DOI:
10.1039/D4MA00514G
(Communication)
Mater. Adv., 2024,
5, 6373-6377
A perspective on contact-electro-catalysis based on frontier molecular orbitals†
Received
20th May 2024
, Accepted 5th July 2024
First published on 16th July 2024
Abstract
The contribution from frontier molecular orbitals to contact-electro-catalysis is discussed. Less energy is required for transferring electrons to polymers with a lower LUMO during contact-electrification, thus facilitating the subsequent formation of reactive oxygen species. We expect that a quantified contact-electro-catalytic diagram could be established based on this study in the future.
Introduction
The contact-electrification (CE) effect is ubiquitous when a liquid comes into contact with a solid,1–3 and electrons have been proven to be the primary charge carrier for the majority of cases of CE.4–6 In virtue of the electron transfer process during CE, contact-electro-catalysis (CEC) has been proposed as a significant supplement to existing catalytic strategies.7–9 Polymers are the most commonly used catalysts for CEC,10–12 and the efficiency of CEC varies drastically according to the polymers employed.13,14 These variations can be partly explained by a recent study focusing on the functional groups of the polymers.15 However, more insights into this process are required to illustrate several vital phenomena, such as the speed threshold for initiating CEC and higher reaction rate under an elevated energy supply.13,16 Based on the close relationship among the catalytic process, triboelectric materials, and energy input, we surmise that a more complete theory could be established by taking the energy state of the transferred electrons into consideration.
Here, exemplified by five representative polymers and their CE with deionized water, we demonstrate that the frontier molecular orbitals of polymers may play an essential role in the electron exchange process. Specifically, polymers with a lower lowest unoccupied molecular orbital (LUMO) exhibit higher CE ability upon contact with water, suggesting that the LUMO may be responsible for receiving the transferred electrons during CE. Further investigations into vertical attachment energy (VAE) verify that less energy is required for transferring electrons to polymers with a lower LUMO. Based on the dominant role of LUMO, an energy diagram is thus proposed to describe the electron transfer process during the CEC. Sufficient energy is necessary for transferring electrons to the LUMO of polymers, and more electrons will be exchanged to the LUMO if higher energy is supplied. For a given energy input, electrons could be more facilely obtained by polymers that exhibit a lower LUMO, which is beneficial for producing hydroxyl radicals. Subsequently, the formation of superoxide radicals could also be promoted, since more electrons are accumulated on such polymers. Therefore, CEC could be significantly enhanced by increasing the energy supply or decreasing the LUMO of the employed materials. More importantly, we expect that this study could lead to the establishment of a contact-electro-catalytic diagram, facilitating the selection of suitable materials and mechanical stimulation for catalyzing target reactions.
Main text
Evaluation of transferred charges during CE between polymers and DI water
The CE between deionized (DI) water and polymers is a representative case of liquid–solid contact-electrification (L–S CE),17–19 which is also the fundamental for contact-electro-catalysis (CEC). To clearly elucidate the impact of solids on L–S CE, we selected five representative polymers that have the same main chain but different side chains. Specifically, polyethylene (PE) with hydrogen groups, polypropylene (PP) with methyl groups, polyvinyl chloride (PVC) with chlorine groups, polyvinylidene fluoride (PVDF) with bifluoride groups, and polytetrafluoroethylene (PTFE) with tetrafluoro groups were investigated; their chemical structures are demonstrated in Fig. 1a. Using a pristine polymer film with an area of 20 cm2 and a 50 μm thickness as the tribo-layer, a single-electrode triboelectric nanogenerator (SE-TENG) was devised for quantifying the CE ability of polymers upon contact with DI water. Fig. 1b illustrates the architecture and operating mechanism of the SE-TENG. The measured results shown in Fig. 1c indicate that the quantity of charges transferred varies drastically depending on the side chain of the employed polymers. The density of functional groups in the side chain could greatly affect the CE performance of the polymers. For example, the highest transferred charge (∼50 nC) is achieved for PTFE, which is characterized by tetrafluoro groups in the side chains. In contrast, only ∼10 nC of transferred charge was obtained for PVDF, which has only two fluorine atoms in the side chain of each monomer. Additionally, the type of side-chain atoms significantly contributes to the CE ability of polymers. The amount of charges transferred in non-fluorine polymers (i.e., PE, PP and PVC) is far less than for PVDF and PTFE. The slight differences among the CE performances of PE, PP, and PVC could also be ascribed to the varying electron-withdrawing (EW) ability of the –H, –CH3, and –Cl groups in the side chain.15 These experimental conclusions are not only indispensable for selecting suitable materials for CE-based applications but also lay the foundation for further theoretical analysis of CE.
