhan and Q. Zhang, Highly efficient self-repairing slippery liquid-infused surface with promising anti-icing and anti-fouling performance, ACS Appl. Mater. Interfaces, 2021, 13, 40032–40041 CrossRef CAS PubMed.
- M. Villegas, Y. Zhang, N. Abu Jarad, L. Soleymani and T. F. Didar, Liquid-infused surfaces: A review of theory, design, and applications, ACS Nano, 2019, 13, 8517–8536 CrossRef CAS PubMed.
- S. Hardt and G. McHale, Flow and drop transport along liquid-infused surfaces, Annu. Rev. Fluid Mech., 2022, 54, 83–104 CrossRef.
-
World Intellectual Property Organization, WO2012100100A2, 2012.
- U. Manna, N. Raman, M. A. Welsh, Y. M. Zayas-Gonzalez, H. E. Blackwell, S. P. Palecek and D. M. Lynn, Slippery liquid-infused porous surfaces that prevent microbial surface fouling and kill non-adherent pathogens in surrounding media: A controlled release approach, Adv. Funct. Mater., 2016, 26, 3599–3611 CrossRef CAS.
- R. M. Shah, A. Cihanoğlu, J. Hardcastle, C. Howell and J. D. Schiffman, Liquid-Infused Membranes Exhibit Stable Flux and Fouling Resistance, ACS Appl. Mater. Interfaces, 2022, 14, 6148–6156 CrossRef CAS PubMed.
- S. Peppou-Chapman, J. K. Hong, A. Waterhouse and C. Neto, Life and death of liquid-infused surfaces: A review on the choice, analysis and fate of the infused liquid layer, Chem. Soc. Rev., 2020, 49, 3688–3715 RSC.
- S. Sett, J. Oh, H. Cha, T. Veriotti, A. Bruno, X. Yan, G. Barac, L. W. Bolton and N. Miljkovic, Lubricant-infused surfaces for low-surface-tension fluids: The extent of lubricant miscibility, ACS Appl. Mater. Interfaces, 2021, 13, 23121–23133 CrossRef CAS.
- S. Sunny, G. Cheng, D. Daniel, P. Lo, S. Ochoa, C. Howell, N. Vogel, A. Majid and J. Aizenberg, Transparent antifouling material for improved operative field visibility in endoscopy, Proc. Natl. Acad. Sci. U. S. A., 2016, 113, 11676–11681 CrossRef CAS PubMed.
- H. Bazyar, N. Van De Beek and R. G. H. Lammertink, Liquid-infused membranes with oil-in-water emulsions, Langmuir, 2019, 35, 9513–9520 CrossRef CAS PubMed.
-
C. Fong, M. J. Andersen, E. Kunesh, E. Leonard, D. Durand, R. Coombs, A. L. Flores-Mireles and C. Howell, Removal of free liquid layer from liquid-infused catheters reduces silicone loss into the environment while maintaining adhesion resistance, medRxiv, 2023, preprint, DOI:10.1101/2023.09.14.23295548.
- Y. Kovalenko, I. Sotiri, J. V. I. Timonen, J. C. Overton, G. Holmes, J. Aizenberg and C. Howell, Bacterial interactions with immobilized liquid layers, Adv. Healthcare Mater., 2017, 6, 1600948 CrossRef.
- D. P. Regan, C. Lilly, A. Weigang, L. R. White, E. J. LeClair, A. Collins and C. Howell, Combining the geometry of folded paper with liquid-infused polymer surfaces to concentrate and localize bacterial solutions, Biointerphases, 2019, 14, 041005 CrossRef PubMed.
- P. Juuti, J. Haapanen, C. Stenroos, H. Niemelä-Anttonen, J. Harra, H. Koivuluoto, H. Teisala, J. Lahti, M. Tuominen, J. Kuusipalo, P. Vuoristo and J. M. Mäkelä, Achieving a slippery, liquid-infused porous surface with anti-icing properties by direct deposition of flame synthesized aerosol nanoparticles on a thermally fragile substrate, Appl. Phys. Lett., 2017, 110, 161603 CrossRef.
- B. Liu, K. Zhang, C. Tao, Y. Zhao, X. Li, K. Zhu and X. Yuan, Strategies for anti-icing: Low surface energy or liquid-infused?, RSC Adv., 2016, 6, 70251–70260 RSC.
