DOI:
10.1039/D3MD00596H
(Review Article)
RSC Med. Chem., 2024,
15, 433-471
Overcoming barriers with non-covalent interactions: supramolecular recognition of adamantyl cucurbit[n]uril assemblies for medical applications
Received
24th October 2023
, Accepted 30th November 2023
First published on 6th December 2023
Abstract
Adamantane, a staple in medicinal chemistry, recently became a cornerstone of a supramolecular host–guest drug delivery system, ADA/CB[n]. Owing to a good fit between the adamantane cage and the host cavity of the cucurbit[n]uril macrocycle, formed strong inclusion complexes find applications in drug delivery and controlled drug release. Note that the cucurbit[n]uril host is not solely a delivery vehicle of the ADA/CB[n] system but rather influences the bioactivity and bioavailability of drug molecules and can tune drug properties. Namely, as host–guest interactions are capable of changing the intrinsic properties of the guest molecule, inclusion complexes can become more soluble, bioavailable and more resistant to metabolic conditions compared to individual non-complexed molecules. Such synergistic effects have implications for practical bioapplicability of this complex system and provide a new viewpoint to therapy, beyond the traditional single drug molecule approach. By achieving a balance between guest encapsulation and release, the ADA/CB[n] system has also found use beyond just drug delivery, in fields like bioanalytics, sensing assays, bioimaging, etc. Thus, chemosensing in physiological conditions, indicator displacement assays, in vivo diagnostics and hybrid nanostructures are just some recent examples of the ADA/CB[n] applicability, be it for displacements purposes or as cargo vehicles.
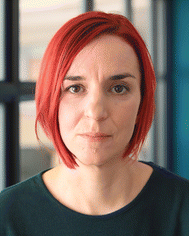 Marija Alešković | Marija Alešković is a senior research associate at the Ruđer Bošković Institute, Zagreb, Croatia. She was born in 1980 in Zagreb, and received her Ph.D. (under the mentorship of Nikola Basarić, Ph.D. and Prof. Kata Majerski) in 2011 from the Faculty of Science, University of Zagreb. Her research interests include organic synthesis, diamondoids, supramolecular chemistry of anion receptors and host–guest chemistry of polycyclic compounds. |
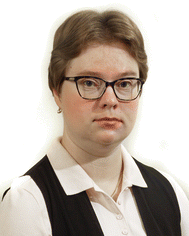 Marina Šekutor | Marina Šekutor is a senior research associate at the Ruđer Bošković Institute, Zagreb, Croatia. She was born in 1986 in Zagreb, received her Ph.D. (under the mentorship of Prof. Kata Majerski) in 2013 from the Faculty of Science, University of Zagreb and did her postdoctoral research as a Humboldt fellow in the group of Prof. Peter R. Schreiner (2015–2017, Germany). For her work she has received several recognitions, including the Award for organic chemistry “Vladimir Prelog” in 2019 (Croatian Chemical Society). Her research interests include supramolecular host–guest chemistry of polycyclic compounds, cluster assemblies in helium nanodroplets, and use of diamondoid derivatives in materials science. |
1. Introduction to the adamantane scaffold
Adamantane is a fascinating molecule. It has attracted the interest of chemists since it was first discovered and isolated from crude oil in 1933.1 After its first synthesis by Vladimir Prelog and Rativoj Seiwerth in 1941 (ref. 2 and 3) and later synthetic scale-up utilizing the Lewis-acid catalyzed rearrangement protocol perfected by Paul von Ragué Schleyer,4,5 the chemistry of adamantane6,7 virtually exploded and the molecule became available on an industrial scale. Potential of the adamantane cage as a scaffold in medicinal chemistry was realized soon after and in parallel to synthetic endeavors research on adamantane derivatives also took a pharmacological direction.8–11 Consequently, the question emerges why adamantane is such a good candidate for bioactive compounds. The answer lies in its structure and the consequences it has on the compound's physicochemical nature. Adamantane (C10H16) is the smallest homologue of diamondoids,12,13 naturally occurring14 cage hydrocarbons that possess the structural arrangement of their carbon atoms comparable to a diamond crystal lattice. Adamantane is thus characterized by its high (Td) symmetry and many exciting properties, like low molecular strain, high thermodynamic stability, high lipophilicity, etc.,7 combined with vast possibilities of cage functionalization with both donor and acceptor functional groups.6,7,15
Application of the adamantane scaffold in medicinal chemistry has a long history dating back to the 1960s when 1-aminoadamantane was discovered to be active against influenza A,16 however, to detail every aspect of its utility is outside the scope of this review. A general feature important to note is that the adamantane cage structure is commonly used as a bulky lipophilic addition to a complex structure of an already bioactive molecule since it enables easier crossings of drugs across cell membranes and can also enhance their positive metabolic profiles.9,11 However, adamantane's use as a valuable subunit for enhancing properties of known pharmacophores is not its only strength; some simple adamantane derivatives can also have medicinal applications. For example, amantadine (1-aminoadamantane), rimantadine (1-(1-aminoethyl)adamantane) and memantine (1-amino-3,5-dimethyladamantane), simple amine derivatives, found use against viral infections (influenza A, herpes simplex) and neurodegenerative disorders (Parkinson's and Alzheimer's disease).11 The antiviral mode of action of simple adamantane amines consists of ion channel blocking of the viral transmembrane proteins,17 effectively preventing further virus replication,18 while the application as neuroactive compounds is based on a high lipophilicity of adamantane that enables efficient crossing of the molecules across the blood–brain barrier.19 Over the years in our laboratory we also explored many adamantane-based compounds as potential bioactive molecules, ranging from applications in enzyme inhibition20,21 and antitrypanosomal activity22 to their liposome incorporation as a means for targeted compound delivery.23–25
In recent times adamantane derivatives have started to attract focus as components of complex drug delivery systems and this exciting new research direction is the main topic of this review. However, before we turn our attention to practical applications, we first need to mention a few key features of the adamantane scaffold that are important in the context of host–guest chemistry. Namely, the adamantane cage is long known to be an efficient scaffold in supramolecular recognition processes, both in chemical and biological systems.26 Going from our own experience, adamantane and similar cage compounds have demonstrated an amazing versatility and can serve as a backbone in various cation27–34 and anion receptors35–44 as well as being desirable guest molecules in inclusion complexes.45–51 While in macrocyclic receptors the adamantane cage usually serves as a rigid backbone that reduces the conformational mobility of a macrocycle and thus pre-organizes the receptor molecule for binding, in the inclusion complexes the role of adamantane is primarily limited to enabling preferential binding of the polycyclic subunit into a host molecules. Exactly this host–guest behavior of adamantane derivatives will be the focus of our continued discussion, as it provides a useful tool for recognition in biological systems.
It is known that adamantane derivatives excellently bind to a β-cyclodextrin host and afford stable 1
:
1 inclusion complexes, with association constants typically between 103–105 M−1.24,50,52–57 Cyclodextrins (CDs) are macrocycles consisting of glucose monomers and are widely used in drug delivery since they are water-soluble, naturally occurring, cheap, and able to bind a wide range of substrates.58 Important to note is that cyclodextrins have a well-defined central hydrophobic cavity that is crucial for successful adamantane incorporation and formation of a strong inclusion complex. A good fit between the β-cyclodextrin cavity and the lipophilic adamantane cage thus enables optimal engagement in non-covalent interactions between the two molecules. This behavior has important implications for potential medicinal use since complexation can facilitate better drug molecule solubility and focused delivery to a biological target.59 However, widespread use of cyclodextrin for this purpose is still somewhat hampered because of difficulties in membrane crossings of the resulting complexes, often too low binding constants for quantitative drug delivery and too pronounced hydrophilic nature of the host mantle itself. On the other hand, in recent years a different host molecule class, cucurbit[n]urils, appeared in the scientific spotlight, also capable of efficient non-covalent binding with adamantane derivatives.
2. Properties and molecular binding of cucurbit[n]urils
In the beginning of the 20th century Behrend et al. synthesized a very stable compound from glycoluril and formaldehyde in acidic reaction conditions.60 Based only on elemental analysis the authors suggested that this amorphous insoluble polymeric material consisted of three glycoluril molecules condensed together with twice as much equivalents of formaldehyde. However, the correct structure of the obtained molecule remained unknown up until 1981 when Freeman and Mock,61 intrigued by this exceptionally thermodynamically stable compound, revised its synthesis. Using modern spectroscopic techniques like NMR and X-ray analysis they elucidated that the stable material in question actually consisted of six glycoluril building blocks interlinked by twelve methylene bridges. The authors also proposed the trivial name “cucurbituril” for the compound because of its structural resemblance to a pumpkin (Cucurbitaceae family), as well as to a cucurbit, a gourd-shaped flask that was a common component of the alchemic distillation equipment.61 Using modern terminology, we nowadays call this compound cucurbit[6]uril or CB[6] or sometimes designated as CB6, Q[6], Q6 or Cuc6, where the number in the brackets represents the number of glycoluril subunits of the macrocycle (i.e., here a hexamer). Cucurbit[6]uril is thus the first described representative of the cucurbituril family of compounds. In early 2000s Kim reported the synthesis of three other macrocycles from the class that differed in their size, namely CB[5], CB[7] and CB[8].62 The trick to obtain other CB[n]s was in lowering and controlling the temperature of the reaction which afforded these kinetic products (Fig. 1).
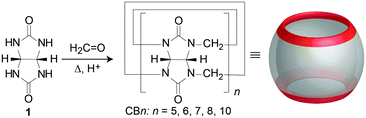 |
| Fig. 1 Synthesis of CB[n]s from glycoluril and formaldehyde under acidic conditions. Reproduced with permission from ref. 63, the Royal Society of Chemistry, 2015. | |
It is curious to note that the discovery of both cyclodextrin and cucurbituril macrocycle families have a similar timeline. However, the studies on CDs have remained consistent over the century whereas the field of cucurbituril chemistry blossomed almost a hundred years after their first synthesis, following the discovery of the mentioned new homologues. Possible reasons for such a slow progress of CB[n] chemistry could be ambiguous and somewhat inconsistent synthetic and purification procedures that only recently became systematic,62,64,65 and that enabled their commercial availability. In addition to the parent CB[n] compounds, other derivatives have been developed as well,66,67 like long chain substituted compounds,68 twisted69,70 and inverted scaffolds,71 open-cavity variations,72etc.
A common feature of all CB[n] and similar host scaffolds mentioned earlier is the existence of a deep, centrally positioned cavity. This structural attribute is essential for the accommodation of guest molecules and the formation of inclusion complexes. Ability of CB[n]s to complex small organic molecules was noted soon after their rediscovery in 1980s73 and from that point onwards the supramolecular chemistry of CB[n]s was developing at an astounding rate and scale.63,74–80 Despite the most common stoichiometry of the complexes between CB[n]s and the guest molecules being 1
:
1, CB[10]81,82 can accommodate even two guest molecules within its cavity. Curiously, CB[10] itself was first isolated as a CB[5]@CB[10] complex.81 The complex strength, i.e., effectiveness of the binding, is expressed through values of the stability constants which are basically the ratio of the rate constant for the complex formation (ingression) and dissociation (egression). It should also be mentioned that CB[n] homologues CB[5]–CB[10]61,62,75,81 have all been fully characterized by X-ray crystallography (Fig. 2), providing key structure parameters that are summarized in Table 1.
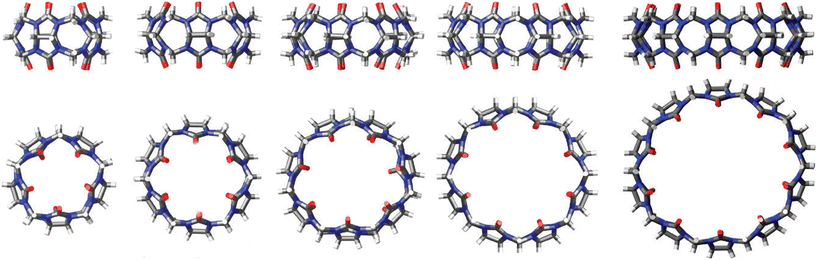 |
| Fig. 2 Representations of X-ray crystal structures for CB[n] (n = 5, 6, 7, 8, 10, left to right). Reproduced with permission from ref. 75, the Royal Society of Chemistry, 2009. | |
Table 1 Structural parameters (CB[5],62 CB[6],61 CB[7],62 CB[8],62 CB[10]75,81) and physicochemical properties for cucurbit[n]urils
CB[n] |
CB[5] |
CB[6] |
CB[7] |
CB[8] |
CB[10] |
Outer diameter/Å |
13.1 |
14.4 |
16.0 |
17.5 |
20.0 |
Inner cavity diameter/Å |
4.4 |
5.8 |
7.3 |
8.8 |
11.7 |
Portal diameter/Å |
2.4 |
3.9 |
5.4 |
6.9 |
10.0 |
Inner cavity volume/Å3 |
84 |
162 |
279 |
479 |
691 |
Height |
9.1 |
9.1 |
9.1 |
9.1 |
9.1 |
Number of water molecules in cavity84 |
2 |
4 |
8 |
12 |
22 |
Solubility in water74,75/mM |
20–30 |
0.02 |
20–30 |
<0.01 |
<0.05 (ref. 82) |
Stability74/°C |
>420 |
425 (ref. 85) |
370 |
>420 |
|
All the mentioned unmodified CB[n]s have the height of 9.1 Å, which is similar to the depth of the CDs, whereas by adding more glycoluril units to the macrocycle ring the cavity diameter and its volume increase (Fig. 2). As can be seen from Fig. 2, on the top and the bottom of the cavity two hydrophilic carbonylated rims are symmetrically positioned. The portals are approximately 2 Å narrower than the cavity, enabling discrimination of the guest molecules based on their size and thus accomplishing selectivity. Additionally, such doubly pinched geometry provides a barrier to association and dissociation of guest molecules. Because of the electronegative nature of the portals, cationic guests form with CB[n] hosts inclusion complexes that are in principle of higher affinity than is the case for comparably sized neutral guest molecules.
