DOI:
10.1039/D3MA00636K
(Review Article)
Mater. Adv., 2024,
5, 9160-9174
Multifunctional carbon-based nanostructures (CBNs) for advanced biomedical applications – a perspective and review
Received
31st August 2023
, Accepted 6th October 2024
First published on 14th October 2024
Abstract
Carbon-based nanostructures (CBNs) have attracted immense attention from biomedical researchers due to their unique combination of extraordinary mechanical, thermal, electrical, and optical properties. They can be easily conjugated with several organic and inorganic molecules, enhancing their potential to perform advanced therapeutic actions, which are not possible with existing materials and techniques. As such, the current advances in some of the diagnostic and therapeutic applications of CBNs are discussed in this review. In particular, the applications of carbon nanotubes (CNTs), graphene, and its oxides, as well as diamond-like carbon in tissue engineering, drug delivery, antimicrobial coatings, and medical diagnostics are critically reviewed. Among the several types of CBNs currently in use, it is shown that nanocomposites of functionalized graphene oxide outweigh the mechanical strength of other biomaterials by 110% without compromise to their biocompatibility. Similarly, due to the unique combination of the surface properties of CNTs, such as charge, polarity, and chemistry, these materials are useful for detecting protein molecules at concentrations as low as 5 ng mL−1. Due to its ease of production, robust mechanical strength, and biocompatibility, DLC is effective as an antimicrobial coating for biomedical implants and devices. Further, we provide insight into the biotoxicity and limitations of current CBNs and highlight the need for a novel biomaterial that can outperform the materials that are currently in use. Finally, the future perspective of carbon-based nanostructures in biomedical applications is discussed by introducing Q-carbon, a newly discovered ferromagnetic phase of carbon with interesting structural and functional properties. We describe the structure and multifunctional capabilities of Q-carbon. We envision Q-carbon as an alternative to CBNs for therapeutic applications, diagnostic applications, and as a protective coating for medical devices.
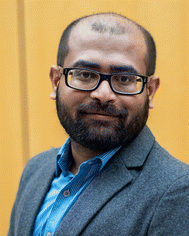
Naveen Narasimhachar Joshi
| Naveen Joshi is a PhD candidate in Materials Science and Engineering at North Carolina State University. He graduated M.Tech with the Institute Gold Medal from IIT Kharagpur in 2021. During master's, he worked on the development of chemical precursors for the synthesis of multifunctional-magnetic-oxide nanostructures. His doctoral research is focused on the non-equilibrium growth of novel phases in carbon and silicon for biomedical, magnetic, and optoelectronic applications. He has published 15+ research papers in archival journals, written 2 book chapters, and presented at 10+ international conferences in India and USA. He is an active reviewer for various high-impact journals of Elsevier. |
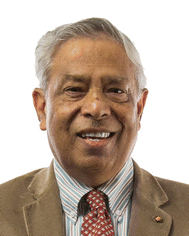
Jagdish Narayan
| Dr Jagdish Narayan is Fan Family Distinguished Chair Professor of Materials Science and Engineering at North Carolina State University. He has made pioneering contributions in laser-solid interactions and transient thermal processing of materials, laser annealing and pulsed laser deposition, defects and interfaces and domain matching epitaxy. His recent research pertains to discovery of Q-carbon and Q-BN and direct conversion of carbon into diamond and h-BN into c-BN at ambient temperatures and pressures. His honors include NAE, NAI and NAS-I, ASM Gold Medal, Acta Materialia Gold Medal, TMS RF Mehl Gold Medal; and Fellow MRS, APS, AAAS, TMS, and ASM. |
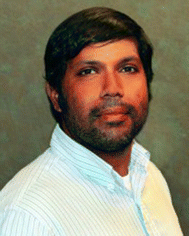
Roger Narayan
| Dr Roger Narayan (MD, PhD) is Distinguished Professor in the Joint Department of Biomedical Engineering of University of North Carolina and North Carolina State University. He has pioneered laser-based additive manufacturing techniques; including laser direct writing and two-photon polymerization, to process materials with micrometer scale and sub-micrometer scale features for medical applications. He has authored over 300 journal papers, and edited Biomedical Materials (Springer), Handbook of Materials for Medical Devices (ASM International), Encyclopedia of Biomedical Engineering (Elsevier), and the Encyclopedia of Sensors and Biosensors (Elsevier). He has received many honors, including Fellow of TMS, MRS, AAAS, ASM, AIMBE, ASME, ACerS. |
Introduction
Biomaterials have gained significant focus over the last four decades as a unique means of treating various diseases.1 The objective of medical treatment is no longer limited to external medical devices but an amalgamation of implant devices functionalized with biomaterials and therapeutic approaches. Approximately 8000 medical devices involve the use of biomaterials to diagnose and/or treat medical conditions.2 The efficacy of a biomaterial is regulated by its response to its biological surroundings. A functional biomaterial is characterized by its ability to perform without affecting the functions of the human body and offers excellent mechanical strength and prolonged resistance to wear and corrosion.3 Along with biocompatibility, it should be non-toxic to the human body.
In that regard, several conventional metals and alloys, such as titanium and its oxides, stainless steel, and Nitinol, have been used for different biomedical applications for a long time.4,5 However, metals release ions into their surroundings gradually and can prove to be cytotoxic. Metals corrode and degrade over time and thus fail to offer a sustainable solution to the problem. This issue calls for sustainable design and development of biomaterials with enhanced capabilities. In the case of first-generation implants, the prototypes were primarily designed based on the bulk properties of the chosen material. However, recent advancements in nanomaterial research have led to the evolution of biomedical devices controlled by their surface properties, offering improved performance and efficiency.6
Due to their atypical surface structure and number density, nanoscale materials significantly alter their functional properties.7 As such, nanoparticles can be engineered to allow site-specific and faster distribution in the body.8 Among nanoparticles in general, carbon nanomaterials demonstrate a wide range of structures and morphology, including nanodiamonds (0D), carbon nanotubes (CNTs) (1D), and graphene (2D); each of these materials exhibits unique functionalities. Furthermore, carbon-based nanostructures (CBNs) have the highest rate of distribution in the body, making them suitable candidates for biomedical implant devices.9 Several functional CBNs have played a crucial role in clinical and biomedical research due to their unique combination of extraordinary mechanical strength, high chemical resistance, biocompatibility, and adsorption properties.10,11 For instance, nanodiamonds with superior biocompatibility are explored for bioimaging and phototherapy applications.12 A facile technique for uptake and clearance in melanoma cells, exploiting the surface charges in graphene quantum dots (GCD), has also been demonstrated.13 As such, carbon dots or carbon quantum dots are being widely explored for theranostic and diagnostic applications due to their excellent biocompatibility, photostability, tunable fluorescence emission, and excitation properties.14–16
Diamond-like carbon (DLC) coatings offer unique properties such as excellent wear resistance, chemical inertness, and biocompatibility, making them suitable for orthopedic and cardiovascular implants.3 In addition, functionalized CNTs are being explored as biomaterial reinforcements due to their unique structure and physicochemical properties.10 Moreover, chemically modified CNTs are emerging as promising candidates in tissue engineering due to their multifunctional capabilities.17–19 Similarly, we have recently shown that reduced graphene oxide (rGO) performed exceptionally well as stent coatings, facilitating the growth of outgrowth endothelial cells on the implants. Fig. 1 shows the quantification and morphology of the proliferation of outgrowth endothelial cells on the bare silicon substrate and those coated with diamond-like carbon and reduced graphene oxide layers. rGO showed about a 150% increase in the proliferation of endothelial cells. This study also showed that rGO is hemocompatible and was effective in controlling the adverse physiological response from the cellular surroundings.20 Q-Carbon, a newly discovered phase of carbon, shows exceptional mechanical properties compared to all other CBNs, making it a promising candidate for biomedical devices.21
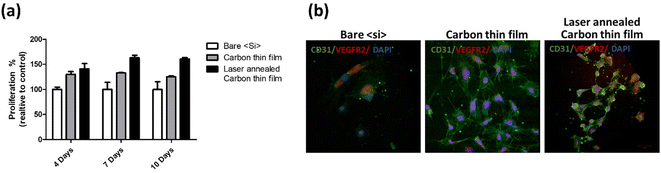 |
| Fig. 1 (a) Proliferation of outgrowth endothelial cells on silicon substrates (b) Morphology and phenotypic expression of outgrowth endothelial cells cultured on Si substrates. Si substrates coated with diamond-like carbon and laser annealed (subsequently forming rGO) showed the highest proliferation rate at all time points.20 | |
Carbon-based nanomaterials have demonstrated significant potential for use in biomedicine, including use in diagnosis and therapy.22 The tunable exterior surface function groups in CBNs enable their seamless integration into a wide range of biomaterials, including lipid-based, metal-based, and polymer-based biological structures; these materials may find use for bioimaging and sensing applications.23–25 In addition to their capability for functionalization, nanocarbon-embedded probes may have use in the detection of individual biomolecules such as DNA. Moreover, the structural strength and tunable functional (e.g., electrical, optical, magnetic, and chemical) properties of these nanostructures facilitate their use in developing personalized tools with point-of-care diagnostics.26 The high strength and biocompatibility of carbon nanostructures also make them suitable for therapeutic applications. Specifically, the ability of carbon nanostructures to manipulate individual molecules at a cellular level has made these materials potential candidates for drug delivery and regenerative medicine applications. Furthermore, carbon nanostructures offer the capability for directing cell differentiation toward specific lineages, making these materials valuable tools in tissue engineering.22 For instance, CBNs have been considered for use in neural scaffold engineering due to their unique combination of mechanical strength, biocompatibility, and tunable electrical conductivity, which sets these materials apart from other nanomaterials. The diversity of CBNs in various biomedical applications is largely attributed to their structure and properties, which can be controlled by selecting the appropriate processing technique. Some recent examples of carbon nanostructures and the most common processing techniques employed for biomedical applications are listed in Table 1.