- G. Zhang, Q. Zhang, T. Cheng, X. Zhan and F. Chen, Polyols-infused slippery surfaces based on magnetic Fe3O4-functionalized polymer hybrids for enhanced multifunctional anti-icing and deicing properties, Langmuir, 2018, 34, 4052–4058 CrossRef CAS PubMed.
- J. C. Overton, A. Weigang and C. Howell, Passive flux recovery in protein-fouled liquid-gated membranes, J. Membr. Sci., 2017, 539, 257–262 CrossRef CAS.
- T. V. J. Charpentier, A. Neville, S. Baudin, M. J. Smith, M. Euvrard, A. Bell, C. Wang and R. Barker, Liquid infused porous surfaces for mineral fouling mitigation, J. Colloid Interface Sci., 2015, 444, 81–86 CrossRef CAS PubMed.
- Q. Cai, J. Xu, Z. Lian, H. Yu and J. Li, Liquid-infused surfaces with anti-corrosion on magnesium alloy, J. Phys.: Conf. Ser., 2022, 2329, 012039 CrossRef CAS.
- S. Li, F. Zhao, Y. Bai, Z. Ye, Z. Feng, X. Liu, S. Gao, X. Pang, M. Sun, J. Zhang, A. Dong, W. Wang and P. Huang, Slippery liquid-infused microphase separation surface enables highly robust anti-fouling, anti-corrosion, anti-icing and anti-scaling coating on diverse substrates, Chem. Eng. J., 2022, 431, 133945 CrossRef CAS.
- Y. Liang, C. Li, P. Wang and D. Zhang, Fabrication of a robust slippery liquid infused porous surface on Q235 carbon steel for inhibiting microbiologically influenced corrosion, Colloids Surf., A, 2021, 631, 127696 CrossRef CAS.
- D. C. Leslie, A. Waterhouse, J. B. Berthet, T. M. Valentin, A. L. Watters, A. Jain, P. Kim, B. D. Hatton, A. Nedder, K. Donovan, E. H. Super, C. Howell, C. P. Johnson, T. L. Vu, D. E. Bolgen, S. Rifai, A. R. Hansen, M. Aizenberg, M. Super, J. Aizenberg and D. E. Ingber, A bioinspired omniphobic surface coating on medical devices prevents thrombosis and biofouling, Nat. Biotechnol., 2014, 32, 1134–1140 CrossRef CAS.
- J. K. Hong, K. Mathur, A. M. Ruhoff, B. Akhavan, A. Waterhouse and C. Neto, Design optimization of perfluorinated liquid-infused surfaces for blood-contacting applications, Adv. Mater. Interfaces, 2022, 9, 2102214 CrossRef CAS.
- J. K. Hong, A. M. Ruhoff, K. Mathur, C. Neto and A. Waterhouse, Mechanisms for reduced fibrin clot formation on liquid-infused surfaces, Adv. Healthcare Mater., 2022, 11, 2201360 CrossRef CAS.
- A. K. Epstein, T.-S. Wong, R. A. Belisle, E. M. Boggs and J. Aizenberg, Liquid-infused structured surfaces with exceptional anti-biofouling performance, Proc. Natl. Acad. Sci. U. S. A., 2012, 109, 13182–13187 CrossRef CAS.
- L. Xiao, J. Li, S. Mieszkin, A. Di Fino, A. S. Clare, M. E. Callow, J. A. Callow, M. Grunze, A. Rosenhahn and P. A. Levkin, Slippery liquid-infused porous surfaces showing marine antibiofouling properties, ACS Appl. Mater. Interfaces, 2013, 5, 10074–10080 CrossRef CAS PubMed.
- S. Basu, B. M. Hanh, J. Q. I. Chua, D. Daniel, M. H. Ismail, M. Marchioro, S. Amini, S. A. Rice and A. Miserez, Green biolubricant infused slippery surfaces to combat marine biofouling, J. Colloid Interface Sci., 2020, 568, 185–197 CrossRef CAS PubMed.
- M. J. Andersen, C. Fong, A. A. La Bella, J. J. Molina, A. Molesan, M. M. Champion, C. Howell and A. L. Flores-Mireles, Inhibiting host-protein deposition on urinary catheters reduces associated urinary tract infections, eLife, 2022, 11, e75798 CrossRef CAS PubMed.
- P. G. Wang, M. Xian, X. Tang, X. Wu, Z. Wen, T. Cai and A. J. Janczuk, Nitric oxide donors: Chemical activities and biological applications, Chem. Rev., 2002, 102, 1091–1134 CrossRef CAS PubMed.