As it was noted right from their first synthesis,60 CB[n]s are unusually thermally stable molecules, while their main drawback is their somewhat poor water solubility. Curiously, CB[n] macrocycles with an odd number of glycoluril subunits (n = 5, 7) are more soluble in pure water than CB[n]s with an even number (n = 6, 8, 10). This can be explained by stabilization of the crystal lattice with multiple close and strong contacts between macrocycles which outnumber the close contacts of CB[n] with water. Water solubility can be enhanced by adding acids or ionic salts to the medium or upon encapsulation with amphiphilic guests. Quite recently it was found that their limited solubility can also be overcome by mechanochemical synthesis of host–guest complexes with CB[7].83
Every region of these exceptional macromolecules has its function in molecular recognition processes and enables a good overall fit and ultimately the formation of a thermodynamically stable complex (Fig. 3). Beside intrinsic characteristics of the host and guest molecules, the supramolecular system also depends on the surroundings, making the binding event and complex stability sensitive to a change of solvent, temperature, ion strength, pH, etc.86–88 Moreover, these supramolecular interactions and macrocyclic characteristics can lead to additional phenomena such as change in intrinsic properties of the guest molecule (e.g., higher solubility), improved chemical and thermal stability, etc. For example, it was found that when a protonated basic guest forms inclusion complexes within the CB[n] cavity the pKa value of the guest becomes greater, demonstrating a different preference for binding of a protonated form when compared to the neutral one.89 Complexation induced shifting of the pKa values of the guest molecules has already found applications in sensors,90–94 assays,95,96 as well as in drug delivery systems.97–99
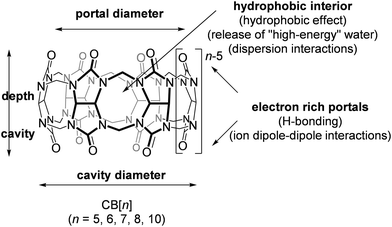 |
| Fig. 3 CB[n]s regions important for molecular recognition. | |
On another note, it was postulated that the optimum occupancy of the CB[n] host interior for the inclusion of guest molecules would be 55 ± 9%.100 In that case physicochemical properties of the guest molecule such as size and its shape to appropriately fit into the host interior could be predictors of selectivity and binding intensity.84 However, there have been examples where packing coefficient is greater or smaller than supposedly optimal and in those cases additional interactions emerge to compensate for the postulated non-ideal packing inside the cavity.45,101 Here should also be noted that the cavity of the “empty” host is not actually empty in any real world system, since it readily interacts with solvent molecules. In fact, CB[n]'s interior can host between 2 and 22 water molecules, depending on its size. In the case of aqueous solutions, water molecules occupying the cavity of low polarity are somewhat isolated from bulk water molecules and as a consequence cannot be optimally stabilized through hydrogen bonds as would be the case in the bulk water environment.102 The release of these so-called “high-energy” water molecules upon encapsulation of the guest molecule is actually one of the primary driving forces for complex formation and was proven to be responsible for strong affinities (Fig. 4).84,103,104
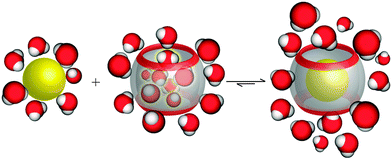 |
| Fig. 4 Depiction of the release of high-energy water molecules from the CB[n] cavity upon binding of a hydrophobic guest. Reproduced with permission from ref. 63, the Royal Society of Chemistry, 2015. | |
Interactions such as the hydrophobic effect and dipole–dipole or ion–dipole interactions between the guest molecules and the electronegative CB[n] portal rim are other important stabilizing factors during the binding event. Dispersion interactions are also to be considered for binding because they can provide an additional boost in the overall association constant values despite their weaker individual strength when compared to other interaction types. All these interactions and effects are synergistic and can result in exceptionally stable complexes with high association constants that were previously rarely or never observed. Such extremely stable complexes can even rival the avidin–biotin affinity,105,106 which is known to be one of the strongest non-covalent interactions between two species found in nature.45,107–111 In continuation we will see how these exceptional characteristics of CB[n]s can be utilized for pharmacologically applicable supramolecular host–guest assemblies.
3. Cucurbit[n]urils in drug delivery
Supramolecular chemistry provides a versatile platform for creating complex structures with applications ranging from drug delivery, therapeutic interventions to diagnostics. Molecular recognition and encapsulation of hydrophobic species in a macrocycle's cavity (especially in aqueous environment) is perhaps the most important advantage of cyclic macromolecules like cyclodextrins,112 pillar[n]arenes,113 cucurbit[n]urils, calixarenes,114etc. Such cyclic host molecules are therefore an excellent alternative to other popular drug delivery systems like micelles,115,116 dendrimers,117 liposomes,118etc., mainly because of the former's thermal and chemical stability, availability of different cavity sizes and the ability to self-assemble into various nanostructures. Macrocyclic carriers in general have well defined binding ability both in vitro and in vivo and the bioactive guest molecule encapsulated in the cavity is protected against inactivation or metabolic degradation, leading eventually to a controlled drug release. In other words, the macrocyclic cavity can be viewed as the binding region of a biological receptor which has a pre-organized shape for the formation of a host–guest complex. This biomimetic function, along with their biocompatibility, intrigued medicinal chemists for a long time and Freudenberg et al. first patented the idea of using cyclodextrins for the inclusion of physiologically active organic compounds as early as the 1950s.119 However, it took almost 25 years after that initial idea until the first cyclodextrin-containing pharmaceutical product was actually marketed.120 Nevertheless, from that point onwards cyclodextrin drug carriers have been constantly developing and currently there are about 35 different approved CD-containing drug products available,120–122 with the overall number of drug containing macrocycles continuously growing.123,124 In fact, usage of a known pharmaceutically active compound which upon binding125 demonstrates enhancement of its initial properties in terms of stability, solubility, etc. is often less time consuming and has economic advantages over the search and testing of new potential candidates. Modulation of chemical and physical parameters of bioactive species in inclusion complexes will be discussed later in more detail.98,126
As for the cucurbit[n]urils, after optimization of their synthesis,62 isolation62,64 and purity analytics,127 studies on this macrocycle family have initially been focused mostly on their molecular recognition properties.73 However, that shifted in recent times towards potential application, especially as even more economical synthesis procedures have been developed, e.g., by using microwave techniques128,129 or through solvent free mechanochemistry methods.130 Nowadays CB[n]s have taken over the prominent spot of highly promising macrocyclic candidates for biological applications and drug delivery.98,122,125,131–151 Moreover, CB[n] hosts have shown potential as supramolecular antidotes, neutralizing the effects of a poison though the encapsulation of a small toxic molecule.147,152,153 The so-called chemical reasons for these new trends are the observations that CDs, when compared to CB[n]s, show generally lower selectivity and weaker binding affinities52 (Ka are usually lower than 105 M−1). It follows that in order to achieve quantitative binding of the guests, one needs to use much larger concentrations of CDs, while CB[n]s possess inherently stronger potential for efficient binding. In other words, because of the high stability constants only small amounts of free uncomplexed CB[n] molecules will be present in solutions, with >99% of drug complexation being achieved. Note however that not all homologues of the CB[n] series are equally suitable as drug carriers; CB[5] is too small to encapsulate drug molecules, while CB[10] is often too large to produce high affinity complexes. CB[6], CB[7] and CB[8] are therefore at present the most commonly considered analogues for bioapplications and in drug delivery systems.
As already mentioned in the introduction, many adamantane (ADA) derivatives have been used as drugs10 for decades and the realization that the adamantyl cage almost ideally occupies the corresponding cucurbituril cavity instantly led to studies on how this host–guest supramolecular system could affect bioactivity and bioavailability of the drug molecule as well as tune drug properties. Moreover, it was also explored how the ADA/CB[n] supramolecular interaction could be implemented in fields other than just drug carrier design, like in sensing, general biology and medicine in a broader sense. We will therefore present in this targeted review illustrative examples of ADA/CB[n] use in biological applications, with special focus not only on the straightforward drug-in-a-carrier cases but also on broader examples of bioactive supramolecular systems benefiting from this extraordinary supramolecular interaction.
Using hydrophobic ADA in combination with CB[n]s is a sort of a golden standard in this field because the scaffold in question fits so snugly into the central cavity of the host,108,109 thus accomplishing strong and selective binding. The consequence is that the ADA/CB[n] system is much more efficient at achieving total encapsulation than is the case for many other guest molecules.154 The binding preferences of various ADA derivatives for CB[n]s have been explored thermodynamically, structurally and electronically,109 thereby making most of the aspects of its molecular recognition in supramolecular processes known. Table 2 presents proof-of-concept association values for complex formation with two commonly used ADA derivatives in a 50 mM NaO2CCD3-buffered D2O (pD 4.74), as determined by 1H NMR competition experiments.
Table 2 Values of Ka (M−1) for the interaction of amantadine and memantine with CB[7] and CB[8]109
Compound |
CB[7] |
CB[8] |
Amantadine |
(4.23 ± 1.00) × 1012 |
(8.19 ± 1.75) × 108 |
Memantine |
(2.50 ± 0.39) × 104 |
(4.33 ± 1.11) × 1011 |
When comparing amantadine (1-aminoadamantane) affinities towards CB[7] and CB[8], it can be seen that the binding preference is significantly in favor of CB[7] (Table 2). This is presumably because of the optimal structural packaging and better complementarity of the ADA scaffold and the CB[7] cavity, resulting in much higher Ka value. At the same time, the opposite is observed for the binding of a trisubstituted ADA derivative memantine (1-amino-3,5-dimethyl-adamantane), i.e., memantine/CB[8] is 107-fold tighter than the complex of memantine/CB[7]. The most probable explanation is that two additional methyl substituents on the ADA cage destabilize the complex that forms with a smaller CB[7] due to unfavorable steric interactions between the convex surface of the guest and the concave cavity wall surface of the host. Nevertheless, CB[n]-type macrocycles retain a relatively high binding affinity towards both amantadine155 and memantine.156
The charge present on protonated adamantane derivatives introduces electrostatic interactions with the CB[n]'s rim, enhancing the binding affinity to CB[n] compared to neutral adamantane compounds, which inspired Isaacs and coworkers to test how the observed trend would affect mechanical features of the two complex types.48 They constructed an assembled DNA template in which a flexible DNA linker was exploited to keep the CB[7] and ADA in close proximity. It was found that positively charged ADA guest possesses higher mechanical stability (49 pN) than neutral adamantane (44 pN) holding on to CB[7], which falls in the strength range of many biological recognitions. Furthermore, it was found that a hexyl group adjacent to the ADA moiety served as a chaperone to assist the formation of the ADA–CB[7] pairs, which was not observed with ADA/CD complexes.157
Extraordinarily stable complexes formed between the adamantyl group and the CB[n]-type macrocycles enable implementation of this supramolecular system in tracking pathways and intracellular delivery since almost quantitative complexation occurs at sub-nanomolar concentrations. An excellent example that illustrates this point very well was reported very recently, dealing with evaluation of the uptake potential of cyclic peptide (VH4127) connected to CB[7] that non-competitively targets the low density lipoprotein (LDL) receptor (LDLR) in order to achieve a new kind of drug delivery system (DDS) (Fig. 5).158 In this context an analogous conjugate was synthesized which comprised of ADA coupled with a fluorescent group and the resulting supramolecular complex retained binding to LDLR and even improved LDLR-mediated endocytosis and intracellular accumulation potential in LDLR-expressing cells. The authors concluded that such an approach expands the design possibilities of a wide range of therapeutic or imaging vehicles through utilization of the transport abilities of the CB[n] macrocycle.
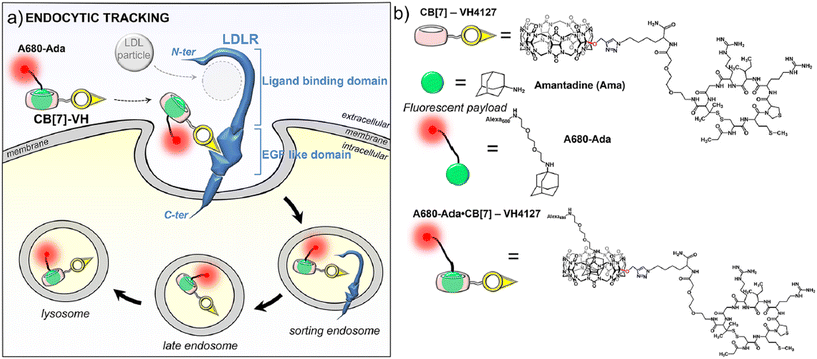 |
| Fig. 5 Illustration of non-competitive binding of a supramolecular complex consisting of an ADA-derivative and peptide-functionalized CB[7] to a low density lipoprotein receptor (LDLR). Reproduced with permission from ref. 158, the American Chemical Society, 2023. | |
However, one must carefully design the system in which ADA/CB[n] will be incorporated and estimate whether the final assembly would be too bulky and/or hydrophilic to enter the cells in sufficient amount as it was demonstrated in the case of four CB[7]s that were non-covalently attached to aminoadamantyl-substituted phthalocyanines (Pcs).159 The study in question showed that supramolecular interaction between CB[7] with Pcs in water improved both fluorescence and singlet oxygen production. On the other hand, bioactivity decreased for at least an order of magnitude after host–guest interaction with CB[7], which was attributed to much lower uptake by cells due to the aforementioned reasons.