Table 1 Most common types of carbon nanostructures, processing techniques, and their biomedical applications27
Carbon nanostructure |
Processing technique |
Application |
Carbon quantum dots |
Hydrothermal |
Bioimaging |
Biosensing |
Antibacterial |
Microwave |
Bioimaging |
Pyrolysis |
Biosensing |
Analgesic |
Sonochemical |
Bioimaging |
Nano diamonds (NDs) |
Laser ablation |
Biosensing |
Oxidized NDs |
Microplasma jet |
Biosensing |
Oxidized carbon nano tubes |
Arc discharge |
Biosensing |
Laser ablation |
Bioimaging |
Graphene oxide (GO) |
Electrochemical |
Biosensing |
Photoelectrochemical |
Biosensing |
Drug delivery |
Reduced GO |
Ascorbic acid reductant |
Drug delivery |
Diamond-like carbon |
Chemical vapor deposition |
Protective coatings |
This review provides a comprehensive evaluation of the performance and biomedical applications of three primary classes of CBNs: diamond-like carbon (DLC), carbon nanotubes (CNTs), as well as graphene and its oxides. We present a critical assessment of their applications in diagnostics, including bioimaging and biosensing, therapeutics (e.g., drug delivery), and tissue engineering. Further, we explore the potential of CBNs as antimicrobial and protective coatings for biomedical implants and devices. Table 2 provides a comprehensive list of the biomedical applications of the carbon-based nanostructures that are discussed in this paper. Finally, we present an overview of the biotoxicity and limitations of existing CBNs as well as introduce Q-carbon as an emergent biomaterial with enhanced structural strength and multifunctional capabilities, with the potential to surpass the performance of existing CBNs. Fig. 2 illustrates the various classes of CBNs employed in different areas of biomedical research that are reviewed in this paper.
Table 2 List of carbon-based nanostructures and their biomedical applications discussed in this review
CBN |
Diagnostic application |
Therapeutic application |
Protective coating |
CNT-poly(T) DNA conjugate |
|
DNA separation and gene delivery |
|
Single-walled CNT (SWCNT)-polyethylene glycol conjugate |
|
Neuronal outgrowth |
|
CNT-functionalized gold quantum dots |
Photoacoustic imaging |
|
|
Indium tin oxide (ITO)-treated multi-walled CNTs (MWCNT) |
Ultrasensitive detection of urea in blood |
|
|
Graphene oxide (GO)-chitosan hydrogel scaffolds |
|
Proliferation of pre-osteoblast MC3T3-E1 cells |
|
Reduced graphene oxide (rGO) coated stents |
|
Proliferation of outgrowth endothelial cells |
|
GO |
Biosensing of quadruplex-DNA binding ligands |
|
|
rGO/iron oxide nanocomposite |
Triple-mode sensor for fluorescence, photoacoustic tomography (PAT), and magnetic resonance imaging (MRI) |
|
|
Diamond-like carbon (DLC)-coated metal–polyethylene |
|
|
Hip joint with high wear resistance |
DLC-coated stents |
|
Reduction in thrombocyte adhesion |
Anticorrosive |
Ag-doped DLC |
|
|
Antibacterial |
Si-doped DLC |
|
|
Antifungal |
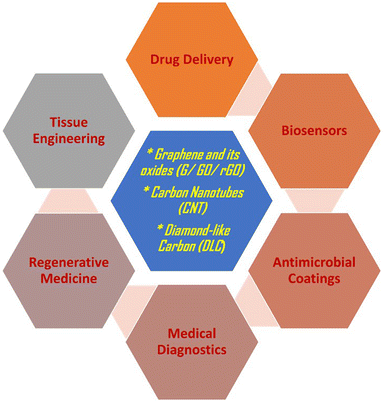 |
| Fig. 2 Advanced biomedical applications of various carbon-based nanostructures that are currently in use. | |
1. Carbon nano tubes (CNTs)
A carbon nanotube (CNT) is a one-dimensional cylindrical structure formed by rolling up of sp2-hybridized graphene sheet.28,29 CNTs are categorized into single-walled (SWCNT) and multi-walled carbon nanotubes (MWCNT) based on the arrangement of the graphene cylinders. CNTs have outstanding mechanical strength, thermal stability, and electrical properties owing to their size and nanostructure.29 Recent studies have shown that CNTs possess superior antioxidant properties against the photodegradation of polymeric materials.30 It is also possible to conjugate a wide range of biomolecules with CNTs, enabling the formation of complex biological structures for target-specific drug delivery in the human body. As such, CNTs have gained much attention for biomedical applications due to their multifunctional capabilities.31
SWCNTs and MWCNTs are synthesized through chemical vapor deposition (CVD), laser ablation, arc discharge techniques, and several other techniques.29,32 MWCNTs are highly crystalline, and weaker van der Waals forces hold together the walls of the cylinders. They find numerous applications in optical and electrical systems due to their versatile structure and properties. As such, the arc discharge method is popularly used to grow MWCNTs due to its ease of synthesis.32 Despite its multi-functionality, one of the significant challenges in using CNTs in biomedical devices is their toxicity. The enhanced surface area of CNTs increases their contact area with cellular membranes, resulting in higher absorption and transportation of toxins.33Fig. 3 shows various cellular responses leading to toxicity in CNT. Furthermore, external factors like the presence of metal impurities and solubilizing agents in the chains also determine the toxicity of CNTs. Besides toxicity, CNTs are highly susceptible to fragmentation in biological fluids.34 However, functionalized CNT is still emerging as an inevitable source of drug carriers due to its advanced drug action and cell penetration capabilities.35 This section is focused on some of the advanced biomedical applications of functionalized CNTs.
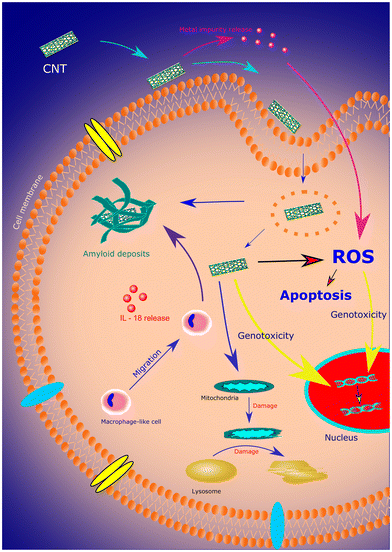 |
| Fig. 3 A schematic representing various cellular responses to CNT leading to toxicity. Reproduced from ref. 36 with permission from Elsevier, copyright 2019. | |
1.1. CNTs in regenerative medicine
The use of CNTs in tissue engineering and regenerative medicine has been explored recently. The superior conducting property and its ability to stimulate cells have enabled the use of CNTs as scaffolds in the human body.37 Furthermore, the functionalization of CNTs has made it possible to stimulate cells for differentiation and proliferation. This approach has enabled the use of CNTs to engineer the growth of new tissue through a cell encapsulation approach.37,38 In one of the studies, Johnson et al. presented a model demonstrating the binding of CNTs to DNA, allowing the effective dispersion of the molecule in aqueous solutions. This work emphasizes applications in CNT solubilization, CNT sorting, biological sensing, ultrafast DNA sequencing, and chemical sensing.39Fig. 4 shows a binding model of a carbon nanotube with DNA in an aqueous and non-aqueous environment. In another study, neurons were incorporated with SWCNTs and polyethylene glycol (PEG) to enable the faster growth of neurites.40 As CNTs can be easily functionalized with biomolecules, conjugation of CNT with endogenous ligands in the central nervous system (CNS) has enabled the reduction in nerve growth factor and consequent extension of neuronal outgrowth.40,41 CNTs have also been explored as promising neuroprosthetic implants.42 Surface-modified CNTs have been considered for use as electrode materials in a neuroprosthetic cochlear implant for spiral ganglion neurons.43 Similarly, Eleftheriou et al. have demonstrated the use of CNTs as electrode materials in retinal implants due to their improved biocompatibility and electrical properties.44 Ostrovsky et al. suggest that the unique combination of surface properties of CNTs, such as charge, polarity, and chemistry, can revolutionize the production of advanced neuroprosthetic implants with advanced neuron electrode coupling.45
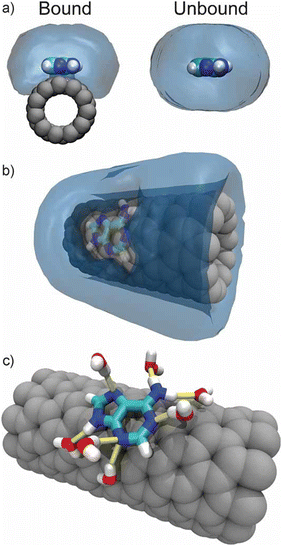 |
| Fig. 4 (a) Water density around bound and unbound DNA (b) water density around CNT showing water excluded from the CNT surface due to the presence of the bound DNA (c) water remains capable of forming hydrogen bonds (yellow) with bound DNA. Reproduced from ref. 39 with permission from John Wiley and Sons, copyright 2009. | |
1.2. Diagnostic and therapeutic applications of CNT
The ease of functionalization of CNTs has transformed biomedical imaging and sensing techniques to image and quantify the constituents of cells, tissues, and organs. Moreover, CNTs are stimulated by applying an external magnetic field, advancing their applications in magnetic resonance imaging (MRI).46 Chen et al. reported that using CNT electrodes in MRI tests resulted in low-distortion images as compared to other electrodes.47 Gong et al. imply that tumor cell imaging can be significantly improved by using CNTs functionalized with gold quantum dots in fluorescent bioimaging probes.46,48 They also suggest that CNTs are an excellent choice for photoacoustic imaging due to their higher absorption capabilities in the NIR regions. In a similar study, it was reported that CNTs functionalized with metal oxide nanoparticles enhanced functionality for bioimaging techniques.49 Minati and others suggest that modified carbon nanotubes are promising materials for both diagnostic and therapeutic applications.50 A study conducted by a team of researchers from the University of Genova reported that biosensors made from CNTs have enhanced sensitivity, faster action, and greater shelf life.51 The bioelectrode formed by treating MWCNTs with indium tin oxide (ITO) was found to be highly sensitive in detecting urea in the bloodstream.52 Guo et al. have developed a DNA-coated CNT-based biosensor as a unique means of detecting proteins with high sensitivity.53Fig. 5 describes the electrochemical activity of the aptamer (DNA)-CNT-based biosensor for detecting target proteins. This approach improved the capabilities of biosensors in medical diagnostics as well as forensic sciences. Recently, Arancibia and the team have shown that CNT-based sensors can be potential tools for sensing therapeutic molecules from different pharmaceutical combinations.54 As such, CNT is emerging as a promising biomaterial due to its unique properties and capabilities.