- A. A. Eroy-Reveles and P. K. Mascharak, Nitric oxide-donating materials and their potential in pharmacological applications for site-specific nitric oxide delivery, Future Med. Chem., 2009, 1, 1497–1507 CrossRef CAS PubMed.
- J. N. Sharma, A. Al-Omran and S. S. Parvathy, Role of nitric oxide in inflammatory diseases, Inflammopharmacology, 2007, 15, 252–259 CrossRef CAS PubMed.
- D. O. Schairer, J. S. Chouake, J. D. Nosanchuk and A. J. Friedman, The potential of nitric oxide releasing therapies as antimicrobial agents, Virulence, 2012, 3, 271–279 CrossRef.
- X. Wang, A. Jolliffe, B. Carr, Q. Zhang, M. Bilger, Y. Cui, J. Wu, X. Wang, M. Mahoney, A. Rojas-Pena, M. J. Hoenerhoff, J. Douglas, R. H. Bartlett, C. Xi, J. L. Bull and M. E. Meyerhoff, Nitric oxide-releasing semi-crystalline thermoplastic polymers: Preparation, characterization and application to devise anti-inflammatory and bactericidal implants, Biomater. Sci., 2018, 6, 3189–3201 RSC.
- M. K. Chug and E. J. Brisbois, Recent developments in multifunctional antimicrobial surfaces and applications toward advanced nitric oxide-based biomaterials, ACS Mater. Au, 2022, 2, 525–551 CrossRef CAS PubMed.
- E. J. Brisbois, T. C. Major, M. J. Goudie, R. H. Bartlett, M. E. Meyerhoff and H. Handa, Improved hemocompatibility of silicone rubber extracorporeal tubing via solvent swelling-impregnation of S-nitroso-N-acetylpenicillamine (SNAP) and evaluation in rabbit thrombogenicity model, Acta Biomater., 2016, 37, 111–119 CrossRef CAS PubMed.
- M. J. Goudie, J. Pant and H. Handa, Liquid-infused nitric oxide-releasing (LINORel) silicone for decreased fouling, thrombosis, and infection of medical devices, Sci. Rep., 2017, 7, 13623 CrossRef PubMed.
- S. M. Lantvit, B. J. Barrett and M. M. Reynolds, Nitric oxide releasing material adsorbs more fibrinogen, J. Biomed. Mater. Res., Part A, 2013, 101, 3201–3210 CrossRef.
- Y. Qian, M. K. Chug and E. J. Brisbois, Nitric oxide-releasing silicone oil with tunable payload for antibacterial applications, ACS Appl. Bio Mater., 2022, 5, 3396–3404 CrossRef CAS PubMed.
- K. H. Homeyer, M. J. Goudie, P. Singha and H. Handa, Liquid-infused nitric-oxide-releasing silicone foley urinary catheters for prevention of catheter-associated urinary tract infections, ACS Biomater. Sci. Eng., 2019, 5, 2021–2029 CrossRef CAS PubMed.
- M. Douglass, S. Ghalei, E. Brisbois and H. Handa, Potent, broad-spectrum antimicrobial effects of S-nitroso-N-acetylpenicillamine-impregnated nitric oxide-releasing latex urinary catheters, ACS Appl. Bio Mater., 2022, 5, 700–710 CrossRef CAS PubMed.
- M. Douglass, S. Hopkins, M. K. Chug, G. Kim, M. R. Garren, M. Ashcraft, D. T. Nguyen, N. Tayag, H. Handa and E. J. Brisbois, Reduction in foreign body response and improved antimicrobial efficacy via silicone-oil-infused nitric-oxide-releasing medical-grade cannulas, ACS Appl. Mater. Interfaces, 2021, 13, 52425–52434 CrossRef CAS.
- Y. Liu, T. Chen, N. Gu and F. Yang, Recent progress in bioactive gas delivery for cancer immunotherapy, Prog. Biomed. Eng., 2022, 4, 022001 CrossRef.
- R. Siracusa, V. A. Voltarelli, A. T. Salinaro, S. Modafferi, S. Cuzzocrea, E. J. Calabrese, R. Di Paola, L. E. Otterbein and V. Calabrese, NO, CO and H2S: A trinacrium of bioactive gases in the brain, Biochem. Pharmacol., 2022, 202, 115122 CrossRef CAS PubMed.