3.1. Bioactivity, toxicity and membrane crossing
From a bioapplicability perspective, CDs can be nephrotoxic160 if they enter the body in a non-metabolized state, thus precipitating as complexes with cholesterol.161 On the other hand, CB[n]s demonstrated lower toxicity in preliminary assays.162–166 In order to assess bioavailability and toxicity of CB[n]s, first it has to be determined whether the host–guest complexes can cross cell membranes. Afterwards, it needs to be estimated if CB[n] itself is (non)toxic and whether the CB[n] complex combined with the drug is (non)toxic as well. Finally, it needs to be determined whether the drug inclusion in CB[n] decreases the toxicity side-effects of the drug itself. Ideal function and safety requirements of the envisioned macrocyclic host imply that it would carry the drug molecule to a desired location within the body, crossing barriers and delivering the active compound at a defined concentration, preferably through a slow, sustained release. At the same time it would be desirable that it does not decrease the activity of the drug towards targets in the organism. Finally, the carrier itself has to be efficiently excreted from the body, either unchanged or in a metabolized form. With those desirable traits in mind, hemocompatibility of erythrocytes and leukocytes with CB[n]s was investigated and no cytotoxic effect was observed even at high concentrations (1 mM).167 Even though CB[n]s do not damage cells directly, enhancement of the early apoptosis of lymphocytes was noticed, thus, CB[7] enhances hemolysis in biological media. Despite this finding, the authors concluded that CB[n]s are still fairly safe organic molecules to be used in lower concentrations. Immunotoxicity and immunomodulation properties of CB[n]s were also tested and it was found that CB[7] and CB[6] did not decrease the viability of mononuclear cells at all tested concentrations (0.1–1 mM range). Furthermore, the results indicated an immunomodulatory effect of different concentrations of CB[n] and after a longer cultivation time CB[n] had an immunostimulating effect, which was indicated by an enhancement of the proliferative activity of cells and increased expression of HLA-DR on lymphocytes.168
Since CB[7] has been the most studied representative of the CB[n] family in terms of potential biological application, biocompatibility and safety of CB[7] was systemically evaluated in vitro, ex vivo, and in vivo using animal and human cell lines, zebrafish and in mice.162–166 It was shown in several in vivo studies that there is little or no obvious toxicity when the cell lines were treated with CB[7] at concentrations below 500 μM,162,163 which is far above the concentrations typically needed for drug delivery. This means that mice can survive intravenous doses up to 250 mg kg−1 of body weight,162 which indeed makes CB[7] a promising candidate in leading drug delivery exploration. The ex vivo detailed studies showed neurotoxicity, myotoxicity, and cardiotoxicity of CB[6] and CB[7] at 1 mM, 300 μM, and 300 μM, respectively.164 What is more, toxicity evaluation of a complex consisting of platinum-based anticancer drug cisplatin and CB[7] on these same tissues was also performed. It was found that the free drug cisplatin displayed toxicity on all examined tissue types, but when encapsulated in CB[7], the myo- and cardiotoxic activities were considerably reduced, while no relevant change in toxicity was observed in the neurotoxicity studies.164 Encapsulation of cisplatin in the CB[7] cavity therefore results in a decrease in its cytotoxicity to healthy cells, which could solve the issues caused by known low specificity of chemotherapeutics to cancer cells. This principle of conditioning cytotoxicity in chemotherapy by applying supramolecular systems was adopted as a new strategy aptly named supramolecular chemotherapy (Fig. 6),169–171 and is an illustrative proof-of-principle of integrating non-covalent interactions into a synergy of drug design, targeted delivery and combined drug therapy.
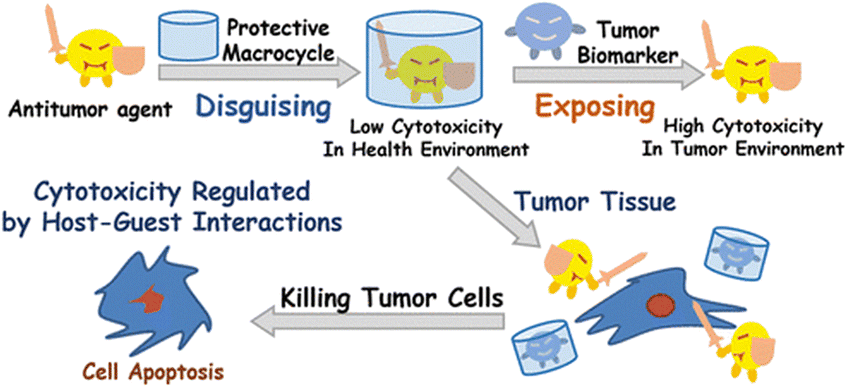 |
| Fig. 6 Schematic representation of supramolecular chemotherapy with a goal to decrease cytotoxicity and increase antitumor activity by host–guest chemistry. Reproduced with permission from ref. 169, the American Chemical Society, 2016. | |
It was previously demonstrated that a Pt(II) complex with the ADA anchored malonate in a β-CD cavity had higher toxicity toward tumor cells due to its increased DNA binding ability and more efficient cell uptake, which pointed towards advantages of applying supramolecular non-covalent interactions in anticancer therapy.172 Quite recently a group of authors173 reported a supramolecular host–guest system consisting of cationic platinum(II) compounds tethered with substituents such as amantadine, rimantadine, and bornylamine and then anchored in the cavity of the CD or CB[n] macrocyclic host (Fig. 7). Again, the idea was to improve on the platinum-based anticancer drugs using lipophilic ligands with bulky substituents that are already pharmacologically active by aiming for a more efficient penetration across membranes, reduction of side effects and tumor targeting. Because of the anchoring capability of these Pt-containing compounds and their ability to fit well into the β-CD and CB[7] cavities, the metallodrug guests are protected against rapid degradation. As expected, the authors observed a preference for the formation of more stable complexes for the CB[7] cavitand rather than for β-CD, albeit with a slower exchange between the free and the bound form. What is more, such strong host–guest interaction is responsible for a reduced release of drug molecules from the CB[n], resulting in a lower than expected biological response of the system. The authors proposed a solution to this issue by keeping the transport benefits afforded by the host molecule while modifying the release through the lowering of the host affinity towards the guest molecule or through lowering the stability of the linker via chemical modification.
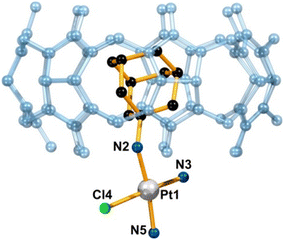 |
| Fig. 7 Supramolecular structure of a host–guest complex consisting of a Pt-containing ADA derivative and CB[7] determined by X-ray diffraction analysis. Hydrogens, counterions, and water molecules are omitted for clarity. Reproduced with permission from ref. 173, the American Chemical Society, 2021. | |
CB[7]'s in vivo biocompatibility was also evaluated on a zebrafish model, which revealed a low toxicity of CB[7] to zebrafish.165 The results show that CB[7] exhibits measureable cardiotoxicity and toxicity at concentrations of 0.50 mM or higher, without significantly observable change on hepatotoxicity phenotype at concentrations up to 0.75 mM.165 Subsequently, a systemic evaluation of the in vivo biocompatibility of CB[7] was conducted on a mice model as well.166 The maximum tolerable single doses of administered CB[7] on mice were 5 g kg−1 orally, 500 mg kg−1 peritoneally, and 150 mg kg−1 intravenously, respectively. There were no significant differences among various groups of mice in terms of body-weight compared to the control group, although there was a small decrease in body-weight within the first three days (Fig. 8). It was concluded that even at higher doses of administrated CB[7] there were no noticeable toxicity effects.165,166
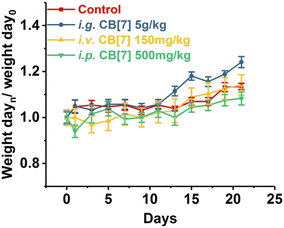 |
| Fig. 8 Body weight changes of mice i.g. (orally), i.v. (intravenously) or i.p. (peritoneally) administered with CB[7], respectively, monitored for 21 days post-administration. Reproduced with permission from ref. 166, Nature Research, 2010. | |
Very recently a systematic evaluation of the CB[7] pharmacokinetics and toxicity was performed to evaluate specific organ toxicity, i.e., on plasma, brain, kidney, and gastrointestinal tract by various methods of injection (intramuscular, intraperitoneal and intragastric).174 Only minor liver damage was observed when CB[7] was administered intragastrically. Overall, parenteral administration led to fast elimination from blood and minimal absorption from the gastrointestinal tract and was well tolerated even when repeated. Unfortunately, kidney damage was observed and 3.6% of animals showed signs of nephrotoxicity which may be the most critical drawback of its parenteral use, since most of its elimination from the body is through excretion by kidneys.174 Nevertheless, toxicological profiles of CB[n]s in general, even acyclic-CB[n] and CB[n]-type175 ones, support the idea of their exploitation in biological applications at subtoxic concentrations. Although CB[n]s seem to be promising molecules, long-term side effects from continuous use of CB[n]s are still relatively unknown and should be determined, which is also one of the reasons that CB[n]s are presently not in clinical use and have not yet been tested in humans.
Note that CB[n] macrocycles and their complexes successfully cross the cell membranes, which is typically visualized by fluorescence microscopy that additionally confirms their already observed excellent bioavailability. Moreover, even large CB[n]-based nanoparticles176 (∼190 nm) or CB[n]-carbohydrate clusters177 can successfully cross the cell membrane by receptor-mediated endocytosis and release the loaded drug into cytoplasm after endocytosis, thereby increasing the pharmaceutical effects of the drugs on a target cell. In another study CB[7] complexes with fluorescents dyes acridine orange and pyronin Y successfully penetrated the cell membrane of mouse embryo muscle cells, demonstrating that other CB[n] host–drug complexes are likely to behave in vivo in a similar fashion.178 CB[7] molecules conjugated to cyanine and rhodamine dyes also displayed uptake into live cells (human breast carcinoma cells, MCF-7) via multiple pathways but mainly by clathrin-mediated endocytosis and passive diffusion (Fig. 9).179 Most of the CB[7]-dye molecules accumulated in lysosomes were afterwards excreted from cells via a lysosome-associated exocytosis, involving the fusion of lysosomes with the plasma membrane rather than Golgi-mediated pathways of excretion for foreign materials.179 Note, however, that the free molecules of dyes were excreted by the Golgi mechanism. All these findings demonstrated that the CB[7] moiety indeed had a substantial influence on the uptake and excretion pathways of molecules, making it suitable as a molecular probe for a wide variety of applications in bioimaging.179 Even more, by using live-cell imaging it was revealed that treatment with subtoxic CB[7] amounts did not have damaging effects on the cellular integrity, as assessed by mitochondrial activity.162
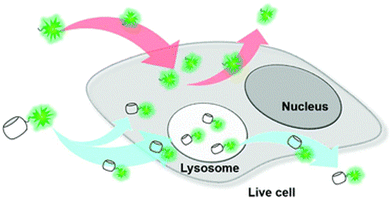 |
| Fig. 9 Illustration of intra- and extracellular translocation of dyes. Reproduced with permission from ref. 179, the Royal Society of Chemistry, 2019. | |
In their toxicity and drug potential study, Isaacs and Briken163 synthesized compounds that consisted of a signaling subunit, such as fluorescein or Alexa Fluor 555, that was then attached to spermidine and 1-aminoadamantane subunits in order to facilitate tight binding with CB[n] or CB[n]-type compounds. Their rationale was that the hydrophobic part of the molecule (ADA or alkyl chain) will provide a good anchor in the CB[n] cavity while the fluorescent part of the molecule can be used to track these CB[n] molecular containers inside cells in real time. Co-localization assays using fluorescence microscopy showed an uptake of the studied ADA-derivative/CB[7] and Dextran-647 into murine macrophage cells with little co-localization after 15 min (Fig. 10A). However, at 45 min, an increase in CB[7] co-localization with Dextran-647 was observed (Fig. 10B). The complex in question had intracellular stability for more than 2 hours.163
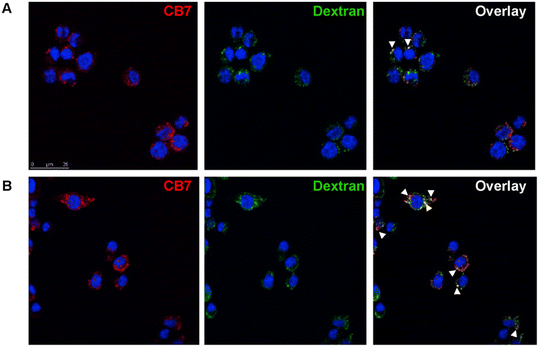 |
| Fig. 10 Intracellular localization of CB[7] in RAW264.7 cell. Cell incubated with Dextran-647 and ADA-derivative/CB[7] showed intracellular localization of CB[7] through the endosomal pathway. RAW264.7 cells were incubated with Dextran-647 (green) overnight and ADA-derivative/CB[7] (red) for 20 min the following day. Cells were chased for 15 (A), 45 (B) min after incubation with ADA-derivative/CB[7]. Arrows indicate co-localization. Reproduced with permission from ref. 163, Public Library Science, 2010. | |
Recently an ADA derivative coupled with a fluorophore nitrobenzoxadiazole (NBD-Ad) was used as a probe that efficiently labels the endoplasmic reticulum (ER) in living cells (Fig. 11).180 CB[7] time-dependent imaging revealed a loss in specificity for ER by observing a reduction in the fluorescence signal, which was a useful proof-of-principle that showed how sub-cellular localization of a probe can be monitored using the supramolecular approach.180
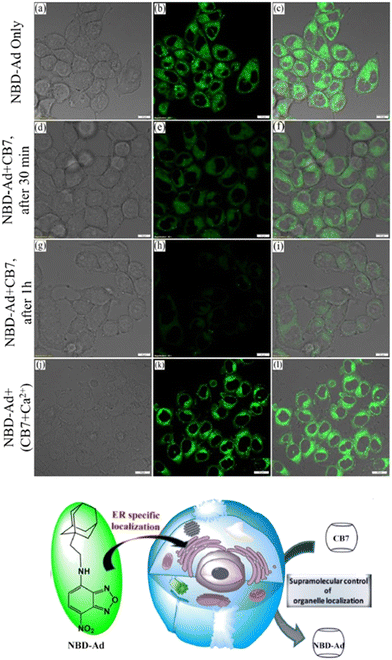 |