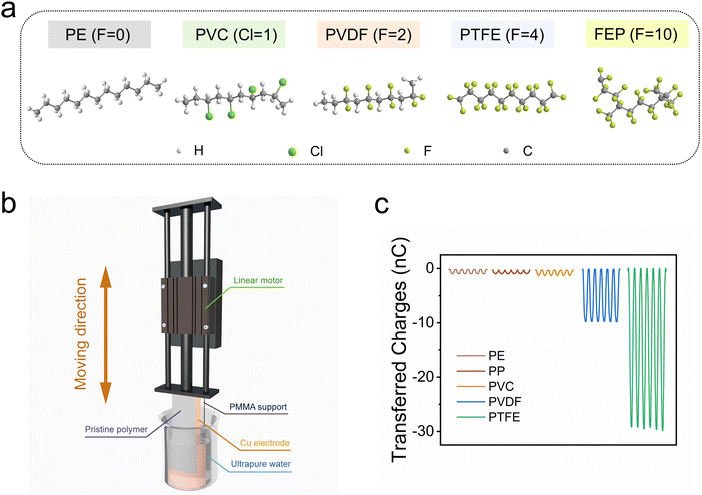 |
| Fig. 1 Experimental measurement for quantifying the CE ability of different polymers. (a) Chemical structure of the utilized polymers. (b) Schematic illustration of the evaluation of the CE ability of the polymers using a single-electrode mode triboelectric nanogenerator (SE-TENG) that repeatedly comes into contact with DI water. (c) Quantity of charges transfer measured when the different polymers were used for CE with DI water. | |
Theoretical investigations of the frontier molecular orbitals and their impact on CE
To establish a unified theory for illustrating the discrepancies in the CE abilities of the distinct polymers, we calculated the energy levels of their frontier molecular orbitals (FMO), which are believed to be responsible for the interaction of the polymers with surrounding molecules.20,21 The optimized structures of the investigated polymers are exhibited in Fig. S1 (ESI†), and the calculated spatial distributions of the LUMO for these polymers are shown in Fig. S2 (ESI†). Detailed information for the theoretical simulation is available in Note S1 (ESI†). The results in Fig. 2a indicate that the higher the CE performance of a polymer, the lower the energy level of its lowest unoccupied molecular orbital (LUMO). For example, PE has the highest LUMO, and it is scarcely electrified when in contact with water. In contrast, PTFE, which exhibits the lowest LUMO, is the best performer in terms of obtaining electrons from water molecules. The calculated LUMOs of these polymers under a solvent environment also demonstrates the same trend22 (ESI,† Fig. S3). The consistency between the energy level of the LUMO and CE ability indicates that the LUMO of the polymer might be responsible for accepting the transferred electron during CE. To verify this assumption, we further compared the vertical attachment energy (VAE) of different polymeric chains, as demonstrated in Fig. 2b. The minimum VAE was observed for PTFE, followed by PVDF, PVC, PP and PE, suggesting that less energy is required when transferring an electron to a polymer with a lower LUMO. Moreover, this tendency is in agreement with a previous study on the relationship between CE capacity and the functional groups of polymers.15 This consistency not only indicates the dominant role of LUMO in CE-driven electron transfer between polymers and water molecules but also implies that the impact on the LUMO level might be the underlying mechanism for illustrating the contribution of functional groups on CE performance. The impact of surface defects on the electron transfer process was also investigated by taking PTFE with a C dangling bond as an example. The obtained results shown in Fig. S4 (ESI†) suggest that the LUMO is reduced after the C dangling bond, an electron-deficient defect, is introduced. A possible energy diagram illustrating the electron transfer process during CE between polymers and water molecules is proposed in Fig. 2c. Prior to contact, the LUMO of the polymer is empty, but the energy barrier for transferring electrons from the water molecules to the LUMO of the polymer is too high. During contact, the energy barrier should decrease as a result of the overlap between the corresponding electron clouds,23 and electron transfer is feasible as long as the input mechanical energy is sufficient. The transferred electrons would stay in the LUMO level of the polymers after separating from the water molecules. Thus, electrons would gradually accumulate on the polymer after repeated contact with water. Certainly, in the case in which the HOMO of the polymers is not fully occupied, i.e., when there is still electron vacancy available and the energy for overcoming the Coulomb repulsive force is not too high, the HOMO could also partly participate in the electron transfer process during CE, as presented in Fig. S5 (ESI†).