- A. A. Holder, S. C. Marshall, P. G. Wang and C.-H. Kwak, The mechanism of the decomposition of a bronchodilator, S-nitroso-N-acetyl-D,L-penicillamine (SNAP), by a bronchoconstrictor, aqueous sulfite: detection of the N-nitrosohydroxylamine-N-sulfonate ion, Bull. Korean Chem. Soc., 2003, 24, 350–356 CrossRef CAS.
- A. P. Dicks, H. R. Swift, D. L. H. Williams, A. R. Butler, H. H. Al-Sa'doni and B. G. Cox, Identification
of Cu+ as the effective reagent in nitric oxide formation from S-nitrosothiols (RSNO), J. Chem. Soc., Perkin Trans. 2, 1996, 481–487 RSC.
- M. C. Frost and M. E. Meyerhoff, Controlled photoinitiated release of nitric oxide from polymer films containing S-nitroso-N-acetyl-dl-penicillamine derivatized fumed silica filler, J. Am. Chem. Soc., 2004, 126, 1348–1349 CrossRef CAS PubMed.
- T. R. Roberts, G. T. Harea, Y. Zang, R. P. Devine, P. Maffe, H. Handa and A. I. Batchinsky, A dual-action nitric oxide-releasing slippery surface for extracorporeal organ support: Dynamic in vitro hemocompatibility evaluation, J. Biomed. Mater. Res., Part B, 2023, 111, 923–932 CrossRef CAS PubMed.
- H. Liang, P. Nacharaju, A. Friedman and J. M. Friedman, Nitric oxide generating/releasing materials, Future Sci. OA, 2015, 1, FSO54 CrossRef PubMed.
- A. Kinnunen, I. Talvitie, J. Ottelin, J. Heinonen and S. Junnila, Carbon sequestration and storage potential of urban residential environment – A review, Sustain. Cities Soc., 2022, 84, 104027 CrossRef.
- V. I. Fagorite, S. O. Onyekuru, A. I. Opara and E. E. Oguzie, The major techniques, advantages, and pitfalls of various methods used in geological carbon sequestration, Int. J. Environ. Sci. Technol., 2023, 20, 4585–4614 CrossRef.
- M. Kazemian and B. Shafei, Carbon sequestration and storage in concrete: A state-of-the-art review of compositions, methods, and developments, J. CO2 Util., 2023, 70, 102443 CrossRef CAS.
- M. S. Yeganeh, A. Jusufi, S. Deighton, M. S. Ide, M. Siskin, A. Jaishankar, C. Maldarelli, P. Bertolini, B. Natarajan, J. L. Vreeland, M. A. King and A. R. Konicek, Solid with infused reactive liquid (SWIRL): A novel liquid-based separation approach for effective CO2 capture, Sci. Adv., 2022, 8, eabm0144 CrossRef CAS PubMed.
- G. T. Rochelle, Amine scrubbing for CO2 capture, Science, 2009, 325, 1652–1654 CrossRef CAS PubMed.
- H. M. Tran, F.-J. Tsai, Y.-L. Lee, J.-H. Chang, L.-T. Chang, T.-Y. Chang, K. F. Chung, H.-P. Kuo, K.-Y. Lee, K.-J. Chuang and H.-C. Chuang, The impact of air pollution on respiratory diseases in an era of climate change: A review of the current evidence, Sci. Total Environ., 2023, 898, 166340 CrossRef CAS PubMed.
- P. Sicard, E. Agathokleous, S. C. Anenberg, A. De Marco, E. Paoletti and V. Calatayud, Trends in urban air pollution over the last two decades: A global perspective, Sci. Total Environ., 2023, 858, 160064 CrossRef CAS PubMed.
- R. Thompson, R. B. Smith, Y. B. Karim, C. Shen, K. Drummond, C. Teng and M. B. Toledano, Air pollution and human cognition: A systematic review and meta-analysis, Sci. Total Environ., 2023, 859, 160234 CrossRef CAS PubMed.
- I. Zahoor and A. Mushtaq, Water pollution from agricultural activities: A critical global review, International Journal of Chemical and Biochemical Sciences, 2023, 23, 164–176 Search PubMed.
- S. Sahoo and S. Goswami, Theoretical framework for assessing the economic and environmental impact of water pollution: A detailed study on sustainable development of India, J. Future Sustain., 2024, 4, 23–34 CrossRef.