| Fig. 11 Fluorescence co-localization microscopy images of live HeLa cells stained with 2 μM NBD-Ad (a–c, up), after 30 min of 20 μM CB[7] post-treatment (d–f, middle), after 1 h of CB[7] post-treatment (g–i, bottom), and (j–l) post-treatment with the Ca2+/CB[7] complex with a retaining of the NBD-Ad in the ER. Reproduced with permission from ref. 180, Elsevier, 2021. | |
3.2. Properties of CB[n]-drug systems
A comprehensive study of broad chemical, physicochemical and biological parameters should be undertaken before employing a supramolecular system in medicine. Especially important is to conduct a thermodynamic study, primarily the investigation of interactions between host and guest molecules with a goal of designing effective functional materials or systems for targeted bioapplications. Key aspects of host–guest behavior to be considered in this context are binding affinity and selectivity toward specific guest molecules, host–guest complementarity, selective and directional nature of intermolecular interactions, etc. Note that the supramolecular CB[n]-drug system possesses unique properties that can contribute to drug protection, stability, and its bioavailability. The first step of the molecular recognition process is unsurprisingly the inclusion of a drug within the hydrophobic cavity of cucurbit[n]urils which provides protection against environmental factors such as light, heat, oxygen, etc. The inclusion phenomenon enhances the solubility of the lipophilic drug in a water environment and can also prevent drug degradation. Maintaining drug stability over time can be accomplished by tailoring complexes of high stability. In the end, improved solubility and stability of drugs within the CB[n]-drug system can positively influence drug molecule's bioavailability. This is particularly important for drugs with low aqueous solubility where enhanced bioavailability can contribute to better therapeutic outcomes. Additionally, encapsulation of certain drugs within CB[n]s may reduce their toxicity by controlling their release and preventing excessive exposure to healthy tissues. Overall, interdisciplinary research studies of supramolecular principles of inclusion complexes in pharmacology significantly contribute to the development of advanced drug delivery systems, improved drug formulations, and enhanced therapeutic outcomes which makes this field very active and also challenging.98,126
As already mentioned, CB[n]s are chemically and thermally exceptionally stable compounds that come in a variety of sizes and offer a broad range of cavity dimensions for potential pharmacophores. The only drawback of using CB[n]s as drug carriers or in biological applications in general is their poor solubility in water when compared to cyclodextrins or sulfonated calixarenes. However, with the optimization of functionalization methods66,67 or by changing the media, like adding biocompatible salts, or by changing the pH, CB[n] hosts can overcome their inherent solubility issues. On the other hand, these protocols often decrease complex stabilities due to the occurrence of competitive binding to the portals.181 Regarding the solubility of CB[n]s in various body fluids, it has been established that they are soluble in range of 1–4, 5–7, 34–45, and 33–37 mM in gastric,182 intestinal, nasal fluids and blood plasma, respectively.143 Moreover, mutual influence on solubility is established upon binding of the drug. Note that exploring modulation of chemical and biological properties of medically relevant guest molecules within a complex is crucial for designing CB[n]-based drug delivery systems with properties tailored to meet specific therapeutic requirements, as can be seen from the listed literature examples.98,126 In practice, this means that CB[n]s increase water solubility of the hydrophobic drug and vice versa encapsulation of already water soluble drug molecules can increase solubility of CB[n]s in a water environment.98 Increased solubility of drugs or bioactive compounds is achieved through host–guest interactions, as was observed for the complexation with either CB[n]s98 or acyclic-CB[n]s.183 Excellent examples of this are fungicides and anthelmintic drugs benzimidazole and its derivatives albendazole, carbendazim, thiabendazole, and fuberidazole which all have low solubility in water and lower affinities for CB[7] when unprotonated, traits that significantly diminish their use (Fig. 12).184,185 However, when these species are protonated, complex association constants become larger and preferential binding increases the pKa values of the conjugate acids of these drug molecules by 2–4 units, consequently improving their solubilities.184,185 These complexes are therefore illustrative examples of the already mentioned pKa induced complexation.97–99 Additionally, encapsulation in CB[7] also enhanced photostability of fuberidazole and thiabendazole,184 while the insoluble anticancer drug camptothecin upon encapsulation in CB[7] and CB[8] increased its solubility about 70 and 8 times, respectively.186 Lastly, encapsulation of a guest molecule into CB[n]'s cavities can even prevent thermal degradation of the guests.187–189
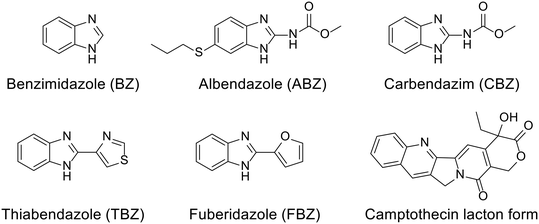 |
| Fig. 12 The studied benzimidazole drug series and camptothecin. | |
Wheate et al. examined the host–guest chemistry of four orally delivered drugs used in the treatment of human diseases, including also memantine, an Alzheimer's NMDA glutamate receptor drug.187 Although inclusion strength of memantine into CB[n]s was already determined109 and is compiled in Table 2, the authors undertook an exhaustive thermodynamic study of this system which included NMR and kinetic exchange data. Analysis of suitable monocrystals of the memantine hydrochloride/CB[7] revealed that memantine is fully bound within the CB[7] cavity and the complex is further stabilized by two hydrogen bonds (1.95 and 2.21 Å) between the guest's amine group and two separate carbonyls of the CB[7] portal (Fig. 13). This total encapsulation and strong interactions resulted in increased thermal stability of the supramolecular system, evidenced by a substantial melting point increase compared to the free drug (no melting observed below 380 °C).187 Prolongation of thermal stability has important implications for the prevention of drug degradation during its processing, formulation and storage.
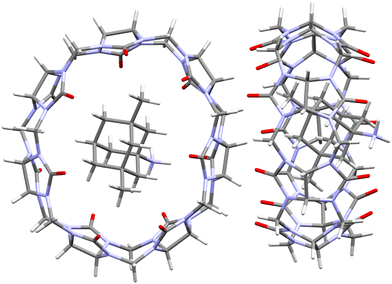 |
| Fig. 13 Single crystal X-ray diffraction structure (CCDC 746893) of the memantine hydrochloride/CB[7] complex.187 | |
Modulation of chemical and physical properties can improve drug stability since shielding in the cavity effectively prevents chemical reactivity (oxidation, hydrolysis), which is a common drawback that lowers drug bioavailability in vivo. This shielding effect is especially obvious in case of chemotherapeutic platinum-based drugs. Namely, it was observed that even partial encapsulation of a platinum drug within the cavity of a CB[n] can provide sufficient steric drug protection from nucleophilic attack from the compounds which are present in the human body in large amounts, like for example thiol peptides and proteins.190,191 Examples of drugs or biologically active compounds that have been studied in combination with CB[n] hosts are numerous and span a wide range of applications, for example, anaesthesia,192,193 treating infections,188,194–197 anticancer drugs,164,186,189,190,198 neuromuscular blocking agents (NMBAs),199–201 biodiagnostics,202,203etc.
3.3. Drug release
Recall that an ideal drug delivery vehicle should not only have the ability to bind and protect a biologically active compound but it also has to overcome multiple biological barriers and finally release the drug molecule in a target cell, preferably in a slow and controlled manner.204 As illustrated in Fig. 14, drug release from a CB[n] cavity can usually be achieved by various methods which employ thermodynamic basis of interactions needed for complex formation. Since host and guest molecules create a complex with non-covalent interactions which are by nature weak and reversible, the real challenge of applying such a system is to achieve a balance between stable encapsulation and efficient release. In exceptional cases CB[n]s make complexes with host molecules with ultra-high affinity binding, but at a cost of very slow complex dissociation.107,108,111 In general, bioapplicable complexes are in the millimolar to micromolar affinity range, with dissociation rate constants of the order of seconds or faster. The dilution effect is a primary release mechanism of a biologically active compound happening soon after administration. It is known that the degree of complexation depends on the absolute concentrations of the host and the guest. Therefore, upon dilution the percentage of a complex will decrease as soon as the complex “enters” into a diluted region, i.e., region with a smaller concentration of the complex.
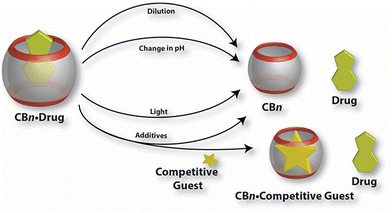 |
| Fig. 14 Illustration of various mechanisms for the release of drug molecules from drug/CB[n] complexes. Reproduced with permission from ref. 146, Frontiers Media SA, 2019. | |
Further mechanism that could be applied for drug release is a simple change of the pH of the environment because it can easily trigger drug decomplexation. The previously mentioned CB[n]-induced pKa shifts97–99 can be used to trigger ejection of the molecule from the macrocyclic cavity, which is possible because CB[n]s have a much higher binding affinity for the protonated form of the drug when compared to its neutral form. Besides the influence of the pH of the biological medium, guest molecules can also be released by a phototriggered pH jump.202,205–207 A good example of that mechanism is a phototriggered reaction of a weakly binding neutral trans-chalcone that photochemically transforms into a flavylium cation, which is a strongly binding competitor and successfully displaces memantine from the CB[7] cavity in a water environment (Fig. 15).206 The authors also demonstrated that memantine can even be partially re-complexed if a thermally activated back reaction occurs, effectively reversing the supramolecular process.
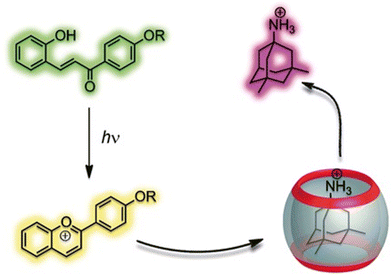 |
| Fig. 15 Controlling host–guest equilibrium with a flavylium-based photoswitch leads to efficient light-triggered release of memantine. Reproduced with permission from ref. 206, Wiley VCH, 2011. | |
Another way often used in releasing molecules captured in macrocyclic cavity is by competitive binding of inorganic cations208 to the portals which are already present in biological fluids or through displacement with another molecule that has a higher affinity for inclusion in the cavity. Bhasikuttan et al. demonstrated how precise control over the formation of the complex and release of the guest is challenging.209 They showed that thioflavin ThT, a fluorescent dye molecule used to diagnose neurodegenerative diseases, and CB[7] can yield two types of complexes with different host
:
guest stoichiometry, a 1
:
1 and a 2
:
1 complex (Fig. 16). Note that upon addition of the alkali or earth alkaline metal ions on the 1
:
1 and 2
:
1 systems different processes occur. In the 1
:
1 complex metal ions can bind to the host portals and thioflavin ThT is displaced from the cavity due to competitive binding. On the other hand, in the 2
:
1 complex the same metal ions serve the purpose of bridging vehicles between the two carbonyl rims of the macrocycles, forming a rather unusual assembly that is characterized by increased complex rigidity and stability, therefore causing its fluorescence. By addition of amantadine hydrochloride, an even stronger competitor, the fluorescent capsular complex is disassembled, thioflavin ThT released and a more stable amantadine/CB[7] complex is formed.209,210
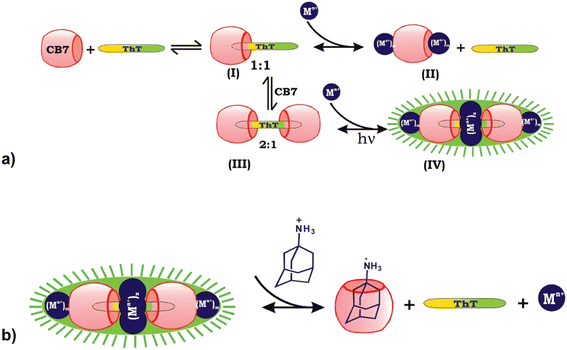 |
| Fig. 16 a) Binding interactions of the thioflavin ThT, CB[7] and metal ion system afford a highly fluorescent supramolecular capsule, (I) the 1 : 1 complex, (II) ion lids formation, (III) the 2 : 1 complex, and (IV) assembly of the cationic charges and sealing of the space between two CB[7] units. b) Release mechanism of ThT from the metal ion-bound supramolecular capsule by adding amantadine hydrochloride as a competitor. Reproduced with permission from ref. 209, the American Chemical Society, 2010. | |
Very recently, even multi-step competitive displacement chemically triggered by memantine was applied (Fig. 17).211 The demonstrated inter-complex reactions show some resemblance to biological systems and mimic how chemical information is communicated along a pathway in a cell. The cascade of displacements between three guest species and two CB[n]s is very well defined by thermodynamic characteristics of all involved host–guest complex equilibriums.211 In general, researchers often apply ADA derivatives as displacement guests in drug release processes,209,210,212–218 especially amantadine or memantine, that are in turn also well-known drugs for viral infections, Alzheimer's disease, etc.10 This feature is especially attractive when using drug targeting to produce a combined activity from two drug species.