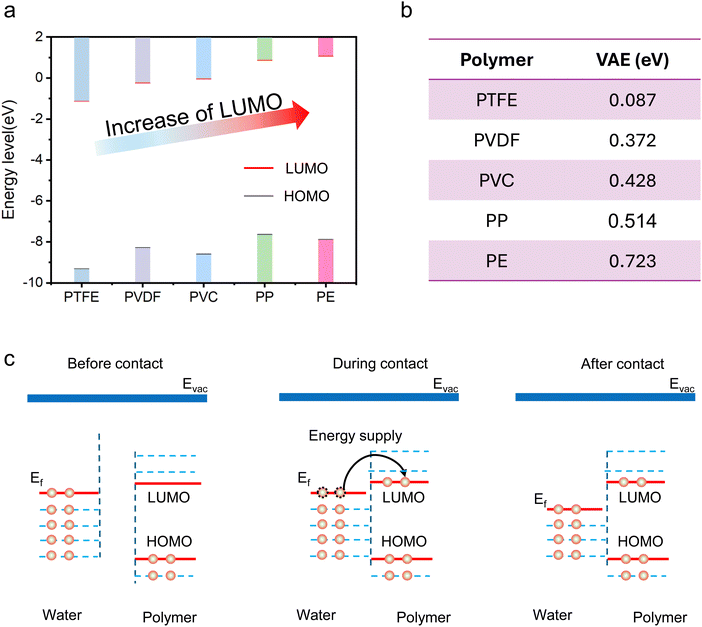 |
| Fig. 2 Theoretical investigations illustrating the varying CE ability of different polymers. (a) Calculated energy levels of the frontier molecular orbitals of various polymers. (b) Vertical attachment energy (VAE) of different polymers. (c) Proposed mechanism for the electron transfer process between polymers and water molecules. | |
Understanding CEC from the frontier molecular orbitals
Contact-electro-catalysis (CEC) refers to the promotion of chemical reactions via CE-driven electron transfer. Fig. 3a summarizes the process of utilizing the CE-driven electron transfer on a polymer surface to promote the formation of hydroxyl and superoxide radicals. Electrons will be transferred from water molecules to the polymer surface when sufficient energy is supplied during their contact. Water molecules are converted to hydroxyl radicals after losing electrons,7 and the LUMO of the polymer should receive these exchanged electrons. According to the proposed energy diagram, more electrons will be transferred to polymers with a lower LUMO for a given energy input. This assumption was verified by the measured results in Fig. 3b using terephthalic acid (THA) as the capture agent to characterize the production of hydroxyl radicals.24 The highest yield of hydroxyl radicals is achieved for PTFE, and no obvious production of hydroxy radical is observed for PE and PP, whose LUMOs are around 2 eV higher than that of PTFE. Subsequently, the accumulated electrons on the polymers will be transferred to oxygen molecules upon contact. The polymers will revert to their original uncharged state, and the superoxide radical will be formed after the oxygen molecules obtain these electrons. The production of superoxide radicals was estimated using nitro blue tetrazolium (NBT) as the capture agent in Fig. 3c.25 PTFE is also the best performer in producing superoxide radicals, which should be ascribed to that fact that more electrons are accumulated on its surface during the previous CE with DI water. Superoxide radicals are scarcely produced by PE or PP, since nearly no electrons are available on their surfaces for subsequent reactions. The reactivity of the produced radicals was examined through the degradation of a methyl orange (MO) aqueous solution, as shown in Fig. 3d, and the dominant role of these two radicals in degrading MO was confirmed by the capture experiment in Fig. 3e. In addition, the enhancement of reactive oxygen species (ROS) production under increased ultrasonic power is illustrated in this FMO-based energy diagram. Thus, a more complete description of the CEC process is available after taking the FMO into consideration. We expect that a contact-electro-catalytic diagram could be established based on this study, which could facilitate the selection of suitable catalysts and mechanical agitation for catalyzing target reactions.