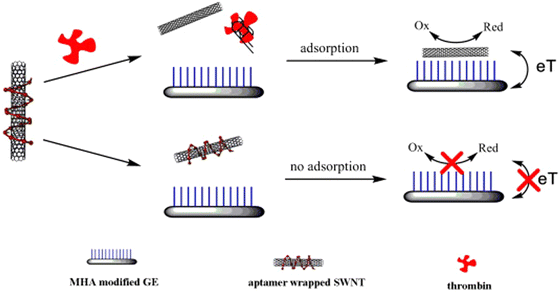 |
| Fig. 5 Electrochemical biosensor strategy for thrombin using aptamer-wrapped SWNT as electrochemical labels. Reproduced from ref. 53 with permission from Elsevier, copyright 2011. | |
2. Graphene and its oxides:
Due to their outstanding mechanical strength and biocompatibility, graphene- and graphene-based carbon materials (referred to as graphene-materials hereafter) are of great interest in the biomedical industry.3 Graphene-coated intravascular implants are known to facilitate the growth of outgrowth endothelial cells, improve the hemocompatibility of biomedical implant material, and control the adverse physiological response from the surroundings.20 However, this approach is not commercially viable for preparing large-scale graphene layers as the current methods of synthesis of graphene are associated with low production rates,55 high thermal budget values,56–59 and contamination.57 Graphene oxide (GO), on the other hand, is straightforward to grow at a large scale without contamination.60 However, GO is insulating, and the oxygen component in the material disturbs its conjugate structure;61 this phenomenon poses complications62 in the biological environment if used as a coating on biomedical implants. Reduced graphene oxide (rGO) overcomes these problems and shows potential for use in biosensors,63 corrosion inhibitors,64 and biomedical implants.65 Moreover, the method of reduction controls the functional properties of rGO, influencing the potential biological applications of these materials.66,67 This section reviews some of the advanced biomedical applications of graphene, GO, and rGO, as represented in Fig. 6.
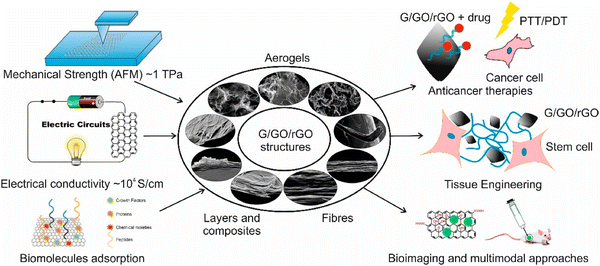 |
| Fig. 6 Schematic showing versatile functionalities of graphene (G), graphene oxide (GO), and reduced graphene oxide (rGO) structures and their typical uses in biomedical devices and systems.68 | |
2.1. Graphene materials in tissue engineering
Composites of graphene are being explored for applications in regenerative medicine, wound healing, and tissue engineering because of their superior mechanical properties and specific biological response.69 Graphene materials are one of the most researched systems for potential reinforcements in tissue engineering scaffolds.70 Recently, it was shown that the tensile strength of polylactide (PLA)/graphene oxide/parathyroid hormone nanofiber membranes was increased up to 110% compared to that of a pure PLA sample, without compromising with the biocompatibility of the system.71 In addition, Zhang et al. reported a significant increase in tensile strength of 132% in GO-incorporated polyvinyl acetate (PVA)-based hydrogels without affecting the cytotoxicity towards osteoblast cells.72 In one of the studies conducted on murine fibrosarcoma cell culture, Depan et al. obtained similar results in graphene-reinforced chitosan films.73 Similarly, dopamine-functionalized graphene oxide nanoplates (GOPD) and gelatin methacrylate (GelMA) were used to develop a printable shear-thinning nanocomposite hydrogel for soft tissue engineering and 3D bioprinting applications. The high mechanical strength of GO imparted an enhancement to the compressive modulus and degradability of the hydrogel.74 Another research reported a substantial development in the adhesion, differentiation, and proliferation of pre-osteoblast MC3T3-E1 cells reinforced with GO–chitosan hydrogel scaffolds.73,75 In a similar study, Nagarajan et al. incorporated antioxidant GO in polycaprolactone scaffolds which were coated with coated with Schwann cell-derived acellular matrix. These scaffolds exhibited antioxidant and remyelination properties; Schwann RSC96 cells seeded on the scaffold showed an increase in cell proliferation as well as significant remyelination.76 The ability to tailor various functionalities on the surface of graphene-based materials has enabled their applications in stem cell engineering. A study conducted by Chen et al. showed that GO-coated scaffolds allow for the direct differentiation of induced pluripotent stem cells into insulin-producing β-cells while maintaining their pluripotency.77 Another research reported improved cell adhesion, differentiation, and proliferation of human mesenchymal stem cells (hMSCs) on graphene- and GO-coated surfaces as compared to glass, silicon, and polydimethylsiloxane substrates.78 Furthermore, graphene foams have shown enhanced differentiation of neural stem cells towards astrocytes.79 McManus et al. envisage that 3D printing of graphene-based inks may replace the complex 3D structures of tissues with enhanced functionalities in the future.80
2.2. Graphene materials as advanced biosensors
Graphene and its oxides are emerging as potential biosensors and platforms for imaging biological entities. Graphene materials are being considered as a potential replacement for existing contrast agents for bioimaging.81,82 Yoo et al. reported the use of graphene/graphene oxide sensors in fluorescent imaging with high quantum yield. They suggest that the biocompatibility of these structures can be easily tuned by functionalizing the surface of graphene materials with various biopolymers.83 Moreover, the properties of the biopolymer/graphene oxide composites can be improved dramatically if the miscibility and compatibility of the components can be controlled.84 For instance, the surface-modified graphene/biopolymer composite showed up to a 95% reduction in the activity of E. coli without affecting the biocompatibility of the composite.85,86 Similarly, graphene oxides are widely studied for their capabilities of sensing several inorganic and organic biomolecules. Fig. 7 shows some of the biosensing and gas sensing applications of graphene materials achieved by hybridizing graphene with different types of nanomaterials (e.g., metallic, polymeric, and carbon materials).87 Gao et al. suggest that graphene oxide can be a simple yet superior replacement for biosensing quadruplex–DNA binding ligands because of its fluorescence-quenching capabilities.88 As such, GO was employed in the fast and sensitive detection of multiple single-stranded DNA molecules.89 Recently, Joshi et al. reported a nonenzymatic electrochemical sensing activity of holey graphene oxide films fabricated via laser processing.90 They show that the sensing capabilities of GO can be controlled by controlling the hole size through laser annealing.90,91 Furthermore, graphene and its oxides are being explored in the fabrication of next-generation multimodal materials for bioimaging that can simultaneously perform more than one function. For instance, a study reported the use of rGO/iron oxide nanocomposite in protodermal therapy and as a triple-mode sensor for photoacoustic tomography (PAT), fluorescence, and magnetic resonance imaging (MRI).92 Similarly, Aburto et al. have discussed the possibility of employing fluorinated graphene oxide in PAT and MRI while performing as a magnetically driven drug carrier.93 Graphene materials can be an excellent replacement for conventional biosensors as they have enabled rapid and sensitive detection of biomolecules at low cost.
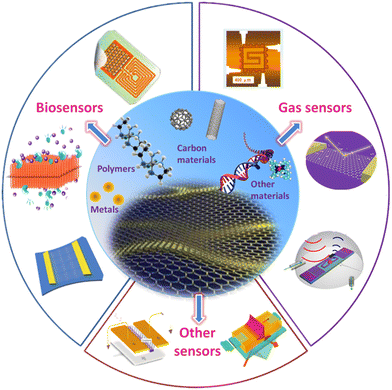 |
| Fig. 7 A schematic showing different kinds of graphene-based composite materials and sensing applications of graphene-materials-based detection devices. Reproduced from ref. 87 with permission from Elsevier, copyright 2017. | |
3. Diamond-like carbon (DLC)
Diamond-like carbon is one of the oldest and most popular forms of CBN materials to be explored for biomedical applications.94 Thomson et al. reported the biocompatibility of DLC coatings in laboratory animals in the early 90s.95 Since then, it has been widely studied for various biomedical applications due to its versatile properties. DLC offers high resistance to corrosion, enhanced surface functionalities, improved tribological properties, antimicrobial activity, and anti-inflammatory action, deeming it suitable for biomedical implants and devices.3,96,97 Furthermore, DLC coatings can be deposited on various types of substrates at relatively low deposition temperatures. The ease of production and unique characteristics of this class of CBN materials make these materials technologically and commercially important.96,98,99 Thus, it has been a popular choice of coating for orthopedic, cardiovascular, and dental implants.