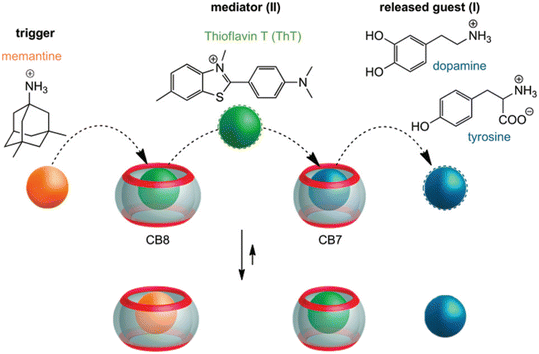 |
| Fig. 17 Chemically-triggered supramolecular communication cascade with memantine as the trigger, thioflavin ThT as the mediator and tyrosine or dopamine as the finally released guest. Reproduced with permission from ref. 211, the Royal Society of Chemistry, 2020. | |
On another note, supramolecular approach to PEGylation (covalent conjugation of polyethylene glycol) was used in controlling the insulin release vs. standardized covalent PEGylation in which the protein in question is permanently modified and thus can have reduced activity and increased immunogenicity. Namely, as a proof-of-principle it was shown that by varying different host–guest affinities it is possible to tune the pharmacokinetic profile of the protein in question. Again, the ADA/CB[7] system was chosen because of its excellent binding strength and the complex was thus associated from the ADA scaffold bound to PEG20k and CB[7]-modified insulin.219
3.4. Bioapplication of ADA/CB[n]
Supramolecular regulation of intracellular events or tuning of biological functions in which supramolecular systems cooperate to some extent has potential future application in biological systems. Adamantane and cucurbit[n]uril molecules as well as their subsequent supramolecular assemblies have the potential to modulate various cellular functions and they are not limited only to the most commonly used drug delivery and controlled release applications. Survival and functionality of supramolecular complexes within a cellular environment are critical considerations for their application in various biomedical contexts, as can be seen from the examples herein, with ADA/CB[n] having a great advantage due to its inherently high stability constant. The real challenges for supramolecular complexes chosen to be exploited in living cell are not only to fulfill the basic requirements of biocompatibility, stability, resistance to degradation and efficient cellular uptake but also to preserve their endurance and function within the complex in a dynamic cellular environment. Assemblies designed in a specific manner in order to reliably interact with each other can modulate signaling pathways and cellular responses or influence intracellular signaling cascades to regulate cellular functions and processes. Incorporation of imaging agents into the ADA/CB[n] system, e.g., fluorescent probes, for cellular imaging purposes can help in tracking and visualization of the assembly within cells. Additionally, using the assembly for diagnostic imaging enables identification of specific cellular markers or abnormalities in tissues. Previously discussed examples showed how designed assemblies can respond to specific cellular stimuli (e.g., pH, temperature, enzymatic activity), allowing for a dynamic and responsive behavior within the cellular microenvironment. Researchers in this field aim to design complexes that can precisely interact with cellular components, providing a platform for therapeutic interventions, diagnostics and understanding of fundamental cellular processes.
Protein–protein interactions are fundamental to the functioning of cells, regulating diverse important physiological functions at a cellular level and playing a crucial role in signal transduction, gene expression, cellular structure, and other essential functions. Protein recognition processes can occur throughout multivalent engagement between separated binding sites that can even cooperate in a synergistic manner and thus induce enhancements of overall binding affinity. Reliable and efficient host–guest interactions between CB[7] and ADA serve as a model system to test whether multivalent interactions will still occur at larger distances between the binding sites, thereby contributing to the intricate network of cellular pathways, and help unravel fundamental cellular processes.220 For that purpose stable and long DNA scaffolds with two attached CB[7] units and two various ADA ligands at the distances varied from 70 to 360 Å were used in order to explore the distance-stability relationship and its limitations in complexes formed upon bivalent interaction (Fig. 18).220,221 The scaffolds were additionally fluorescence-labeled to assess the interactions (cross-linking) between CB[7] and the ADA moieties. It was found that the affinity gain provided by bivalency is indeed dependent on and controlled by the strength of individual monovalent interactions, by the distance between host–guest pairs and the overall flexibility of the scaffold. This suggests that binding systems of low affinity and distant sites will be more likely cross-linked via long flexible tethers, thereby enabling a nearing of the respective DNA strands.220
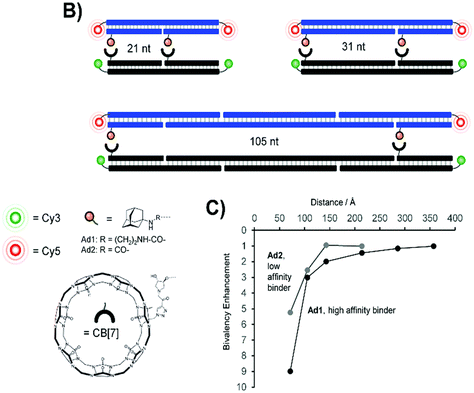 |
| Fig. 18 Bivalent library with separated ADA/CB[7] host–guest pairs (top, B) and distance dependency of bivalency-enhanced interactions between distance-matched CB[7] and ADA displays (bottom, C). Reproduced with permission from ref. 222, the Royal Society of Chemistry, 2020. | |
A recent report details how host–guest chemistry was employed in locally administrated targeted drug delivery.223 The idea was that a CB[7]-rich injectable hydrogel would be applied into the tumor tissue and would be used to facilitate drug localization through high affinity that would lead to substantial drug aggregation at the target site (Fig. 19). In order to validate the effectiveness of this strategy, the authors first synthesized test molecules which consisted of the anchoring group for the CB[n] cavity and a fluorophore replacing the drug at its defined position in the compound. The ADA/CB[7] system was used as a model of a complex with moderate association constant while ferrocene/CB[7] was labelled as a strong guest complexing agent. After repeated administrations, fluorescence in the target site increased linearly, suggesting that the proposed strategy was indeed efficient. However, since the ADA/CB[7] system had lower targeting loading than the corresponding ferrocene, the latter was chosen for the in vivo experiments in combination with the drug doxorubicin. Nevertheless, these findings illustrated that substance aggregation in tumors was significant, which has implications for possible higher dosing of active species at the tumor site without potential concomitant issues from off-site toxicity.
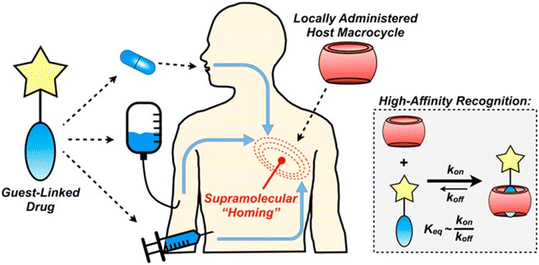 |
| Fig. 19 Supramolecular homing of guest-appended small molecules on the basis of affinity for locally applied host macrocycles. Reproduced with permission from ref. 223, the American Chemical Society, 2019. | |
Going further, in the next example authors take advantage of non-covalent interactions between CB[7] and synthetic amine derivatives that exceed biological macromolecule affinity for their targets by taking control over the enzymatic activity of bovine carbonic anhydrase (BCA) or acetylcholinesterase (AChE).224 Synthesized inhibitors consisted of two binding regions, an enzyme-binding group and a macrocycle-binding group. The first domain contained a benzenesulfonamide group which is a known inhibitor scaffold responsible for binding into the enzyme active site and the second domain contained 1-aminoadamantane, trimethylsilylmethyl amine or hexylamine responsible for docking into the CB[n] cavity. As shown in Fig. 20, addition of CB[7] to a catalytically inactive form amine/BCA afforded a ternary transient complex amine/CB[7]/BCA that underwent rapid dissociation to a free enzyme, which thereby restored the activity along with producing a supramolecular complex of the synthesized inhibitor in a CB[7] container, amine/CB[7]. By adding a strong competitor (the ADA guest derivative) into the systems, the inhibitor molecule is displaced from the CB[7] and thus regenerated to settle again into the active site of the enzyme, which is in turn inhibited again. The authors state that on–off switching of the catalytic activity can be repeated in several cycles and can be monitored by combined spectroscopic techniques such as UV/vis, fluorescence and 1H NMR. On the other hand, upon addition of CB[7] to the inhibited AChE no recovery of enzyme activity was observed. A possible explanation of that finding could be that relatively open active and peripheral sites of AChE can accommodate both inhibitors and their CB[n] complex, as shown in Fig. 20. Competitive binding of the two-faced inhibitors with enzyme vs. CB[7] was thus used as a controlling factor for enzyme activity. The authors highlight that this principle is different from typical allosteric regulation of enzyme activity found in nature where a small molecule typically binds to a site distant from the enzyme's active site with a resulting decrease or increase of enzymatic activity.
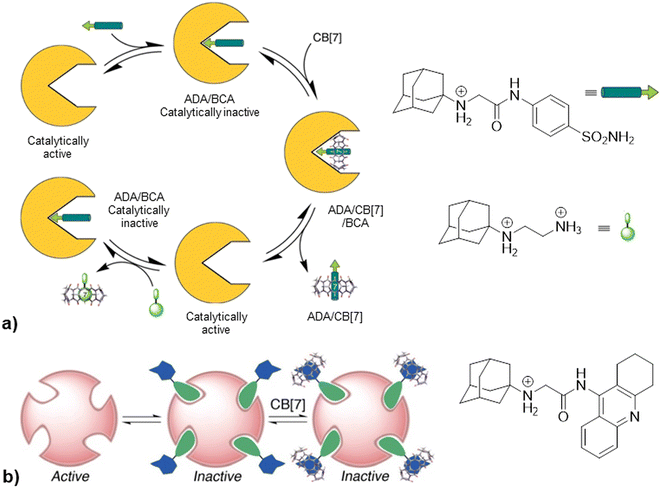 |
| Fig. 20 a) Representation of the control of enzymatic activity of BCA based on enzyme and CB[7] competition for common inhibitor, b) formation of ternary complex upon addition of CB[7] to ADA/AChE. Reproduced with permission from ref. 224, the American Chemical Society, 2010. | |
Host–guest recognition between adamantane and cucurbit[n]uril offers a versatile platform for designing DNA-based systems with enhanced functionalities. Here we will mention several examples in which the ADA/CB[7] system can be employed in conjunction with DNA to control activities of enzymes within cells, to regulate and intervene in DNA processes, etc. The CB[n] macrocycle was recently, for the first time, covalently linked to a DNA-small molecule chimera while the ADA scaffold was linked to another DNA chain with the aim to use the emerging host–guest complexes to control the activity of carbonic anhydrase II (CAII) (Fig. 21).225 Adenosine 5′-triphosphate (ATP) was used as a trigger that led to the displacement of a protein inhibitor guest molecule bound within a CB[7] host. The event was accomplished by combination of the ATP-induced DNA duplex assembly and intramolecular host–guest interactions. Note that once again the strong interactions of the supramolecular ADA/CB[7] complex are used to control the inhibition of CA-II.
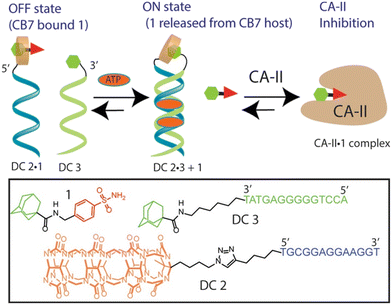 |
| Fig. 21 A DNA-small molecule chimera (DC) transducer based on a split DNA aptamer that converts an ATP input into functional release of a CA-II inhibitor ADA from the CB[7] host. Reproduced with permission from ref. 225, the American Chemical Society, 2017. | |
Similarly, in 2020 a group of authors reported on an ADA/CB[7] assisted epigenetically active DNA binding system that mimics transcription factors pair and recruits the epigenetic modifier to a particular DNA target site (Fig. 22).226 The approach of the host–guest system is believed to happen first, followed by synergic DNA binding.
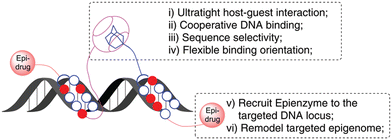 |
| Fig. 22 Illustration of an ADA/CB[7] assisted epigenetically active DNA binding system. Reproduced with permission from ref. 226, the Royal Society of Chemistry, 2020. | |
Employment of CB[7], spermine, and 1-aminoadamantane has recently been studied as a supramolecular platform for the regulation of a DNA transition process, as shown in Fig. 23.227 Right-handed helical DNA (B-DNA), which is more favored and thermodynamically more stable, could be converted into left-handed helical DNA (Z-DNA) in the presence of spermine. This transition process could be reversed upon addition of CB[7] which binds spermine and thus induces a conversion of Z-DNA to B-DNA. Reversely, active spermine could be again released upon addition of 1-aminoadamantane which is a CB[7] binding competitor and therefore readily induces reconversion of the B- into the Z-DNA helical structure. Additionally, the authors noted that a cycled transition could be achieved by repeatedly adding CB[7] and 1-aminoadamantane.
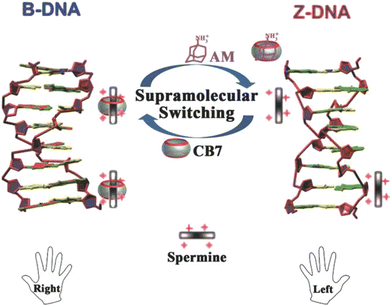 |
| Fig. 23 A CB[7]-based supramolecular approach for reversible B/Z-DNA transition. Reproduced with permission from ref. 227, Wiley VCH, 2018. | |
In the next example DNA was modified with ADA-functionalized 5-formylcytosine (5fc-AD) which served as a binding anchor for the CB[7] cavity.228 By utilizing supramolecular interactions of the ADA/CB[7] system it was possible to reversibly intervene in biochemical activities of the DNA, including restriction endonuclease digestion, DNA polymerase elongation, and polymerase chain reaction. It was also demonstrated that steric hindrance of the rigid CB[7] prevents the enzyme from binding to the substrate, whereas the CB[7]/5fC-AD host–guest interactions can be reversed by treatment of the system with a competitor substrate like 1-aminoadamantane (Fig. 24).