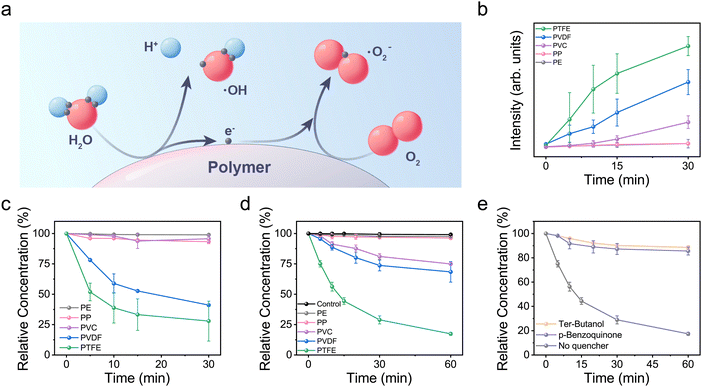 |
| Fig. 3 Variation of contact-electro-catalysis (CEC) when different polymers are utilized. (a) Schematic illustration of the CEC process for producing reactive oxygen species (ROS). (b) Evaluation of the production of OH radicals in different groups using the fluorescence intensity of THA-OH. (c) Evaluation of the production of superoxide radical in different groups using the degradation of NBT aqueous solutions. (d) Degradation of methyl orange (MO) aqueous solution by CEC-induced ROS. (e) Evolution of the relative concentration of MO in the presence of different radical scavengers with the same final concentration of 1 mM. | |
Conclusions
Using contact-electrification (CE) with deionized (DI) water as a representative example, the contribution of the frontier molecular orbitals (FMO) of polymers to the electron transfer process during CE was investigated. The obtained results suggest that the lowest unoccupied molecular orbital (LUMO) may play a dominant role in accepting transferred electrons from water molecules. The calculated vertical attachment energies (VAE) of the different polymers further prove that less energy is required for electron exchange between the DI water and polymers with a lower LUMO. A corresponding schematic illustration was thus proposed, in which electrons are mainly transferred from water molecules to the empty LUMO when sufficient mechanical energy is applied. The trend in the contact-electro-catalysis (CEC) could also be well explained by this mechanism. Sufficient energy is necessary for the transfer of the electrons to the polymers during CE, and more electrons will be exchanged if a higher energy is supplied. For a given energy supply, more hydroxyl radicals could be produced by polymers with a lower LUMO, since more electrons would be transferred from water molecules. Additionally, more electrons would accumulate on the surface of such polymers, facilitating the subsequent formation of superoxide radicals. It is noteworthy that, in realistic situations, surface defects may also contribute to the electron transfer process since they can accommodate electrons. Therefore, some surface states may also be electron acceptors, similar to the case of semiconductors. In summary, we expect that this study will not only provide insights for promoting CEC from in the area of materials but also lay the foundation for establishing a quantified contact-electro-catalytic diagram.
Author contributions
W. T. conceived the idea and supervised the experiment. Z. W., X. D., F.-J. L., and W. T. prepared the manuscript. Z. W. and X. D. developed the experimental setups. F.-J. L. and X. D. performed data measurements. All the authors discussed the results and commented on the manuscript. This work was financially supported by the Beijing Municipal Science and Technology Commission (Grant No. Z181100003818016, Z171100000317001, Z171100002017017, and Y3993113DF, W. T.); Youth Innovation Promotion Association, Chinese Academy of Sciences (W. T.); and China Postdoctoral Science Foundation (Grant No. 2022M723101, Z. W.).
Data availability
The data supporting this article have been included as part of the ESI.†
Conflicts of interest
There are no conflicts to declare.
References
- S. Lin, X. Chen and Z. L. Wang, Chem. Rev., 2022, 122, 5209–5232 CrossRef CAS.
- F. Zhan, A. C. Wang, L. Xu, S. Lin, J. Shao, X. Chen and Z. L. Wang, ACS Nano, 2020, 14, 17565–17573 CrossRef CAS PubMed.
- J. Nie, Z. Wang, Z. Ren, S. Li, X. Chen and Z. Lin Wang, Nat. Commun., 2019, 10, 2264 CrossRef.
- J. Nie, Z. Ren, L. Xu, S. Lin, F. Zhan, X. Chen and Z. L. Wang, Adv. Mater., 2020, 32, 1905696 CrossRef CAS PubMed.