DLC is a form of amorphous carbon with a mixture of sp2- and sp3-hybridized carbon bonds, which is deposited utilizing high-energy carbon species as precursors.100 DLC films can be grown through a number of chemical vapor deposition (CVD) and physical vapor deposition (PVD) techniques such as radio-frequency plasma-enhanced CVD (rf-PECVD), filtered cathodic vacuum arc (FCVA), magnetron sputtering, ion beam sputtering, and pulsed laser deposition (PLD).101 Moreover, Gotzmann et al. have shown that the DLC coatings deposited through these techniques all exhibited biocompatible properties.102 In addition, the properties of DLC can be controlled by alloying it with various metals and non-metals, as the amorphous structure of carbon facilitates straightforward doping of elements.103 DLC films have been doped and alloyed with various elements (e.g., P, Ti, N, Si, F, Cu, and Ag104) as well as various compounds (e.g., ZnO105 and CaO106). The properties of the doped-DLC structures can be modulated by controlling the sp2/sp3 ratio and hydrogen content in the film.100,107
Among them, Si is widely explored as a popular choice of dopants for DLC as it is known to increase the adhesion of the film to the substrate, improve thermal stability, and reduce the internal stresses in the film.108 Consequently, it is possible to grow thicker layers of DLC with better adherence to the substrate.109 Furthermore, Si-doped DLC (Si-DLC) of thickness <100 nm shows a low coefficient of friction and better wear resistance even in a humid environment, as compared to undoped DLC coatings.110 Linder et al. observed that the biocompatibility of DLC films is influenced by dopant atoms.111 Kheradmandfard and others noted that Si/DLC multilayer nanocomposite coatings exhibited no toxicity and showed enhanced cell adhesion in comparison to Ti-29Nb-13Ta-4.6Zr alloy.112 Wang et al. also showed that plasma-treated Si-DLC exhibits improved corrosion resistance, higher interfacial toughness, and biocompatibility in body fluids.113 Similarly, Ag-incorporated DLC has been reported to reduce the internal stresses in the coating while providing antimicrobial activity.114 Titanium-containing diamond-like carbon films that grown on silicon substrates at room temperature with the filtered cathodic vacuum arc technique were also noted to reduce the internal stress and enhance the adhesion characteristics of the coatings.115 All these findings indicate that DLC coatings have a strong potential for applications in the biomedical industry. Some of the applications are reviewed in this section.
3.1. Orthopedic applications
The life of prosthetic joints is severely shortened due to their tendency to wear and corrosion over time. So, DLC coatings have attracted interest in orthopedic applications due to their inertness, improved resistance to wear, low friction coefficient, and biocompatibility.3 In one of the studies, it was demonstrated that the DLC-coated femoral head Ti-6Al-4V alloy showed enhanced wear resistance as compared to the wear rate of uncoated titanium, stainless steel, alumina, and zirconia implants.116 In another study, Tiainen et al. showed that the wear rate of DLC-coated metal–polyethylene hip joints was reduced by 106 times smaller than for metal–metal and metal–polyethylene joints.117 The Dowling group reported improved biocompatibility in DLC-coated hip joints with high sp3 content,118 achieved by doping DLC with silicon. Similarly, Sheeja et al. showed that the corrosion resistance and hardness of Co–Cr–Mo alloy (orthopedic implant material) was significantly increased by coating it with Si-DLC.119 In another study performed by Mitura and others, DLC-coated orthopedic screws showed no signs of corrosion or inflammation even after 52 weeks.120 On the other hand, there are reports on the failure of DLC-coated orthopedic implants. DLC-coated knee joint implants showed excessive wear and spallation against ultra-high molecular weight polyethylene (UHMWPE).121 In a similar study, Taeger et al. found that the DLC-coated Ti-6Al-V femoral head failed faster than the alumina femoral head,122 indicating a need for the development of a biomaterial with enhanced functionalities.
3.2. Cardiovascular applications
One of the primary requirements of a cardiovascular implant is that it should not activate plasma enzymes in order to prevent thrombosis. As such, early studies on DLC-coated heart implants showed a tendency to prevent platelet activation and coagulation of blood.123 Cheng et al. have reported improved hemocompatibility in Ti-doped DLC due to its hydrophobicity and smooth surface.124 In another study, researchers have shown that incorporating Si into DLC improves endothelial cell attachment while reducing the adhesion of platelets at the same time.125 They saw that the results were even better when Si-DLC films were treated with fluorine. All these findings indicated that functionalized DLC coatings could be used for cardiovascular devices like stents and heart valves. In addition, Gutensohn et al. showed that DLC-coated stents were resistant to corrosion, while non-coated stents released metal ions into the human plasma within four days of implantation.126 In another study, Choi and others have shown that catheters coated with silver-doped DLC were highly effective in preventing bacterial infection while preventing the adhesion of platelets.127 They indicated that a greater focus should be made on utilizing DLC coatings in cardiovascular devices owing to their multifunctional capabilities.
3.3. Antimicrobial coatings
Microbial biofilms are ubiquitously increasing the risk of medical device infection.128 As such, treatment related to medical device infections often requires the removal and replacement of devices, increasing healthcare costs and mortality.128,129 Since microbes adhere to the surfaces of medical devices and cause infection, infections can be prevented by coating medical devices with a suitable antimicrobial layer. DLC is widely used as an antibacterial and antifungal coating because of its potential to inhibit the adhesion and growth of microorganisms. Kai-Hung et al. demonstrated that oxygen plasma-treated silicon-incorporated DLC is hydrophilic, has lower negative zeta potential, and showed antifungal activity against Candida albicans (Fig. 8).130 In another study, Robertson and others reported that Ge-doped DLC significantly reduced the formation of P. aeruginosa biofilms on SS316 substrates.131 Bociaga et al. opine that the antimicrobial response of Si-DLC is promising but needs further investigation.132 A recent report on the anti-biofilm properties of prosthetic meshes coated with several metal-containing DLC thin films indicated that all of the materials showed significant antimicrobial activity against five microbial species.133 They propose that modified DLC coatings are suitable for use as biomedical coatings because of their biocompatibility, mechanical strength, and antimicrobial activity.
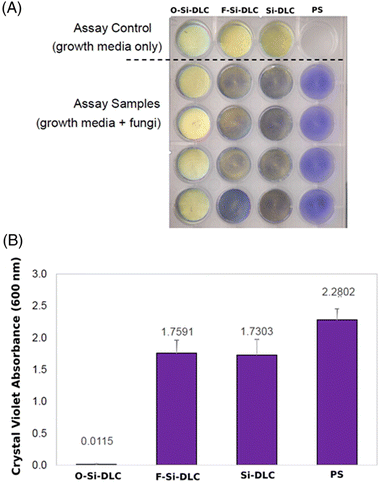 |
| Fig. 8 (A) Images of O-Si-DLC, F-Si-DLC, Si-DLC, and PS with fungal pathogen Candida albicans after crystal violet staining. (B) Crystal violet quantification of C. albicans on O-Si-DLC, F-Si-DLC, Si-DLC, and PS samples using absorbance measured at 600 nm. (O – oxygen, F – fluorine, PS – polystyrene).130 | |
4. Biotoxicity of carbon nanostructures
Despite the extraordinary strength and multifunctional capabilities, biotoxicity limits the performance of many current CBNs for biomedical applications.134 The toxicity and side effects of these materials are attributed to the size, shape, structure, composition, and surface area of the nanostructures.135–138 Srikanth et al. noted that graphene was found to be the most toxic among the carbon nanostructures, with an average toxicity of 52.24%, followed by carbon nanotubes, fullerene, and carbon nanowires.139 The morphology, concentration, and contact duration of these nanostructures with the fibroblast cells were considered to analyze the extent of toxicity in these materials. In another study, it was shown that functionalized CNTs translocated into brain cells, inducing neuroinflammation and cognitive impairment.140 Similarly, Visalli et al. found that MWCNTs caused DNA damage as well as overproduction of reactive oxygen species in neurons, leading to cell inflammation.141 Moreover, GO and fullerenes were shown to alter the gene and protein expression in the liver, leading to increased toxicity in the hepatic cells.142 Several reports described exposure to carbon nanostructures causing nephrotoxicity in both humans and animals. Due to the reduced dispersion of CNTs in biological fluids, human embryonic kidney cells were shown to degrade when exposed to polyethylene-modified CNTs at concentrations above 200 μg ml−1.143 A study on the toxic effects of graphene on kidney cells indicated that GO altered the architecture of the chromatin network, leading to inflammation.144 Carbon nanostructures have also been shown to induce cardiotoxicity, leading to thrombotic events in humans. A recent study on the inhalation exposure to nanodiamonds in mice revealed the disruption of the mitochondrial membrane potential and high levels of glutathione disulfide (GSSG) in the heart tissue.145 Moreover, nanodiamonds and graphene have been shown to reduce vascularization and blood vessel density in chicken embryos.146 Penetration of CBNs into the skin has been reported to have caused contact urticaria, irritation, allergic contact dermatitis, and other cutaneous reactions.147 One of the most controversial issues of CNT structures is their tendency to induce various forms of cancer. Recently, Kasai et al. reported the carcinogenicity of MWCNTs, causing bronchioloalveolar carcinoma.148 Another case study reported recently demonstrated the carcinogenicity of different types of CNTs administered via intraperitoneal injection in rats.149 It was shown that MWCNTs induced higher rates of mesothelioma in comparison to other forms of carbon nanotubes. As such, the extent of toxicity of CBNs, as well as their potential effects on human and animal health, are not currently predictable; comprehensive assessment and documentation of the toxicity of these materials are required. As such, it is essential to develop systematic approaches to analyze the toxicity of carbon nanostructures and their impact on the biological environment.