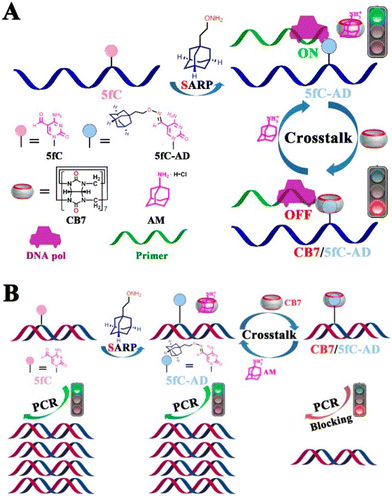 |
| Fig. 24 Reversible intervention of 5fC-targeted DNA pol elongation and PCR reaction (A and B). The binding of CB[7] to 5fC-AD nucleotide is assumed to generate large steric hindrance that prevents the approach of DNA pol and inhibits enzyme activity. The CB[7]/5fC-AD host–guest interactions can be reversed by treatment with 1-aminoadamantane. Reproduced with permission from ref. 228, the American Chemical Society, 2017. | |
Jayawickramarajah and coworkers broadened the toolbox of dynamic DNA chemistry and nanotechnology by introducing a synthetic surrogate and utilizing ADA/CB[7] interactions for DNA strand displacement. A classical concept of base-pair-driven toehold-mediated strand displacement (BP-TMSD) was replaced with host–guest-driven toehold-mediated strand displacement (HG-TMSD), which showed possibilities for enzyme activity control and layered reactions that detect specific microRNA.229
Efficient protein purification can be laborious work and has many drawbacks such as protein denaturation, contamination, low scale, etc. Kim's group recently proposed a scalable method for affinity purification of large quantities of recombinant therapeutic proteins with the aim of utilizing supramolecular interactions of an ADA/CB[7] system (Fig. 25).230 Herceptin, an antibody drug used to treat breast cancer, was first site-specifically tagged with 1-aminoadamantane via an enzymatic reaction. A highly specific and selective inclusion of the ADA scaffold into the CB[7] cavity was used for binding with the CB[7]-conjugated agarose beads. Lastly, the protein was recovered in high purity using a competitive guest affinity for binding into the CB[7]. The stable and robust CB[7]-beads can be recycled up to 4 times by treatment with concentrated salts. Here it should also be mentioned that quite recently a new strategy of a ADA/CB[7] protein assembly for affinity purification of methyllysine proteomes has been successfully employed.231
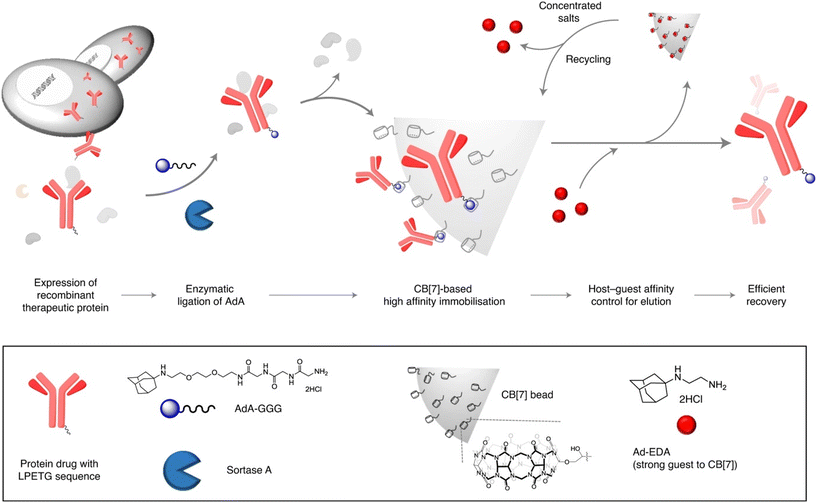 |
| Fig. 25 Recombinant therapeutic proteins with the LPETG sequence expressed in cells are tagged with ADA–GGG via sortase A ligation and the tagged proteins are then selectively captured on CB[7]-conjugated beads and efficiently recovered via strong guests. Reproduced with permission from ref. 230, Nature Research, 2020. | |
Development of oxidative stress related diseases is often associated with the mitochondrial fission, since the fragmentation of mitochondria membrane advances production of reactive oxygen species and promotes apoptosis. The first example of an artificial pathway to induce mitochondrial fusion via supramolecular mitochondrial aggregation was proposed to be the inception of a completely different approach when compared to traditional methods of fusing mitochondria by manipulating specific proteins.232 Namely, employment of ADA/CB[n] system in engineering living cells is possible because of its precise design where the puzzle pieces interact specifically with each other and allow for a precise control over cellular processes. First, mitochondria are surface-modified with a guest species, i.e., adamantane along with polyethylene glycol (PEG) system that was tagged with triphenylphosphonium (TPP) (Fig. 26). Afterwards the addition of CB[7] modified with hyaluronic acid (HA) induced supramolecular aggregation and subsequent fusion of mitochondria via ADA/CB[n] strong host–guest interactions that were employed to cross-link several mitochondria together. It should be noted that the chemically stressed SH-SY5Y cells and zebrafish neurons were effectively protected when this supramolecular mitochondrial fusion strategy was applied in vitro and in vivo, respectively, suggesting that the described approach may be applicable in a broader sense in the future.
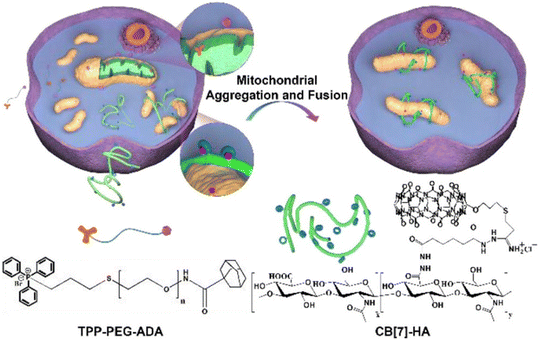 |
| Fig. 26 Illustration of supramolecular mitochondrial aggregation and fusion. Reproduced with permission from ref. 232, American Chemical Society, 2020. | |
Furthermore, by functionalizing the ADA/CB[n] assembly with ligands for specific cell types one can enable targeted interactions or by tailoring the assembly for disease-specific markers achieve targeted therapy. Recently, Wang and coworkers demonstrated that supramolecular recognition can be used to anchor drug-loading liposomes (L) on macrophages (M) for cell-hitchhiking drug delivery where “hand-holding” and “marriage” between CB[7] and ADA functionalized on the surface of the macrophage and liposome, respectively, was the driving force to obtain the M–L conjugate (Fig. 27). It was found that the resulting complex remained stable for up to at least 4 h, which was sufficient for in vivo hitchhiking delivery of toxic drugs loaded in a liposome to the tumor, consequently enhancing the chemoimmunotherapy of melanoma in mice. The authors concluded that the CB[n] and ADA systems which are stable for months could have potential in clinical translation, for example in a form of a biological kit consisting of drug-loaded ADA liposomes and CB[7] functionalized moieties present as two separate reagents.233
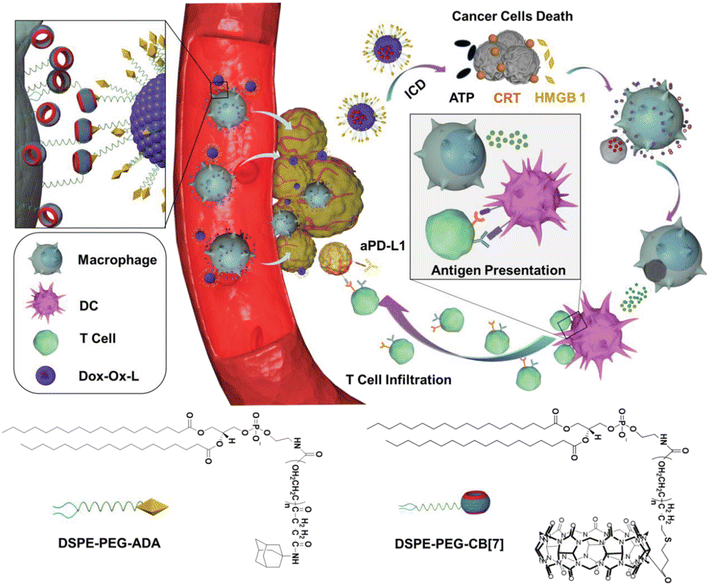 |
| Fig. 27 Illustration of a cell-hitchhiking drug delivery enabled by host–guest interactions between CB[7] modified macrophage and ADA modified liposome, leading to melanoma cell death. Reproduced with permission from ref. 233, Wiley VCH, 2021. | |
Research in this area is dynamic and advancements in supramolecular chemistry are continually expanding the possibilities for utilizing assemblies like tightly bound ADA/CB[n] complexes in cellular and organismal regulation. However, it is essential to approach these applications with a thorough understanding of cellular processes, biocompatibility and safety considerations.
3.5. Bioanalytics of ADA/CB[n]
Chemosensing in biological systems, especially physiologically relevant ones, is highly challenging due to nanomolar to micromolar concentration range of the analyte of interest and also due to interference of highly competitive substrates present in such environment. Cucurbit[n]uril host–guest complexes have also emerged as promising tools for biologically relevant analytical135–137 and diagnostic applications, owing to their stable and specific interactions, bio-orthogonality in binding, refined synthesis, thermodynamic stability, etc., but most importantly due to their high selectivity and strong binding. CB[n]-based molecular sensors have lately been employed as indicator displacement assays (IDAs)234 for detection of various ADA derivatives in biological environmental conditions, e.g., for acetyl amantadine235 or amantadine236 in urine, amantadine in human serum237,238 and memantine in blood serum239 or in biofluids.240 Unlike direct binding assays (DBA) where binding of an analyte to the CB[n] cavity yields a spectroscopic response but is not very selective, in IDAs the CB[n] cavity is pre-complexed with a fluorophore or a chromophore molecule which is upon analyst binding displaced and shows observable specific response (Fig. 28). In continuation selected examples of IDAs with ADA/CB[n] systems where the ADA derivative is a drug or a metabolism product will be presented.
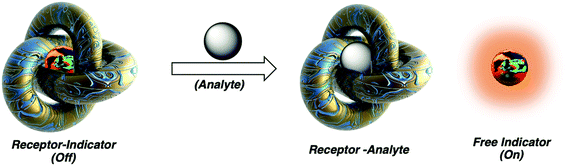 |
| Fig. 28 Analyte mediated displacement of an indicator from a supramolecular host. Reproduced with permission from ref. 234, Royal Society of Chemistry, 2021. | |
Recently it was proposed that analysis of acetyl amantadine (AcAm) in urine could serve as a diagnostic tool to measure the spermidine/spermine N1-acetyltransferase (SSAT) activity.234 The ADA derivative in question is exclusively a product of the metabolism of amantadine (Am) upon catalysis with SSAT, which in turn is known as a biomarker for multiple aggressive cancers. The authors used the IDA detection concept of fluorescent indicators bound to CB[n] that was afterwards replaced with AcAm due to the ADA/CB[n] system's capability for strong and selective binding (Fig. 29a). The limit of detection of AcAm was 0.087 μM with a linear response range from 0 to 1 μM. This study is therefore a proof-of-concept for designing similar analytical testing methods, however, since quantitative sensing was not achieved it is not yet of clinical relevance.
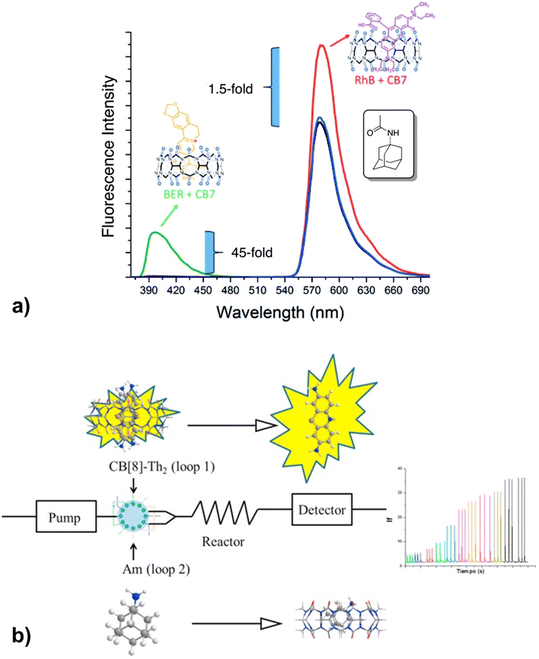 |
| Fig. 29 a) Displacement of rhodamine B (RhB) and berberine (BER) from CB[7] during fluorescence titration with acetyl amantadine (AcAm) in deionized H2O and b) amantadine determination in human serum using a fluorometric flow injection analysis system. Reproduced with permissions from ref. 235, Canadian Science Publishing, 2016 and from ref. 237, Elsevier, 2018. | |
In another approach, a competitive assay between the thionine dye and amantadine for the CB[8] macrocycle was constructed.237 Indirect fluorescence detection of amantadine was based on a competitive reaction with the fluorescent probe for the inclusion within the CB[8] cavity, but was carried out in a flow injection spectrophotometric analysis system (FIA) (Fig. 29b). This method allows for detection of amantadine in pharmaceutical formulations and in human serum samples with excellent recoveries, ranging from 83 to 98%, depending on the nature of the matrix assayed. Even more, the selectivity of the method was evaluated against different antiviral drugs: rimantadine, acyclovir and ribavirine. According to the authors, this was the first time that a CB[n] supramolecular system has been employed for an analytical determination in a FIA system.