- C. J. L. Murray, K. S. Ikuta, F. Sharara, L. Swetschinski, G. R. Aguilar, A. Gray, C. Han, C. Bisignano, P. Rao, E. Wool, S. C. Johnson, A. J. Browne, M. G. Chipeta, F. Fell, S. Hackett, G. Haines-Woodhouse, B. H. Kashef Hamadani, E. A. P. Kumaran, B. McManigal, S. Achalapong, R. Agarwal, S. Akech, S. Albertson, J. Amuasi, J. Andrews, A. Aravkin, E. Ashley, F.-X. Babin, F. Bailey, S. Baker, B. Basnyat, A. Bekker, R. Bender, J. A. Berkley, A. Bethou, J. Bielicki, S. Boonkasidecha, J. Bukosia, C. Carvalheiro, C. Castañeda-Orjuela, V. Chansamouth, S. Chaurasia, S. Chiurchiù, F. Chowdhury, R. C. Donatien, A. J. Cook, B. Cooper, T. R. Cressey, E. Criollo-Mora, M. Cunningham, S. Darboe, N. P. J. Day, M. De Luca, K. Dokova, A. Dramowski, S. J. Dunachie, T. Duong Bich, T. Eckmanns, D. Eibach, A. Emami, N. Feasey, N. Fisher-Pearson, K. Forrest, C. Garcia, D. Garrett, P. Gastmeier, A. Z. Giref, R. C. Greer, V. Gupta, S. Haller, A. Haselbeck, S. I. Hay, M. Holm, S. Hopkins, Y. Hsia, K. C. Iregbu, J. Jacobs, D. Jarovsky, F. Javanmardi, A. W. J. Jenney, M. Khorana, S. Khusuwan, N. Kissoon, E. Kobeissi, T. Kostyanev, F. Krapp, R. Krumkamp, A. Kumar, H. H. Kyu, C. Lim, K. Lim, D. Limmathurotsakul, M. J. Loftus, M. Lunn, J. Ma, A. Manoharan, F. Marks, J. May, M. Mayxay, N. Mturi, T. Munera-Huertas, P. Musicha, L. A. Musila, M. M. Mussi-Pinhata, R. N. Naidu, T. Nakamura, R. Nanavati, S. Nangia, P. Newton, C. Ngoun, A. Novotney, D. Nwakanma, C. W. Obiero, T. J. Ochoa, A. Olivas-Martinez, P. Olliaro, E. Ooko, E. Ortiz-Brizuela, P. Ounchanum, G. D. Pak, J. L. Paredes, A. Y. Peleg, C. Perrone, T. Phe, K. Phommasone, N. Plakkal, A. Ponce-de-Leon, M. Raad, T. Ramdin, S. Rattanavong, A. Riddell, T. Roberts, J. V. Robotham, A. Roca, V. D. Rosenthal, K. E. Rudd, N. Russell, H. S. Sader, W. Saengchan, J. Schnall, J. A. G. Scott, S. Seekaew, M. Sharland, M. Shivamallappa, J. Sifuentes-Osornio, A. J. Simpson, N. Steenkeste, A. J. Stewardson, T. Stoeva, N. Tasak, A. Thaiprakong, G. Thwaites, C. Tigoi, C. Turner, P. Turner, H. R. van Doorn, S. Velaphi, A. Vongpradith, M. Vongsouvath, H. Vu, T. Walsh, J. L. Walson, S. Waner, T. Wangrangsimakul, P. Wannapinij, T. Wozniak, T. E. M. W. Young-Sharma, K. C. Yu, P. Zheng, B. Sartorius, A. D. Lopez, A. Stergachis, C. Moore, C. Dolecek and M. Naghavi, Global burden of bacterial antimicrobial resistance in 2019: A systematic analysis, Lancet, 2022, 399, 629–655 CrossRef CAS PubMed.
- CDC, The biggest antibiotic-resistant threats in the U.S., https://www.cdc.gov/drugresistance/biggest-threats.html, (accessed 28 December 2023).
- M. A. Kohanski, M. A. DePristo and J. J. Collins, Sub-lethal antibiotic treatment leads to multidrug resistance via radical-induced mutagenesis, Mol. Cell, 2010, 37, 311–320 CrossRef CAS PubMed.
- M. A. Alfhili and M.-H. Lee, Triclosan: An update on biochemical and molecular mechanisms, Oxid. Med. Cell. Longevity, 2019, 2019, e1607304 Search PubMed.