5. Q-Carbon as an emergent biomaterial
The introduction of carbon-based nanostructures has virtually revolutionized the field of biomaterial research. They exhibit superior functional properties, which enables their potential use in biomedical devices. However, carbon nanostructures are often fabricated via equilibrium growth techniques that are difficult to reproduce with the same precision.55 These techniques employ metal ions as reducing agents, rendering untreated carbon nanostructures toxic to the human body. Moreover, the chemical transfer of carbon nanostructures onto biomedical devices is associated with defects, uneven thickness, and poor adhesion to the substrate.55,150 In addition, equilibrium-based processing techniques are often employed with high-temperature annealing processes, limiting their practical applications in medical devices.151 Although it is widely utilized in biomedical devices, diamond-like carbon (DLC) exhibits several strain-localized regions during tensile loading, reducing the stress-bearing functionality of the film.152 The ultimate tensile strength of Si-incorporated DLC is also known to diminish with increasing temperature, which reduces the utility of DLC-coated implant devices. Moreover, the biotoxicity of some of the CBNs discussed above may require the functionalization of the CBN surface, which may add to the complexity of biomedical device fabrication.134 Thus, even though carbon materials have shown promising features for their applications in biomedical devices, they require continual replacement as the materials degrade over time. However, replacement procedures increase operating costs and put the patient at risk for infection. As such, there is a pressing need for a novel biomaterial that offers a unique combination of mechanical strength, biocompatibility, antimicrobial capabilities, and resistance to corrosion; moreover, it should be straightforward to produce at a reasonable cost.
Quenched-in-carbon or Q-carbon is a newly discovered allotrope of carbon, chemically analogous to diamond-like carbon; this material exhibits extraordinary structural and functional properties. Q-Carbon is comprised of randomly packed tetrahedra with a very high sp3/sp2 ratio at its interface, making it the hardest known material to date.21,153 The Raman spectra and the microstructure of Q-carbon nanostructures are shown in Fig. 9.154 Due to its unique structure, Q-carbon has a higher number density of covalently bonded carbon atoms, making it up to 70% harder than diamond. Thus, it can be used in ultrahard coatings as it is harder, tougher, and more adherent than diamond and DLC structures.155 The Narayan Research Group at NC State University, Raleigh, USA, has developed a process for growing Q-carbon/diamond composite protective coatings for use as a next-generation super-hard coating on practical substrates like sapphire, silicon, glass, steels, and WC–Co.155–158 In addition to its high hardness and toughness, Joshi et al. have demonstrated that Q-carbon is biocompatible, with superior antimicrobial properties.157 They showed that silicon-incorporated Q-carbon inhibited the formation of biofilms up to 80%, making it a promising material for several types of biomedical applications. Q-Carbon is conventionally grown by the pulsed laser deposition and subsequent pulsed laser annealing of DLC thin film using a nanosecond pulsed excimer laser source.155 Recently, Q-carbon growth with wafer-scale integration on substrates as large as 6′′ in diameter was demonstrated; this approach may enable the industrial-scale production of Q-carbon with controlled thickness and other material properties.158
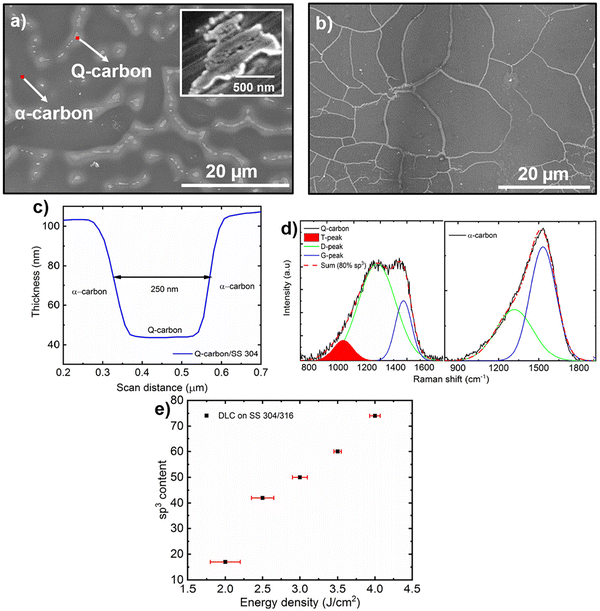 |
| Fig. 9 (a) SEM image demonstrating the formation of Q-carbon on 304 SS, inset shows high-resolution image of Q-carbon in alpha-matrix, (b) SEM image of Q-carbon, (c) surface roughness measurement of Q-carbon, (d) Raman spectra of Q- and alpha-carbon, and (e) correlation between sp3 content of the DLC as a function of laser energy density used during deposition.159 Reproduced from ref. 154 with permission from Elsevier, copyright 2020. | |
In addition to its fascinating structural properties, Q-carbon exhibits robust room-temperature ferromagnetism, with magnetization as high as 20 emu g−1 and Tc of ∼757 K; these characteristics make Q-carbon an excellent candidate for targeted drug delivery applications160.160 Compared to DLC coatings, Q-carbon shows superior field-emission potential due to its unique sp2/sp3 mixture in the emitter and the presence of a semimetallic alpha-carbon matrix in the film.161 Q-Carbon-based field emitters display exceptional performance, featuring hysteresis-free emission, high-emission stability, high current density operation without defect generation, and long-term reliability over successive emission measurements.162 Unlike DLC, Q-carbon is highly resistant to radiation damage, withstanding ion radiations that induce extreme atomic displacements as well as electronic excitations.163 Moreover, the large-scale production of Si-incorporated Q-carbon has enabled the wafer-scale growth of epitaxial diamond films, providing a platform to integrate Si-vacancy nanodiamonds for advanced sensing applications.158 The unique electronic structure of Q-carbon is responsible for its fascinating characteristics, making it an ideal substitute for DLC coatings. Thus, Q-carbon is a truly versatile material with extraordinary properties, finding potential applications in diagnostics and therapeutics. With its ability to overcome the limitations of existing biomaterials, we envision Q-carbon as a superior alternative to the CBNs that are currently in use for a wide range of applications.
Conclusions and future perspective of carbon-based biomaterials
Carbon-based nanostructures have been widely explored in biomedical research due to their outstanding mechanical, thermal, electrical, and optical properties. The unique combination of high aspect ratio and chemical reactivity provided by carbon nanostructures make them suitable candidates for applications in drug delivery and regenerative medicine. Furthermore, CBNs may serve as a useful coating material for biomedical devices owing to their antimicrobial capabilities, outstanding mechanical strength, biocompatibility, and corrosion resistance. Thus, the conjugation of CBNs with biomolecules has led to the development of sophisticated biomedical devices for diagnostic applications. The current review has introduced some of the advanced therapeutic and diagnostic applications of diamond-like carbon, carbon nanotubes, and graphene and its oxides in antimicrobial coatings, regenerative medicine, drug delivery, bioimaging, and biosensing. We critically evaluated the use of various carbon materials and their potential biomedical applications. The toxicology and limitations of existing carbon-based nanostructures were also considered. Finally, we have introduced Q-carbon as a novel CBN and assessed its capabilities as a potential biomaterial.
Future perspectives
Despite advancements in the functionalization of CBNs to improve their biocompatibility and reduce toxicity, concerns regarding biosafety of these materials persist. However, CBNs remain indispensable in the biomedical industry due to their unique combination of robust mechanical strength, chemical inertness, and multifunctional capabilities. We envision that Q-carbon may offer an alternative to conventional CBNs.
Data availability
This review article does not contain new data that have been generated or analyzed by the author. All of the data referenced in this paper are available in the original publications that are cited in the reference list.
Conflicts of interest
There are no conflicts of interest to declare.
Acknowledgements
The authors wish to acknowledge support from National Science Foundation Award #1836767.
References
- B. D. Ratner and S. J. Bryant, Annu. Rev. Biomed. Eng., 2004, 6, 41–75 CrossRef CAS PubMed.
-
J. S. Temenoff and A. G. Mikos, Biomaterials: the intersection of biology and materials science, Pearson/Prentice Hall Upper Saddle River, NJ, USA, 2008, vol. 1 Search PubMed.
- R. K. Roy and K. Lee, J. Biomed. Mater. Res., Part B, 2007, 83, 72–84 CrossRef PubMed.
- N. Huang, P. Yang, Y. Leng, J. Chen, H. Sun, J. Wang, G. Wang, P. Ding, T. Xi and Y. Leng, Biomaterials, 2003, 24, 2177–2187 CrossRef CAS PubMed.
- A. Shah, R. Sinha, N. Hickok and R. Tuan, Bone, 1999, 24, 499–506 CrossRef CAS PubMed.
- M. Auffan, J. Rose, J.-Y. Bottero, G. V. Lowry, J.-P. Jolivet and M. R. Wiesner, Nat. Nanotechnol., 2009, 4, 634–641 CrossRef CAS PubMed.
-
V. S. Patil, S. C. Sharath, S. V. Halse, B. Saraswathi, B. K. Murgunde, N. N. Joshi and M. N. Kalasad, in Handbook of Emerging Materials for Sustainable Energy, ed. N. V. Kulkarni and B. I. Kharissov, Elsevier, 2024, pp. 825–851 Search PubMed.
- S. Kadian, N. Chaulagain, N. N. Joshi, K. M. Alam, K. Cui, K. Shankar, G. Manik and R. J. Narayan, Nanotechnology, 2023, 34, 30LT01 CrossRef PubMed.
- J. Simon, E. Flahaut and M. Golzio, Materials, 2019, 12, 624 CrossRef CAS PubMed.
- C. Cha, S. R. Shin, N. Annabi, M. R. Dokmeci and A. Khademhosseini, ACS Nano, 2013, 7, 2891–2897 CrossRef CAS PubMed.