In 2019 Biedermann et al. published IDAs based on CB[8] that were capable of detecting memantine in a blood serum.239 The operational system was designed after mathematical simulations and a new class of [2.2]paracyclophane-derived indicator dyes was developed, possessing both excellent binding for the CB[8] host as well as good spectroscopic properties. Potential competitive binding of biofluids through the IDA was therefore overcome and quantification of memantine in blood serum could be successfully achieved. Afterwards other covalently bound CB[n]-indicators for amantadine quantification in biofluids were also designed, surpassing fundamental limitations of IDA like impractically slow equilibration time.241,242
Another illustrative example of two-fold implementation is an ADA/CB[n] system where an adamantyl-bearing 1,2-dioxetane acts as a chemosensor enclosed into the CB[n] cavity and also works as IDA for low micromolar memantine detection in human urine and human serum samples (Fig. 30).240 The authors showed that CB[8] can serve as a versatile chemiluminescent enhancer and moreover that this approach can be readily adaptable to a commercially available dioxetane, having the potential to improve chemiluminescence-based diagnostic assays.
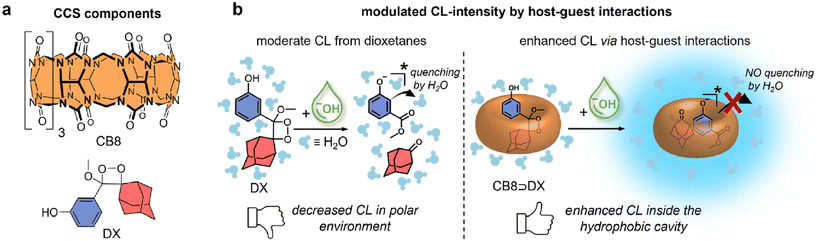 |
| Fig. 30 Illustration of the host and guest structures (a) and the role of their interactions in a supramolecular assay based on a chemiluminescent chemosensor (b) possessing an ADA subunit. Reproduced with permission from ref. 240, American Chemical Society, 2022. | |
Sensing of adamantane derivatives utilizing the ADA/CB[n] interactions was not achieved only through IDA assays243 but also by designing various sensors and by employing multidisciplinary analytical techniques and in continuation we will briefly mention a few related examples. A bioinspired smart nanochannel sensor based on competitive interactions for the sensitive and selective recognition of 1-aminoadamantane based on the ADA/CB[n] system was recently constructed.244 Specific host–guest interactions on the modified nanochannel led to the change of hydrophobicity and surface charge of the nanochannel that was observed as a change in ionic current, thus enabling high discrimination over other analytes and leading to sensitivity as high as 4.54 nM for 1-aminoadamantane recognition. Furthermore, a graphene and CB[7] electrode was developed for the differential pulse voltammetry (DPV) determination method of amantadine in biological fluids.245 Another example showcased that different CB[n] macrocycles with their specific portal-based host–guest interactions played a crucial role in promoting the formation of carbon dots (CDs) with a tunable particle size and tunable fluorescence properties.246 It was pointed out that these systems could be further employed as fluorescent biosensors for 1-aminoadamantane with a limit of detection up to a nM level (4.65 × 10−9 M) in a water environment.
We can conclude that implementation of the ADA/CB[n] system in combination with various analytical methods shows the advantages of using naturally occurring selective intermolecular interactions, which are happening on a molecular level, to obtain observable and measurable macroscopic responses that are of practical use.
3.6. ADA/CB[n] in sensing assays
Supramolecular systems are often employed in biosensing and diagnostic applications in medicinal and pharmaceutical fields. As we already described in the previous section, the ADA/CB[n] supramolecular system in particular has many desirable characteristics, like its reliable synthesis, chemical and thermodynamic stability, small size, compact packaging, etc. Furthermore, its binding affinity is exploited thoroughly and processes of association and dissociation can be effectively controlled. Especially important in terms of the ADA/CB[n] application in bioimaging and biosensing is its bio-orthogonality in binding to biomolecules. For example, Kim and coworkers designed an ultratight binding complex consisting of a modified CB[7] with a cyanine 3 donor dye (Cy3-CB[7]) and of 1-aminoadamantane conjugated with a cyanine 5 acceptor dye (Ad-Cy5) that exhibited fluorescence resonance energy transfer (FRET) upon complexation (Fig. 31).247 This complex type was used to study mechanisms of biological processes like SNARE (soluble N-ethylmaleimide-sensitive factor attachment protein receptor) mediated membrane fusion. More specifically, Cy3-CB[7] and Ad-Cy5 were encapsulated in ν- and t-vesicles, respectively, and when fusion occurred energy transfer from Cy3 to Cy5 could be observed due to the nearing of these fluorescent Cy3 and Cy5 moieties mediated through the encapsulation of the ADA moiety within the CB[7] cavity. With the help of fluorescent molecular probes, detection of pore opening and content release/mixing could be reliably done on a macroscopic level. The authors emphasized that this was the first successful sensing of flickering dynamics of a fusion pore by an in vitro assay using neuronal SNARE-reconstituted vesicles.247
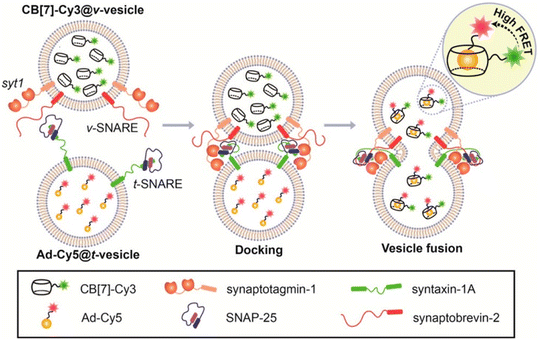 |
| Fig. 31 SNARE-mediated content mixing using a host–guest FRET pair: ν-vesicle containing Cy3-CB[7] and t-vesicle containing Ad-Cy5 undergo fusion and the resulting content mixing is detected by a FRET signal. Reproduced with permission from ref. 247, American Chemical Society, 2015. | |
In the next example, the same molecular probe FRET pair was helpful in visualization of intracellular processes such as autophagy in living cells.248 By using confocal laser scanning microscopy it was observed that Cy3-CB[7] and Ad-Cy5 crossed the membrane and accumulated in lysosomes and mitochondria, respectively (Fig. 32). Upon complexation of the ADA moiety into the CB[7] cavity a FRET signal emerged as a result of the fusion of lysosomes and mitochondria. In that way not only can the FRET signal event be observed but the host–guest system can also be exploited as a new tool for imaging cellular processes in living cells.
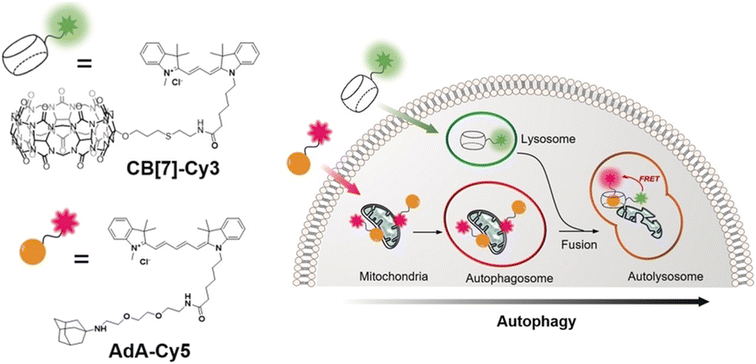 |
| Fig. 32 Emergence of FRET after the fusion of two different organelles (lysosome and mitochondria) through the process of autophagy. Reproduced with permission from ref. 248, Wiley VCH, 2018. | |
Very recently the same principle was applied for a rational design of supramolecular FRET pair consisting of Cy3-CB[7] and boron-dipyrromethene 630/650-adamantylammonium (BDP-AdA), with a purpose to visualize lipophagy.249 The authors showed how intracellular accumulations of Cy3-CB[7] in lysosomes and BDP-AdA in lipid droplets resulted in FRET signals upon formation of an inclusion complex between Cy3-CB[7] and BDP-AdA, thus enabling visualization of the lipid droplets fusion with lysosomes (lipophagy). Again the ADA/CB[7] system was utilized but now with a different fluorescent dye conjugated to the lipophilic ADA moiety, which served to demonstrate a diversity of substrates that could be built into molecular probes for various live imaging purposes.
This same supramolecular approach was also used for fluorescence imaging of proteins located in and on fixed or living cells.250 The proteins of interest were first chemically or biologically conjugated with ferrocene or ADA derivatives and afterwards Cy3-CB[7] was latched to proteins via intermolecular interactions acting during the process of inclusion of 1-aminoadamantyl (AdA) or ferrocenemethyl (FcA) moieties into CB[7] (Fig. 33). By applying fluorescence microscopy it was possible to obtain clear fluorescence images needed for accurate and precise analysis of protein locations. Control of the system was elegantly achieved by treatment with a stronger guest competitor for the CB[7] cavity. At low temperature this allows for a selective release of Cy3-CB[7] from AdA or FcA labeled proteins on the cell surface. The fluorescent signal of proteins on cellular surfaces vanishes whereas that for the internalized proteins remains. The authors showcased the advantages of using such a synthetic system, i.e., an ultrastable complex formed between Cy3-CB[7] and FcA or AdA conjugated proteins, when compared to the streptavidin-biotin (SA-BT) pair. The latter is a natural protein-based binding pair which is very sensitive in cellular environment and prone to enzymatic degradation.
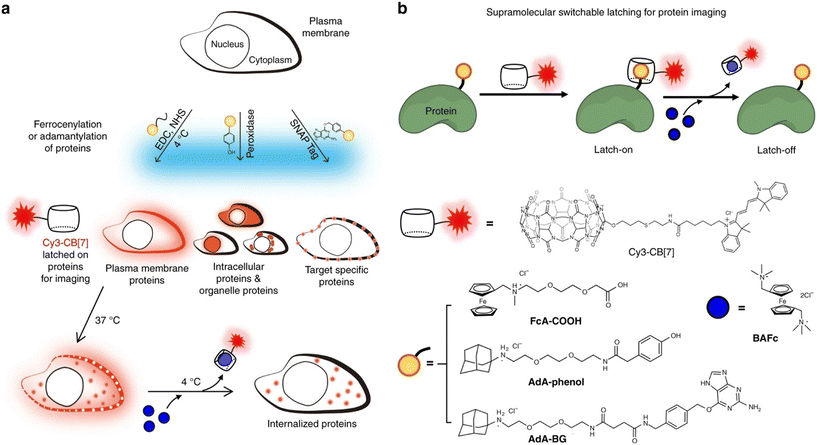 |
| Fig. 33 Universal and selective imaging of cellular proteins using a switchable supramolecular latching system (a) based on the interactions between Cy3-CB[7] and ADA derivatives (b). Reproduced with permission from ref. 250, Nature Research, 2018. | |
Kim's group even applied the same principle of non-covalent in situ anchoring of small synthetic molecules in a live organism (worm, Caenorhabditis elegans) by using a fully synthetic high-affinity binding pair consisting of Cy3-CB[7] and BODIPY BDP630/650-AdA (Fig. 34).251 What is more, it was also demonstrated that in vivo cancer imaging in a live mouse could be achieved using supramolecular latching of the synthetic system consisting of cyanine 5-AdA (Cy5-AdA) on a prelocalized CB[7]-conjugating antibody. This novel approach inspired many scientists to pursue synthetic host–guest pairs consisting of CB[n]s and ADA,252–254 eventually resulting in promising findings in the bioimaging field and providing new chemical tools for precise analysis of complicated mechanisms in biology.255
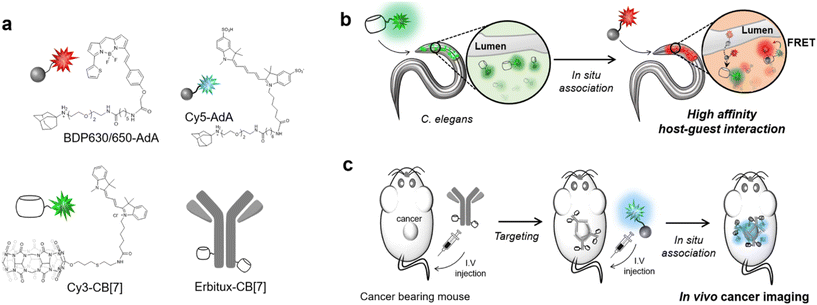 |
| Fig. 34 Locations of high-affinity host–guest interaction sites (a) in live C. elegans (b) and in vivo cancer imaging using the interaction between Erbitux-CB[7] and AdA-Cy5 that are sequentially injected to a cancer-bearing mouse through the tail vein (c). Reproduced with permission from ref. 251, American Chemical Society, 2019. | |
As mentioned earlier, macrocyclic compounds have also found application as antidotes for toxins since upon encapsulation of those harmful agents their extreme toxicity towards non-target organisms is significantly reduced, especially towards animals and humans.146,152,153 An illustrative example to mention here is a highly fluorescent rhodamine-based probe for detection of herbicide paraquat (PQ) that was improved by utilizing the ADA/CB[7] system which had a stronger fluorescence intensity than rhodamine B itself.256 This feature could be attributed to the host–guest interaction which induced a spirocyclic ring-opening process and a suppression of host molecule aggregation. The complex in question displayed high sensitivity toward PQ, with an excellent nanomolar limit of detection (10 nM) and the authors pointed out that it could serve as a highly selective and sensitive probe for detecting PQ in various biological applications as well as in real life samples. It was concluded that this unique detection mechanism involved a process of self-assembly, disassembly and reassembly, which could be regenerated by the addition of host CB[7], thus providing a reversible fluorescence response to the analyte herbicide PQ (Fig. 35).