- Y. Wang and W. Liang, Occurrence, toxicity, and removal methods of triclosan: A timely review, Curr. Pollut. Rep., 2021, 7, 31–39 CrossRef CAS.
- U. Manna and D. M. Lynn, Fabrication of liquid-infused surfaces using reactive polymer multilayers: Principles for manipulating the behaviors and mobilities of aqueous fluids on slippery liquid interfaces, Adv. Mater., 2015, 27, 3007–3012 CrossRef CAS PubMed.
- M. Milanović, L. Đurić, N. Milošević and N. Milić, Comprehensive insight into triclosan—from widespread occurrence to health outcomes, Environ. Sci. Pollut. Res., 2023, 30, 25119–25140 CrossRef PubMed.
- B. L. Bassler and R. Losick, Bacterially speaking, Cell, 2006, 125, 237–246 CrossRef CAS PubMed.
- H. Z. Asfour, Anti-quorum sensing natural compounds, J. Microsc. Ultrastruct., 2018, 6, 1–10 CrossRef PubMed.
- M. J. Kratochvil, M. A. Welsh, U. Manna, B. J. Ortiz, H. E. Blackwell and D. M. Lynn, Slippery liquid-infused porous surfaces that prevent bacterial surface fouling and inhibit virulence phenotypes in surrounding planktonic cells, ACS Infect. Dis., 2016, 2, 509–517 CrossRef CAS PubMed.
- H. Wang, Y. Fan, Y. Hou, B. Chen, J. Lei, S. Yu, X. Chen and X. Hou, Host-guest liquid gating mechanism with specific recognition interface behavior for universal quantitative chemical detection, Nat. Commun., 2022, 13, 1906 CrossRef CAS PubMed.
- X. Hou, Y. Hu, A. Grinthal, M. Khan and J. Aizenberg, Liquid-based gating mechanism with tunable multiphase selectivity and antifouling behaviour, Nature, 2015, 519, 70–73 CrossRef CAS PubMed.
- B. Chen, R. Zhang, Y. Hou, J. Zhang, S. Chen, Y. Han, X. Chen and X. Hou, Light-responsive and corrosion-resistant gas valve with non-thermal effective liquid-gating positional flow control, Light: Sci. Appl., 2021, 10, 127 CrossRef CAS PubMed.
- H. Agarwal, T. J. Polaske, G. Sánchez-Velázquez, H. E. Blackwell and D. M. Lynn, Slippery nanoemulsion-infused porous surfaces (SNIPS): Anti-fouling coatings that can host and sustain the release of water-soluble agents, Chem. Commun., 2021, 57, 12691–12694 RSC.
- M. Kole and S. Khandekar, Engineering applications of ferrofluids: A review, J. Magn. Magn. Mater., 2021, 537, 168222 CrossRef CAS.
-
S. S. Papell, Low viscosity magnetic fluid obtained by the colloidal suspension of magnetic particles, US Pat., US3215572A, 1965 Search PubMed.
- W. Bottenberg, L. Melillo and K. Raj, The dependence of loudspeaker design parameters on the properties of magnetic fluids, J. Audio Eng. Soc., 1980, 28, 17–25 Search PubMed.
- J. R. McCarthy and R. Weissleder, Multifunctional magnetic nanoparticles for targeted imaging and therapy, Adv. Drug Delivery Rev., 2008, 60, 1241–1251 CrossRef CAS PubMed.
- C. Sun, J. S. H. Lee and M. Zhang, Magnetic nanoparticles in MR imaging and drug delivery, Adv. Drug Delivery Rev., 2008, 60, 1252–1265 CrossRef CAS PubMed.
- X. Zhang, L. Sun, Y. Yu and Y. Zhao, Flexible ferrofluids: Design and applications, Adv. Mater., 2019, 31, 1903497 CrossRef CAS PubMed.
- V. Socoliuc, M. V. Avdeev, V. Kuncser, R. Turcu, E. Tombácz and L. Vékás, Ferrofluids and bio-ferrofluids: Looking back and stepping forward, Nanoscale, 2022, 14, 4786–4886 RSC.
- O. Oehlsen, S. I. Cervantes-Ramírez, P. Cervantes-Avilés and I. A. Medina-Velo, Approaches on ferrofluid synthesis and applications: Current status and future perspectives, ACS Omega, 2022, 7, 3134–3150 CrossRef CAS PubMed.