- X. Ye, Q. Fan, L. Shang and F. Ye, Eng. Regen., 2022, 3, 352–364 Search PubMed.
- L. N. Ramana and V. Agarwal, Nanotechnology, 2021, 32, 475602 CrossRef CAS PubMed.
- L. N. Ramana, L. N. M. Dinh and V. Agarwal, Nanoscale Adv., 2021, 3, 3513–3521 RSC.
- W. Su, H. Wu, H. Xu, Y. Zhang, Y. Li, X. Li and L. Fan, Mater. Chem. Front., 2020, 4, 821–836 RSC.
- M. Villalva and V. Agarwal, Nanomedicine, 2021, 16, 1595–1611 CrossRef CAS PubMed.
- S. Shukla, S. Khanna, S. Sahoo, N. Joshi and R. Narayan, ACS Appl. Bio Mater., 2024, 7, 472–484 CrossRef CAS PubMed.
-
R. Ganjoo, S. Sharma and A. Kumar, Chemically Modified Carbon Nanotubes for Commercial Applications, 2023, pp. 347–364 Search PubMed.
- N. Akiyama, K. D. Patel, E. J. Jang, M. R. Shannon, R. Patel, M. Patel and A. W. Perriman, J. Mater. Chem. B, 2023, 11, 6225–6248 RSC.
- Y. Al-Hadeethi, A. Nagarajan, S. Hanuman, H. Mohammed, A. M. Vetekar, G. Thakur, L. N. M. Dinh, Y. Yao, E. M. Mkawi, M. A. Hussein, V. Agarwal and M. Nune, RSC Adv., 2023, 13, 1392–1401 RSC.
-
N. Joshi, P. Joshi, J. Narayan and R. J. Narayan, (manuscript under preparation).
- J. Narayan and A. Bhaumik, J. Appl. Phys., 2015, 118, 215303 CrossRef.
- K. P. Loh, D. Ho, G. N. C. Chiu, D. T. Leong, G. Pastorin and E. K.-H. Chow, Adv. Mater., 2018, 30, 1802368 CrossRef PubMed.
- N. Joshi, S. Azizi Machekposhti and R. J. Narayan, JID Innovations, 2023, 3, 100225 CrossRef PubMed.
- S. Shukla, S. A. Machekposhti, N. Joshi, P. Joshi and R. J. Narayan, Small Sci., 2023, 3, 2200087 CrossRef CAS.
- S. Shukla, N. Narasimhachar Joshi, S. Kadian and R. J. Narayan, ACS Appl. Bio Mater., 2024, 7, 5382–5396 CrossRef CAS PubMed.
- K. K. Jain, Clin. Chem., 2007, 53, 2002–2009 CrossRef CAS PubMed.
- S. Adorinni, M. C. Cringoli, S. Perathoner, P. Fornasiero and S. Marchesan, Appl. Sci., 2021, 11, 2490, DOI:10.3390/app11062490.
- C. L. Kane and E. Mele, Phys. Rev. Lett., 1997, 78, 1932 CrossRef CAS.
- S. Iijima, Nature, 1991, 354, 56–58 CrossRef CAS.
- P. Watts, P. Fearon, W. Hsu, N. Billingham, H. Kroto and D. Walton, J. Mater. Chem., 2003, 13, 491–495 RSC.
- S. Polizu, O. Savadogo, P. Poulin and L. Yahia, J. Nanosci. Nanotechnol., 2006, 6, 1883–1904 CrossRef CAS PubMed.
- T. W. Ebbesen and P. M. Ajayan, Nature, 1992, 358, 220–222 CrossRef CAS.
- L. Lacerda, S. Raffa, M. Prato, A. Bianco and K. Kostarelos, Nano Today, 2007, 2, 38–43 CrossRef.
- A. P. Francis and T. Devasena, Toxicol. Ind. Health, 2018, 34, 200–210 CrossRef CAS PubMed.
- D. Pantarotto, C. D. Partidos, R. Graff, J. Hoebeke, J.-P. Briand, M. Prato and A. Bianco, J. Am. Chem. Soc., 2003, 125, 6160–6164 CrossRef CAS PubMed.
- V. R. Raphey, T. K. Henna, K. P. Nivitha, P. Mufeedha, C. Sabu and K. Pramod, Mater. Sci. Eng., C, 2019, 100, 616–630 CrossRef CAS PubMed.
-
S. Bosi, L. Ballerini and M. Prato, Making and exploiting fullerenes, graphene, and carbon nanotubes, 2014, pp. 181–204 Search PubMed.
- A. Paul, A. Hasan, H. A. Kindi, A. K. Gaharwar, V. T. Rao, M. Nikkhah, S. R. Shin, D. Krafft, M. R. Dokmeci and D. Shum-Tim, ACS Nano, 2014, 8, 8050–8062 CrossRef CAS PubMed.
- R. R. Johnson, A. C. Johnson and M. L. Klein, Small, 2010, 6, 31–34 CrossRef CAS PubMed.
- E. B. Malarkey, R. C. Reyes, B. Zhao, R. C. Haddon and V. Parpura, Nano Lett., 2008, 8, 3538–3542 CrossRef CAS PubMed.
- K. Matsumoto, C. Sato, Y. Naka, A. Kitazawa, R. L. Whitby and N. Shimizu, J. Biosci. Bioeng., 2007, 103, 216–220 CrossRef CAS PubMed.
- T. J. Webster, M. C. Waid, J. L. McKenzie, R. L. Price and J. U. Ejiofor, Nanotechnology, 2004, 15, 009 CrossRef PubMed.
- N. Burblies, J. Schulze, H.-C. Schwarz, K. Kranz, D. Motz, C. Vogt, T. Lenarz, A. Warnecke and P. Behrens, PLoS One, 2016, 11, e0158571 CrossRef PubMed.
- C. G. Eleftheriou, J. B. Zimmermann, H. D. Kjeldsen, M. David-Pur, Y. Hanein and E. Sernagor, Biomaterials, 2017, 112, 108–121 CrossRef CAS PubMed.
- S. Ostrovsky, S. Hahnewald, R. Kiran, P. Mistrik, R. Hessler, A. Tscherter, P. Senn, J. Kang, J. Kim and M. Roccio, RSC Adv., 2016, 6, 41714–41723 RSC.
- H. Gong, R. Peng and Z. Liu, Adv. Drug Delivery Rev., 2013, 65, 1951–1963 CrossRef CAS PubMed.
- G. Chen, B. Dodson, F. Johnson, I. Hancu, E. Fiveland, W. Zhang, C. Galligan, C. Puleo, R. C. Davis and J. Ashe, J. Magn. Reson., 2018, 295, 72–79 CrossRef CAS PubMed.
- A. Tan, L. Yildirimer, J. Rajadas, H. De La Peña, G. Pastorin and A. Seifalian, Nanomedicine, 2011, 6, 1101–1114 CrossRef PubMed.
- J. H. Choi, F. T. Nguyen, P. W. Barone, D. A. Heller, A. E. Moll, D. Patel, S. A. Boppart and M. S. Strano, Nano Lett., 2007, 7, 861–867 CrossRef CAS PubMed.
- L. Minati, V. Antonini, M. Dalla Serra and G. Speranza, Langmuir, 2012, 28, 15900–15906 CrossRef CAS PubMed.
- G. Ijeomah, F. Obite and O. Rahman, Int. J. Nano Biomater., 2016, 6, 83–109 CrossRef CAS.
- K. K. Reza, S. Srivastava, S. K. Yadav and A. Biradar, Mater. Lett., 2014, 126, 126–130 CrossRef.
- K. Guo, Y. Wang, H. Chen, J. Ji, S. Zhang, J. Kong and B. Liu, Electrochem. Commun., 2011, 13, 707–710 CrossRef CAS.
- V. Arancibia, J. Penagos-Llanos, E. Nagles, O. García-Beltrán and J. J. Hurtado, J. Pharm. Anal., 2019, 9, 62–69 CrossRef PubMed.
- L. Lin, H. Peng and Z. Liu, Nat. Mater., 2019, 18, 520–524 CrossRef CAS PubMed.
- L.-S. Lin, W. Bin-Tay, Y.-R. Li, Z. Aslam, A. Westwood and R. Brydson, Carbon, 2020, 167, 307–321 CrossRef CAS.
- X. Yang and M. Yan, Nano Res., 2020, 13, 599–610 CrossRef CAS.
- Y. Dan, Y. Lu, N. J. Kybert, Z. Luo and A. T. C. Johnson, Nano Lett., 2009, 9, 1472–1475 CrossRef CAS PubMed.
- G. R. Yazdi, F. Akhtar, I. G. Ivanov, S. Schmidt, I. Shtepliuk, A. Zakharov, T. Iakimov and R. Yakimova, Appl. Surf. Sci., 2019, 486, 239–248 CrossRef CAS.
- J. I. Paredes, S. Villar-Rodil, A. Martínez-Alonso and J. M. D. Tascón, Langmuir, 2008, 24, 10560–10564 CrossRef CAS PubMed.
- K. Spilarewicz-Stanek, A. Kisielewska, J. Ginter, K. Bałuszyńska and I. Piwoński, RSC Adv., 2016, 6, 60056–60067 RSC.
- A. B. Seabra, A. J. Paula, R. de Lima, O. L. Alves and N. Durán, Chem. Res. Toxicol., 2014, 27, 159–168 Search PubMed.
- M. Zhou, Y. Zhai and S. Dong, Anal. Chem., 2009, 81, 5603–5613 CrossRef CAS PubMed.
- D. S. Chauhan, M. A. Quraishi, K. R. Ansari and T. A. Saleh, Prog. Org. Coat., 2020, 147, 105741 CrossRef CAS.