- S. Lin, L. Xu, A. Chi Wang and Z. L. Wang, Nat. Commun., 2020, 11, 399 CrossRef CAS PubMed.
- S. Lin, L. Zhu, Z. Tang and Z. L. Wang, Nat. Commun., 2022, 13, 5230 CrossRef CAS.
- Z. Wang, A. Berbille, Y. Feng, S. Li, L. Zhu, W. Tang and Z. L. Wang, Nat. Commun., 2022, 13, 130 CrossRef CAS PubMed.
- Z. Wang, X. Dong, W. Tang and Z. L. Wang, Chem. Soc. Rev., 2024, 53, 4349–4373 RSC.
- H. Li, A. Berbille, X. Zhao, Z. Wang, W. Tang and Z. L. Wang, Nat. Energy, 2023, 8, 1137–1144 CrossRef CAS.
- W. Li, J. Sun, M. Wang, J. Xu, Y. Wang, L. Yang, R. Yan, H. He, S. Wang, W.-Q. Deng, Z.-Q. Tian and F. R. Fan, Angew. Chem., Int. Ed., 2024, 63, e202403114 CrossRef CAS PubMed.
- J. Zhao, X. Zhang, J. Xu, W. Tang, Z. Lin Wang and F. Ru Fan, Angew. Chem., 2023, 135, e202300604 CrossRef.
- Y. Zhao, Y. Liu, Y. Wang, S. Li, Y. Liu, Z. L. Wang and P. Jiang, Nano Energy, 2023, 112, 108464 CrossRef CAS.
- Z. Wang, X. Dong, X.-F. Li, Y. Feng, S. Li, W. Tang and Z. L. Wang, Nat. Commun., 2024, 15, 757 CrossRef CAS PubMed.
- S. Li, Z. Zhang, P. Peng, X. Li, Z. L. Wang and D. Wei, Nano Energy, 2024, 122, 109286 CrossRef CAS.
- S. Li, J. Nie, Y. Shi, X. Tao, F. Wang, J. Tian, S. Lin, X. Chen and Z. L. Wang, Adv. Mater., 2020, 32, 2001307 CrossRef CAS.
- X. Dong, Z. Wang, A. Berbille, X. Zhao, W. Tang and Z. L. Wang, Nano Energy, 2022, 99, 107346 CrossRef CAS.
- B. Chen, Y. Xia, R. He, H. Sang, W. Zhang, J. Li, L. Chen, P. Wang, S. Guo and Y. Yin, Proc. Natl. Acad. Sci. U. S. A., 2022, 119, e2209056119 CrossRef CAS.
- J. Zhang, S. Lin and Z. L. Wang, ACS Nano, 2023, 17, 1646–1652 CrossRef CAS PubMed.
- J. Li, Y. Xia, X. Song, B. Chen and R. N. Zare, Proc. Natl. Acad. Sci. U. S. A., 2024, 121, e2318408121 CrossRef CAS PubMed.
- Y. Xu, C. Li, Z. Li, Q. Wang, X. Cai, J. Wei and Y. Wang, Angew. Chem., Int. Ed., 2020, 59, 17442–17446 CrossRef CAS PubMed.
- C. Xu, B. Zhang, A. C. Wang, H. Zou, G. Liu, W. Ding, C. Wu, M. Ma, P. Feng, Z. Lin and Z. L. Wang, ACS Nano, 2019, 13, 2034–2041 CAS.
- W. Li, J. Sun, M. Wang, J. Xu, Y. Wang, L. Yang, R. Yan, H. He, S. Wang and W. Q. Deng, Angew. Chem., 2024, e202403114 CAS.
- Z. L. Wang and A. C. Wang, Mater. Today, 2019, 30, 34–51 CrossRef CAS.
- J. C. Barreto, G. S. Smith, N. H. Strobel, P. A. McQuillin and T. A. Miller, Life Sci., 1994, 56, PL89–PL96 CrossRef PubMed.
- H. Huang, S. Tu, C. Zeng, T. Zhang, A. H. Reshak and Y. Zhang, Angew. Chem., Int. Ed., 2017, 56, 11860–11864 CrossRef CAS.
|
This journal is © The Royal Society of Chemistry 2024 |
Click here to see how this site uses Cookies. View our privacy policy here.