- K. S. Khalil, S. R. Mahmoudi, N. Abu-dheir and K. K. Varanasi, Active surfaces: Ferrofluid-impregnated surfaces for active manipulation of droplets, Appl. Phys. Lett., 2014, 105, 041604 CrossRef.
- J. Zhang, X. Wang, Z. Wang, S. Pan, B. Yi, L. Ai, J. Gao, F. Mugele and X. Yao, Wetting ridge assisted programmed magnetic actuation of droplets on ferrofluid-infused surface, Nat. Commun., 2021, 12, 7136 CrossRef CAS PubMed.
- S. Zhu, Y. Bian, T. Wu, C. Chen, Y. Jiao, Z. Jiang, Z. Huang, E. Li, J. Li, J. Chu, Y. Hu, D. Wu and L. Jiang, High Performance Bubble Manipulation on Ferrofluid-Infused Laser-Ablated Microstructured Surfaces, Nano Lett., 2020, 20, 5513–5521 CrossRef CAS PubMed.
- P. Irajizad, M. Hasnain, N. Farokhnia, S. M. Sajadi and H. Ghasemi, Magnetic slippery extreme icephobic surfaces, Nat. Commun., 2016, 7, 13395 CrossRef CAS PubMed.
- W. Wang, J. V. I. Timonen, A. Carlson, D.-M. Drotlef, C. T. Zhang, S. Kolle, A. Grinthal, T.-S. Wong, B. Hatton, S. H. Kang, S. Kennedy, J. Chi, R. T. Blough, M. Sitti, L. Mahadevan and J. Aizenberg, Multifunctional ferrofluid-infused surfaces with reconfigurable multiscale topography, Nature, 2018, 559, 77–82 CrossRef CAS PubMed.
- D. Luo, K. A. Carter, A. Razi, J. Geng, S. Shao, D. Giraldo, U. Sunar, J. Ortega and J. F. Lovell, Doxorubicin encapsulated in stealth liposomes conferred with light-triggered drug release, Biomaterials, 2016, 75, 193–202 CrossRef CAS PubMed.
- Y. Park, C. Hashimoto, Y. Ozaki and Y. M. Jung, Understanding the phase transition of linear poly(N-isopropylacrylamide) gel under the heating and cooling processes, J. Mol. Struct., 2016, 1124, 144–150 CrossRef CAS.
- J. L. Paris, M. Manzano, M. V. Cabañas and M. Vallet-Regí, Mesoporous silica nanoparticles engineered for ultrasound-induced uptake by cancer cells, Nanoscale, 2018, 10, 6402–6408 RSC.
- C. Howell, T. L. Vu, C. P. Johnson, X. Hou, O. Ahanotu, J. Alvarenga, D. C. Leslie, O. Uzun, A. Waterhouse, P. Kim, M. Super, M. Aizenberg, D. E. Ingber and J. Aizenberg, Stability of surface-immobilized lubricant interfaces under flow, Chem. Mater., 2015, 27, 1792–1800 CrossRef CAS.
- Krytox™ 157 FSL Oil, https://miller-stephenson.com/product/krytox-157-fsl/, (accessed 3 January 2024).
- N. H. L. Leung, Transmissibility and transmission of respiratory viruses, Nat. Rev. Microbiol., 2021, 19, 528–545 CrossRef CAS PubMed.
- D. P. Regan, C. Fong, A. C. S. Bond, C. Desjardins, J. Hardcastle, S.-H. Hung, A. P. Holmes, J. D. Schiffman, M. S. Maginnis and C. Howell, Improved recovery of captured airborne bacteria and viruses with liquid-coated air filters, ACS Appl. Mater. Interfaces, 2022, 14, 50543–50556 CrossRef CAS PubMed.
- J. W. G. Tyrrell and P. Attard, Images of nanobubbles on hydrophobic surfaces and their interactions, Phys. Rev. Lett., 2001, 87, 176104 CrossRef CAS PubMed.
- X. Zhang and D. Lohse, Perspectives on surface nanobubbles, Biomicrofluidics, 2014, 8, 041301 CrossRef PubMed.
- M. Alheshibri, J. Qian, M. Jehannin and V. S. J. Craig, A history of nanobubbles, Langmuir, 2016, 32, 11086–11100 CrossRef CAS PubMed.