- Y. Bai, Y. Bai, J. Gao, W. Ma, J. Su and R. Jia, J. Alloys Compd., 2016, 688, 657–667 CrossRef CAS.
- V. Agarwal and P. B. Zetterlund, Chem. Eng. J., 2021, 405, 127018 CrossRef CAS.
- J. Jagiełło, A. Chlanda, M. Baran, M. Gwiazda and L. Lipińska, Nanomaterials, 2020, 10, 1846, DOI:10.3390/nano10091846.
- K. Tadyszak, J. K. Wychowaniec and J. Litowczenko, Nanomaterials, 2018, 8, 944, DOI:10.3390/nano8110944.
- Q. Zhang, Z. Wu, N. Li, Y. Pu, B. Wang, T. Zhang and J. Tao, Mater. Sci. Eng., C, 2017, 77, 1363–1375 CrossRef CAS PubMed.
- W. Xue, J. Du, Q. Li, Y. Wang, Y. Lu, J. Fan, S. Yu and Y. Yang, Tissue Eng., Part B, 2022, 28, 1121–1136 CrossRef CAS PubMed.
- F. Fei, H. Yao, Y. Wang and J. Wei, Int. J. Mol. Sci., 2023, 24, 5799, DOI:10.3390/ijms24065799.
- L. Zhang, Z. Wang, C. Xu, Y. Li, J. Gao, W. Wang and Y. Liu, J. Mater. Chem., 2011, 21, 10399–10406 RSC.
- D. Depan, B. Girase, J. S. Shah and R. D. K. Misra, Acta Biomater., 2011, 7, 3432–3445 CrossRef CAS PubMed.
- M. Najarzadegan, S. Nouri Khorasani, S. Khalili, M. R. Molavian, S. Saleki, A. Kakapour and M. Hafezi, Eur. Polym. J., 2023, 195, 112204 CrossRef CAS.
- L. Zhang, X. Li, C. Shi, G. Ran, Y. Peng, S. Zeng and Y. He, Stem Cells Int., 2021, 2021, 5594370 Search PubMed.
- A. Nagarajan, N. Rizwana, M. Abraham, M. Bhat, A. Vetekar, G. Thakur, U. Chakraborty, V. Agarwal and M. Nune, J. Mater. Sci.: Mater. Med., 2023, 34, 49 CrossRef CAS PubMed.
- G.-Y. Chen, D. W.-P. Pang, S.-M. Hwang, H.-Y. Tuan and Y.-C. Hu, Biomaterials, 2012, 33, 418–427 CrossRef CAS PubMed.
- L.-A. Turner and M. J. Dalby, Biomater. Sci., 2014, 2, 1574–1594 RSC.
- R. Guo, J. Li, C. Chen, M. Xiao, M. Liao, Y. Hu, Y. Liu, D. Li, J. Zou, D. Sun, V. Torre, Q. Zhang, R. Chai and M. Tang, Colloids Surf., B, 2021, 200, 111590 CrossRef CAS PubMed.
- D. McManus, S. Vranic, F. Withers, V. Sanchez-Romaguera, M. Macucci, H. Yang, R. Sorrentino, K. Parvez, S.-K. Son, G. Iannaccone, K. Kostarelos, G. Fiori and C. Casiraghi, Nat. Nanotechnol., 2017, 12, 343–350 CrossRef CAS PubMed.
-
J. Lin, Y. Huang and P. Huang, in Biomedical Applications of Functionalized Nanomaterials, ed. B. Sarmento and J. das Neves, Elsevier, 2018, pp. 247–287 Search PubMed.
- N. Joshi, S. Shukla and R. J. Narayan, Transl. Biophotonics, 2022, 4, e202200001 CrossRef CAS PubMed.
- J. M. Yoo, J. H. Kang and B. H. Hong, Chem. Soc. Rev., 2015, 44, 4835–4852 RSC.
- N. Asim, M. S. Su’ait, M. Badiei, M. Mohammad, M. Akhtaruzzaman, A. Rajabi, N. Amin and M. J. Ghazali, Nanotechnol. Rev., 2022, 11, 1525–1554 CrossRef CAS.
- S. Joshi, R. Siddiqui, P. Sharma, R. Kumar, G. Verma and A. Saini, Sci. Rep., 2020, 10, 9441 CrossRef CAS PubMed.
- R. Teixeira-Santos, S. Belo, R. Vieira, F. J. M. Mergulhão and L. C. Gomes, Biomolecules, 2023, 13, 1571, DOI:10.3390/biom13111571.
- X. Yu, W. Zhang, P. Zhang and Z. Su, Biosens. Bioelectron., 2017, 89, 72–84 CrossRef CAS PubMed.
- Y. Gao, J. Tian, X. Zhang, B. Qiao, Y. Cao, X. Wang and Q. Wu, Analyst, 2020, 145, 1190–1194 RSC.
- B. Liu, Z. Sun, X. Zhang and J. Liu, Anal. Chem., 2013, 85, 7987–7993 CrossRef CAS PubMed.
- P. Joshi, S. Shukla, S. Gupta, P. R. Riley, J. Narayan and R. Narayan, ACS Appl. Mater. Interfaces, 2022, 14, 37149–37160 CrossRef CAS PubMed.
- P. Joshi, S. Shukla, S. Gupta, N. Joshi, J. Narayan and R. Narayan, MRS Commun., 2023, 13, 554–560 CrossRef CAS.
- X. Ma, H. Tao, K. Yang, L. Feng, L. Cheng, X. Shi, Y. Li, L. Guo and Z. Liu, Nano Res., 2012, 5, 199–212 CrossRef CAS.
- R. Romero-Aburto, T. N. Narayanan, Y. Nagaoka, T. Hasumura, T. M. Mitcham, T. Fukuda, P. J. Cox, R. R. Bouchard, T. Maekawa, D. S. Kumar and S. V. Torti, Adv. Mater., 2013, 25, 5632–5637 CrossRef CAS PubMed.
- G. Dearnaley and J. H. Arps, Surf. Coat. Technol., 2005, 200, 2518–2524 CrossRef CAS.
- L. Anne Thomson, F. C. Law, N. Rushton and J. Franks, Biomaterials, 1991, 12, 37–40 CrossRef PubMed.
- F. Pan, M. Khan, A. H. Ragab, E. Javed, H. A. Alsalmah, I. Khan, T. Lei, A. Hussain, A. Mohamed, A. Zada and M. Z. Ansari, Mater. Des., 2023, 233, 112179 CrossRef CAS.
-
S. Angela, T.-C. You, D. Pham, T.-N. Le and W.-W. Hsiao, Nanodiamonds in Analytical and Biological Sciences, 2023, pp. 52–72 Search PubMed.
- N. A. Sánchez, C. Rincón, G. Zambrano, H. Galindo and P. Prieto, Thin Solid Films, 2000, 373, 247–250 CrossRef.
-
S. Angela, R. Hsin, S.-W. Lu, L. Trong-Nghia and W.-W. Hsiao, Nanodiamonds in Analytical and Biological Sciences, 2023, pp. 171–197 Search PubMed.
- C. A. Love, R. B. Cook, T. J. Harvey, P. A. Dearnley and R. J. K. Wood, Tribol. Int., 2013, 63, 141–150 CrossRef CAS.
- A. Grill, Diamond Relat. Mater., 1999, 8, 428–434 CrossRef CAS.
- G. Gotzmann, J. Beckmann, C. Wetzel, B. Scholz, U. Herrmann and J. Neunzehn, Surf. Coat. Technol., 2017, 311, 248–256 CrossRef CAS.
- M. Kalin and J. Vižintin, Thin Solid Films, 2006, 515, 2734–2747 CrossRef CAS.
- Y.-Y. Chang, D.-Y. Wang and W. Wu, Thin Solid Films, 2002, 420–421, 241–247 CrossRef CAS.
- S. Buchegger, A. Kamenac, S. Fuchs, R. Herrmann, P. Houdek, C. Gorzelanny, A. Obermeier, S. Heller, R. Burgkart, B. Stritzker, A. Wixforth and C. Westerhausen, Sci. Rep., 2019, 9, 17246 CrossRef PubMed.
- A. Dorner-Reisel, C. Schürer, C. Nischan, O. Seidel and E. Müller, Thin Solid Films, 2002, 420–421, 263–268 CrossRef CAS.
- A. Erdemir and C. Donnet, J. Phys. D: Appl. Phys., 2006, 39, R311 CrossRef CAS.
- J. Damasceno, S. Camargo, F. Freire and R. Carius, Surf. Coat. Technol., 2000, 133–134, 247–252 CrossRef CAS.
-
A. Hotta and T. Hasebe, in Thin Films and Coatings in Biology, ed. S. Nazarpour, Springer, Netherlands, Dordrecht, 2013, pp. 171–228 Search PubMed.
- W.-J. Wu and M.-H. Hon, Surf. Coat. Technol., 1999, 111, 134–140 CrossRef CAS.
- S. Linder, W. Pinkowski and M. Aepfelbacher, Biomaterials, 2002, 23, 767–773 CrossRef CAS PubMed.
- M. Kheradmandfard, O. V. Penkov, S. F. Kashani-Bozorg, J. S. Lee, C.-L. Kim, M. Khadem, S.-W. Cho, A. Z. Hanzaki and D.-E. Kim, Ceram. Int., 2022, 48, 17376–17384 CrossRef CAS.
- M. Wang, Y. Zhao, R. Z. Xu, M. Zhang, R. K. Y. Fu and P. K. Chu, Mater. Sci. Forum, 2014, 783–786, 1396–1401 CAS.
- P. Písařík, M. Jelínek, J. Remsa, J. Mikšovský, J. Zemek, K. Jurek, Š. Kubinová, J. Lukeš and J. Šepitka, Mater. Sci. Eng., C, 2017, 77, 955–962 CrossRef PubMed.
- X. Ding, B. K. Tay, S. P. Lau, P. Zhang and X. T. Zeng, Thin Solid Films, 2002, 408, 183–187 CrossRef CAS.
- D.-H. Kim, H.-E. Kim, K.-R. Lee, C.-N. Whang and I.-S. Lee, Mater. Sci. Eng., C, 2002, 22, 9–14 CrossRef.
- V.-M. Tiainen, Diamond Relat. Mater., 2001, 10, 153–160 CrossRef CAS.
- D. P. Dowling, P. V. Kola, K. Donnelly, T. C. Kelly, K. Brumitt, L. Lloyd, R. Eloy, M. Therin and N. Weill, Diamond Relat. Mater., 1997, 6, 390–393 CrossRef CAS.
- D. Sheeja, B. Tay, S. Lau and L. Nung, Surf. Coat. Technol., 2001, 146–147, 410–416 CrossRef CAS.
- E. Mitura, S. Mitura, P. Niedzielski, Z. Has, R. Wolowiec, A. Jakubowski, J. Szmidt, A. Sokołowska, P. Louda, J. Marciniak and B. Koczy, Diamond Relat. Mater., 1994, 3, 896–898 CrossRef CAS.
- A. Escudeiro, M. A. Wimmer, T. Polcar and A. Cavaleiro, Tribol. Int., 2015, 89, 97–104 CrossRef CAS.
- G. Taeger, L. E. Podleska, B. Schmidt, M. Ziegler and D. Nast-Kolb, Materialwiss. Werkstofftech., 2003, 34, 1094–1100 CrossRef CAS.
- I. Dion, X. Roques, H. Baquey, E. Baudet, B. Basse Cathalinat and N. More, Bio-Med. Mater. Eng., 1993, 3, 51–55 CAS.
- H.-C. Cheng, S.-Y. Chiou, C.-M. Liu, M.-H. Lin, C.-C. Chen and K.-L. Ou, J. Alloys Compd., 2009, 477, 931–935 CrossRef CAS.
- A. A. Ogwu, T. I. T. Okpalugo, N. Ali, P. D. Maguire and J. A. D. McLaughlin, J. Biomed. Mater. Res., Part B, 2008, 85B, 105–113 CrossRef CAS PubMed.
- K. Gutensohn, C. Beythien, J. Bau, T. Fenner, P. Grewe, R. Koester, K. Padmanaban and P. Kuehnl, Thromb. Res., 2000, 99, 577–585 CrossRef CAS PubMed.
- H. W. Choi, R. H. Dauskardt, S.-C. Lee, K.-R. Lee and K. H. Oh, Diamond Relat. Mater., 2008, 17, 252–257 CrossRef CAS.
- M. Habash and G. Reid, J. Clin. Pharmacol., 1999, 39, 887–898 CrossRef CAS PubMed.
- A. MK, A. AV, M. CM, V. P. Reddy, B. Shetty and N. Joshi, J. Indian Orthod. Soc., 2023, 57, 215–223 CrossRef.
- K.-H. Yang, P. Riley, K. B. Rodenhausen, S. A. Skoog, S. J. Stafslien, L. Vanderwal and R. J. Narayan, Int. J. Appl. Ceram. Technol., 2022, 19, 2545–2555 CAS.
- S. N. Robertson, D. Gibson, W. G. MacKay, S. Reid, C. Williams and I. Birney, Surf. Coat. Technol., 2017, 314, 72–78 CrossRef CAS.
- D. Bociaga, A. Sobczyk-Guzenda, W. Szymanski, A. Jedrzejczak, A. Jastrzebska, A. Olejnik and K. Jastrzebski, Appl. Surf. Sci., 2017, 417, 23–33 CrossRef CAS.
- E. M. Cazalini, W. Miyakawa, G. R. Teodoro, A. S. S. Sobrinho, J. E. Matieli, M. Massi and C. Y. Koga-Ito, J. Mater. Sci.: Mater. Med., 2017, 28, 97 CrossRef PubMed.
- M. Farmand, F. Jahanpeyma, A. Gholaminejad, M. Azimzadeh, F. Malaei and N. Shoaie, 3 Biotech., 2022, 12, 159 CrossRef PubMed.
- T. R. Naik, N. Joshi, S. Shivashankar and P. Bindu, J. Mater. NanoSci., 2019, 6, 67–72 CAS.
- N. Joshi, S. A. Shivashankar and R. Narayan, Nano Express, 2023, 4, 035009 CrossRef CAS.
- N. N. Joshi and S. A. Shivashankar, MRS Adv., 2024 DOI:10.1557/s43580-024-00943-9.
- R. Madannejad, N. Shoaie, F. Jahanpeyma, M. H. Darvishi, M. Azimzadeh and H. Javadi, Chem.-Biol. Interact., 2019, 307, 206–222 CrossRef CAS PubMed.
-
M. Srikanth, H. Misak, S.-Y. Yang and R. Asmatulu, Effects of morphology, concentration and contact duration of carbon-based nanoparticles on cytotoxicity of L929 cells, ASME International Mechanical Engineering Congress and Exposition, American Society of Mechanical Engineers, 2015, vol. 57571, p. V014T11A027 Search PubMed.
- C. Bussy, K. T. Al-Jamal, J. Boczkowski, S. Lanone, M. Prato, A. Bianco and K. Kostarelos, ACS Nano, 2015, 9, 7815–7830 CrossRef CAS PubMed.
- G. Visalli, M. Currò, D. Iannazzo, A. Pistone, M. P. Ciarello, G. Acri, B. Testagrossa, M. P. Bertuccio, R. Squeri and A. Di Pietro, Environ. Toxicol. Pharmacol., 2017, 56, 121–128 CrossRef CAS PubMed.
- A. Patlolla, J. Rondalph, A. Kumari and P. Tchounwou, Int. J. Environ. Res. Public Health, 2016, 13, 380 CrossRef PubMed.
- S. Yu, X. Su, J. Du, J. Wang, Y. Gao, L. Zhang, L. Chen, Y. Yang and X. Liu, New Carbon Mater., 2018, 33, 36–45 CrossRef CAS.
- Y. Sun, H. Dai, S. Chen, M. Xu, X. Wang, Y. Zhang, S. Xu, A. Xu, J. Weng and S. Liu, Nanotoxicology, 2018, 12, 117–137 CrossRef CAS PubMed.
- Y. Khosravi, A. Salimi, J. Pourahmad, P. Naserzadeh and E. Seydi, Xenobiotica, 2018, 48, 860–866 CrossRef CAS PubMed.
- M. Wierzbicki, E. Sawosz, M. Grodzik, A. Hotowy, M. Prasek, S. Jaworski, F. Sawosz and A. Chwalibog, Int. J. Nanomed., 2013, 3427–3435 Search PubMed.
- N. Ali and F. Oehme, Vet. Hum. Toxicol., 1992, 34, 428–437 CAS.
- T. Kasai, Y. Umeda, M. Ohnishi, T. Mine, H. Kondo, T. Takeuchi, M. Matsumoto and S. Fukushima, Part. Fibre Toxicol., 2015, 13, 1–19 CrossRef PubMed.
- Y. Sakamoto, M. Hojo, Y. Kosugi, K. Watanabe, A. Hirose, A. Inomata, T. Suzuki and D. Nakae, J. Toxicol. Sci., 2018, 43, 587–600 CrossRef CAS PubMed.
- S. Gupta and J. Narayan, Carbon, 2019, 153, 663–673 CrossRef CAS.
- W. Huang, Y. Wang, G. Luo and F. Wei, Carbon, 2003, 41, 2585–2590 CrossRef CAS.
- Y. Yu, W. Tang, Z. Liu and L. Bai, Diamond Relat. Mater., 2020, 110, 108099 CrossRef CAS.
- J. Narayan, S. Sundar Sahoo, N. Joshi and R. Narayan, Mater. Res. Lett., 2023, 11, 688–696 CrossRef CAS.
- P. Joshi, S. Gupta, A. Haque and J. Narayan, Diamond Relat. Mater., 2020, 104, 107742 CrossRef CAS.
- R. Sachan, S. Gupta and J. Narayan, ACS Appl. Mater. Interfaces, 2019, 12, 1330–1338 CrossRef PubMed.
- J. Narayan, S. Gupta, A. Bhaumik, R. Sachan, F. Cellini and E. Riedo, MRS Commun., 2018, 8, 428–436 CrossRef CAS.
- N. Joshi, S. Shukla, N. Khosla, L. Vanderwal, S. Stafslien, J. Narayan and R. J. Narayan, Thin Solid Films, 2024, 791, 140227 CrossRef CAS.
- P. R. Riley, P. Joshi, N. Khosla, R. J. Narayan and J. Narayan, Carbon, 2022, 196, 972–978 CrossRef CAS.
- J. Narayan, A. Bhaumik, S. Gupta, P. Joshi, P. Riley and R. J. Narayan, Carbon, 2021, 176, 558–568 CrossRef CAS.
- A. Bhaumik, S. Nori, R. Sachan, S. Gupta, D. Kumar, A. K. Majumdar and J. Narayan, ACS Appl. Nano Mater., 2018, 1, 807–819 CrossRef CAS.
- J. Narayan, A. Bhaumik, S. Gupta, A. Haque and R. Sachan, Mater. Res. Lett., 2018, 6, 353–364 CrossRef CAS.
- A. Haque and J. Narayan, Diamond Relat. Mater., 2018, 86, 71–78 CrossRef CAS.
- J. Narayan, P. Joshi, J. Smith, W. Gao, W. J. Weber and R. J. Narayan, Carbon, 2022, 186, 253–261 CrossRef CAS.
|
This journal is © The Royal Society of Chemistry 2024 |
Click here to see how this site uses Cookies. View our privacy policy here.