- C. Vega-Sánchez, S. Peppou-Chapman, L. Zhu and C. Neto, Nanobubbles explain the large slip observed on lubricant-infused surfaces, Nat. Commun., 2022, 13, 351 CrossRef PubMed.
- S. Peppou-Chapman, C. Vega-Sánchez and C. Neto, Detection of nanobubbles on lubricant-infused surfaces using AFM meniscus force measurements, Langmuir, 2022, 38, 10234–10243 CrossRef CAS PubMed.
- M. Muschi, B. Brudieu, J. Teisseire and A. Sauret, Drop impact dynamics on slippery liquid-infused porous surfaces: Influence of oil thickness, Soft Matter, 2018, 14, 1100–1107 RSC.
- A. Vashist, A. Kaushik, K. Alexis, R. Dev Jayant, V. Sagar, A. Vashist and M. Nair, Bioresponsive injectable hydrogels for on-demand drug release and tissue engineering, Curr. Pharm. Des., 2017, 23, 3595–3602 CrossRef CAS PubMed.
- M. Biondi, F. Ungaro, F. Quaglia and P. A. Netti, Controlled drug delivery in tissue engineering, Adv. Drug Delivery Rev., 2008, 60, 229–242 CrossRef CAS PubMed.
- M. E. Alkahtani, M. Elbadawi, C. A. R. Chapman, R. A. Green, S. Gaisford, M. Orlu and A. W. Basit, Electroactive polymers for on-demand drug release, Adv. Healthcare Mater., 2024, 13, 2301759 CrossRef CAS PubMed.
- D. Samanta, R. Mehrotra, K. Margulis and R. N. Zare, On-demand electrically controlled drug release from resorbable nanocomposite films, Nanoscale, 2017, 9, 16429–16436 RSC.
- Z. Sheng, M. Zhang, J. Liu, P. Malgaretti, J. Li, S. Wang, W. Lv, R. Zhang, Y. Fan, Y. Zhang, X. Chen and X. Hou, Reconfiguring confined magnetic colloids with tunable fluid transport behavior, Natl. Sci. Rev., 2021, 8, nwaa301 CrossRef CAS PubMed.
- N. Vogel, R. A. Belisle, B. Hatton, T.-S. Wong and J. Aizenberg, Transparency and damage tolerance of patternable omniphobic lubricated surfaces based on inverse colloidal monolayers, Nat. Commun., 2013, 4, 2176 CrossRef PubMed.
- F. L. Geyer, E. Ueda, U. Liebel, N. Grau and P. A. Levkin, Superhydrophobic–superhydrophilic micropatterning: Towards genome-on-a-chip cell microarrays, Angew. Chem., Int. Ed., 2011, 50, 8424–8427 CrossRef CAS PubMed.
- E. Ueda and P. A. Levkin, Emerging applications of superhydrophilic-superhydrophobic micropatterns, Adv. Mater., 2013, 25, 1234–1247 CrossRef CAS PubMed.
- J. Bruchmann, I. Pini, T. S. Gill, T. Schwartz and P. A. Levkin, Patterned SLIPS for the formation of arrays of biofilm microclusters with defined geometries, Adv. Healthcare Mater., 2017, 6, 1601082 CrossRef PubMed.
- D. Paulssen, W. Feng, I. Pini and P. A. Levkin, Formation of liquid–liquid micropatterns through guided liquid displacement on liquid-infused surfaces, Adv. Mater. Interfaces, 2018, 5, 1800852 CrossRef.
- M. Pelizzari, G. McHale, S. Armstrong, H. Zhao, R. Ledesma-Aguilar, G. G. Wells and H. Kusumaatmaja, Droplet self-propulsion on slippery liquid-infused surfaces with dual-lubricant wedge-shaped wettability patterns, Langmuir, 2023, 39, 15676–15689 CrossRef CAS PubMed.
- K. Marquis, B. Chasse, D. P. Regan, A. L. Boutiette, A. Khalil and C. Howell, Vascularized polymers spatially control bacterial cells on surfaces, Adv. Biosyst., 2020, 4, 1900216 CrossRef CAS PubMed.
- B. Dixon, C. Sui, A. Briley, P.-C. Hsu and C. Howell, Continuous, nondestructive detection of microorganism growth at buried interfaces with vascularized polymers, ACS Appl. Bio Mater., 2023, 6, 519–528 CrossRef CAS PubMed.
|
This journal is © Institute of Process Engineering of CAS 2024 |
Click here to see how this site uses Cookies. View our privacy policy here.