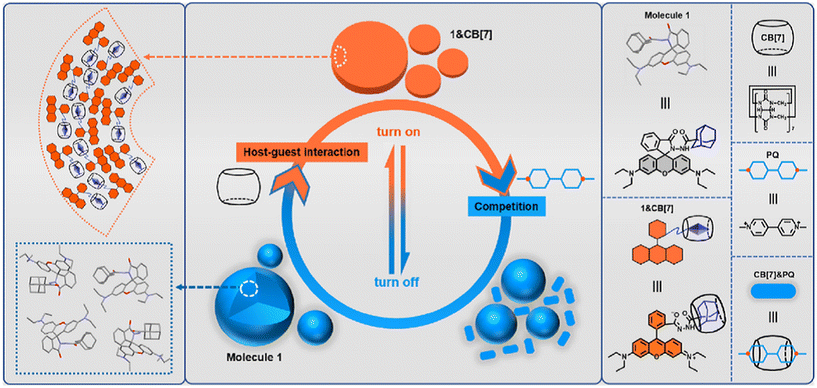 |
| Fig. 35 Illustration of complex formation and herbicide (paraquat, PQ) detection. Reproduced with permission from ref. 256, Elsevier, 2023. | |
On another note, in 2018 preliminary results dealing with the suitability of the ADA/CB[7] system in pre-targeted positron emission tomography (PET) imaging were presented (Fig. 36).257 The idea was that the CB[n]-conjugated antibodies could be visualized in the future by various radiolabeled ADA derivatives. This early proof-of-concept work shows that the ADA/CB[7] system itself (without antibody conjugation) is capable of remarkably fast complex formation and clearance in vivo and indeed has the potential for possible application in pre-targeted PET imaging procedures. This research direction was very recently further expanded on with the development of three 64Cu-labeled adamantane-containing guests/radioligands that were demonstrated to have high in vitro stability in combination with both specific and high tumor uptake.258
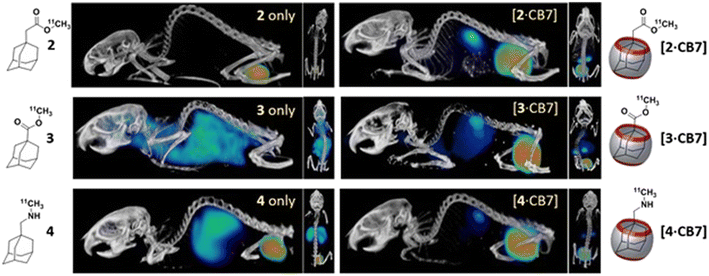 |
| Fig. 36 Immuno-PET imaging showing biodistribution of labeled ADA molecules in mice in the absence (left column) and presence (right column) of CB[7]. Reproduced with permission from ref. 257, Sage Publications, 2018. | |
A different example of the ADA/CB[n] use in bioimaging is a recently reported supramolecular assembly that was based on a purely organic light-harvesting phosphorescence energy transfer (PET) in an aqueous solution.259 It consisted of a pyridine modified β-cyclodextrin (CD-PY) as a donor, CB[8] as a mediator, rhodamine B (RhB) as an acceptor, and hyaluronic acid modified with the adamantane subunit (HA-ADA) as a cancer cell targeting agent (Fig. 37). In the first step pseudorotaxane is formed with CB[8] and CD-PY and the phosphorescence of CD-PY is “turned on” with a green phosphorescence emission at 510 nm. Afterwards, when RhB is encapsulated into the β-CD cavity, efficient solution-state light-harvesting PET occurs between pseudorotaxane and RhB, providing high energy transfer efficiency and an ultrahigh antenna effect between pseudorotaxane and RhB. However, the most important step is when HA-ADA assembles to form nanoparticles as it both promotes the delayed emission at 590 nm and successfully targets the mitochondria of A549 cancer cells.
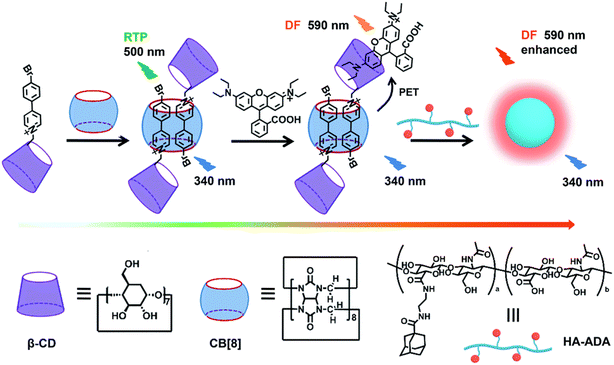 |
| Fig. 37 Construction of the supramolecular assembly for a purely organic light-harvesting PET system and related molecules. Reproduced with permission from ref. 259, the Royal Society of Chemistry, 2020. | |
3.7. Interdisciplinary applications and perspectives
Remarkably, in just 30 years since the exact structure of CB[n]s has been confirmed these macrocycles have been extensively studied in various systems of diverse biological applications. Simple host–guest systems, due to their promising features like bioavailability and non-toxicity, have the opportunity to dominate over other macrocycles in the fields of drug delivery and controlled drug release, with a possibility to further extend their sphere of influence to the fields of biosensing and diagnostic uses in medicine and pharmaceutical research. Additionally, CB[n] macrocycles in the role of host molecules80 could be implemented with other systems to build heterogeneous nano-assemblies, also with promising potential biological applications like drug and gene delivery, bioimaging, and photodynamic cancer therapy.131,137,171,260–270 In such hybrid systems CB[n]s are typically carrier vehicles for biologically active compounds or are functional parts of the emerging nanostructure and are therefore involved in controlling drug release mechanism from the nanomaterial. Up to now cucurbit[n]urils have successfully been combined with other nanomaterials271 like polymers,272–276 proteins,131–133 (e.g., bovine serum albumin), metal nanoparticles,277–281etc. Even in these elaborate supramolecular assemblies the ADA/CB[n] system found its use to some extent, mostly for displacement purposes, and is applied either in compilation with heterogenic organic hybrids such as proteins250 or with organic–inorganic hybrids such as mesoporous silica nanoparticles (MSNPs),282 gold nanoparticles (AuNPs),283,284 zinc-doped iron-oxide nanoparticles,285–287etc. It should also be mentioned that inorganic nanoparticles usually have fascinating photo, thermal and magnetic properties or structural characteristics like porous morphology suitable for cargo loading, making them currently the leading research objectives in the field of drug delivery and bioimaging.
Host–guest interactions between functionalized ADA and CB[7] were also utilized in smart design of platelet-mimicking supramolecular nanomedicine (SN) for cascade amplification of synergistic chemotherapy. The applied supramolecular method proved to be superior because it allowed for exact control of the ratio of camptothecin (CPT) to cisplatin (Pt), two drugs which often could not be precisely regulated in different delivery systems.288 Among cucurbit[n]uril-based amphiphiles289,290 that self-assemble into nanomaterials as functional supramolecular micelles (FMS), many examples of ADA/CB[n] system could be employed for perspective biological and technological application,291 more specifically, for cell-targeted drug delivery292 or even as multifunctional theranostic agents for synergistic photodynamic therapy and hypoxia-activated chemotherapy.293 In these examples FSMs exhibited favorable characteristics such as high bioavailability, stability, precise targeting, and excellent delivery efficiency, making them highly promising for use in the drug delivery domain. In addition, high-affinity ADA/CB[n] interaction between functionalized DNA strands could direct hierarchical assembly of origami monomers into DNA nanofibers of micrometer-length.294
As another fascinating example of bioapplicable nanomaterial important for understanding and diagnosing various diseases, Wang and coworkers reported supramolecular luminol–AIEgen nanoparticles (SLA NP) used for bioimaging of deep-tissue-inflammation. The supramolecular system was constructed with ADA and CB[7] functionalized with luminol and AIEgen, respectively, using the resulting host–guest interactions to produce efficient bioluminescence resonance energy transfer (BRET) from blue-emissive luminol to red-emissive AIEgen, thus providing fluorescent imaging.266
In the end, we will present one recent example of AuNPs combined with a different host–guest ADA/CB[n] supramolecular system that was developed for quantitative detection of SARS-CoV-2 antibodies in human serum.295 The sensing principle involves several steps (Fig. 37) and the highly specific response for SARS-CoV-2 antibodies is based on two characteristic signals obtained by translocations of ADA/CB[6]-modified probe DNAs through α-hemolysin (α-HL) (step 5 on Fig. 38). The signals in question differ from signals of other biomolecules and act as surrogates instead of directly measuring IgG or IgM antibodies. The authors claim that such DNA-assisted nanopore biosensor with two unique oscillating patterns in the signal can reliably quantify SARS-CoV-2 antibodies with high accuracy, large dynamic range, and potential for assay automation, despite the assay protocol consisting of multiple manual steps and long incubation times.295
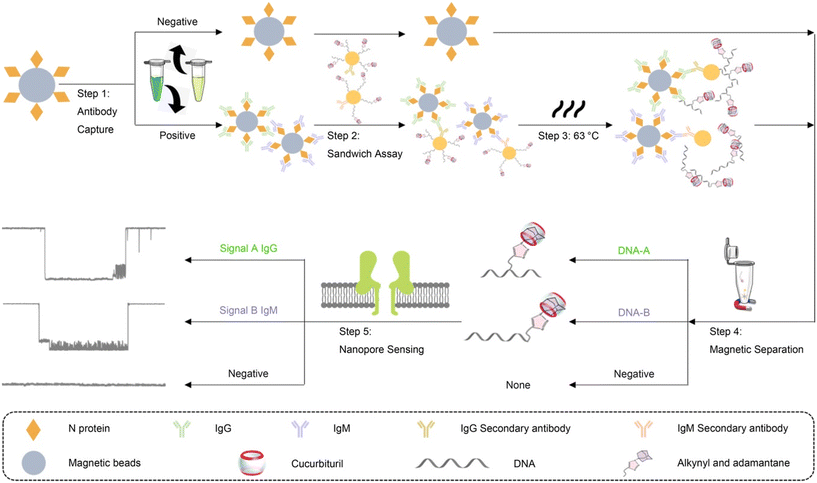 |
| Fig. 38 SARS-CoV-2 antibodies sensing: (1) capture of IgG and IgM antibodies with N-protein functionalized magnetic beads (MBs); (2) formation of the sandwich structure between IgG or IgM antibodies and probe DNA modified AuNPs; (3) thermal dehybridization of the probe DNAs from the AuNPs; (4) magnetic separation of probe DNAs from the sandwich structures; and (5) quantification of probe DNAs using the nanopore sensor. Reproduced with permission from ref. 295, Elsevier, 2021. | |
4. Conclusion and outlook
Interest of medicinal chemists for the adamantane scaffold has recently expanded to the supramolecular ADA/CB[n] system. Such attention to adamantane derivatives as components of complex drug delivery systems stems from the fact that the adamantyl cage almost ideally occupies the corresponding CB[n] cavity, enabling this supramolecular system to be regarded by many as a golden standard in the field of host–guest mediated drug delivery. Through the selected recent examples we showcased how the ADA/CB[n] not only affects the bioactivity and bioavailability of drug molecules but can also tune drug properties. However, the applicability of this system goes beyond even that; implementation could be achieved also in the fields of practical bioapplication, bioanalytics, sensing assays, bioimaging, etc.
A good fit between the hydrophobic ADA and the CB[n] host cavity enables a strong and selective binding and affords stable inclusion complexes, which in turn opens up new venues for drug delivery. Namely, the emerging synergistic properties of such complexes in live cells give rise to exciting new pharmacological tools and offer an advantage over traditional single drug molecule approach. For example, host–guest non-covalent interactions are a useful and often affordable way to overcome existing physicochemical barriers hindering medicinal application of some drug candidates since supramolecular effects can facilitate the change of intrinsic properties of the drug (guest), like heightening its solubility, improving its chemical and thermal stability and metabolic resistivity by encapsulation, etc. The result is a focused delivery to a biological target and efficient drug action upon release, while at the same time reducing the amount of the active compound needed for a successful treatment. Non-covalent interactions responsible for guest encapsulation essentially enable active compound shielding and steric protection against metabolic processes like oxidation, hydrolysis and various nucleophilic attacks during the in vivo transport, thereby increasing the overall drug bioavailability. Furthermore, the host's macrocyclic cavity mimics the function of the binding region of a biological receptor with its pre-organized shape and by engaging in complexation with the guest the resulting ADA/CB[n] system becomes essentially a biomimetic entity. Here we should mention that one significant drawback to using CB[n] hosts as drug carriers or in other bioapplications that was identified is their somewhat poor water solubility. However, that could be successfully circumvented by chemical functionalization methods, by adding to the formulations biocompatible salts, or by changing the pH. Complexation induced shifting of the pKa values of the guest molecules and their subsequent water solubility increase is especially useful for compounds that are readily protonated by nature. Additionally, the synergistic effect upon complexation is not limited solely to guest (drug) molecules; by inclusion complex formation the solubility of the supramolecular ADA/CB[n] system as a whole can often be increased to levels acceptable for practical purposes. Note that despite a higher binding preference of ADA towards CB[n] when compared to cyclodextrin hosts, drug release from the CB[n] cavity can still be effectively achieved by using thermodynamic means since complex formation is a dynamic process governed by non-covalent interactions. A method commonly used for drug release from reversible supramolecular systems is introduction of a second molecule with higher affinity for the host, aptly termed guest displacement. However, one future challenge of this field is to develop new ADA/CB[n] variations capable of achieving a good balance between stable encapsulation and efficient release at biological target sites. Supramolecular chemotherapy, encapsulation of inherently toxic bioactive compounds in order to decrease their cytotoxicity to healthy cells, is also a principle readily used for integrating non-covalent interactions in targeted drug therapy.
Applications of ADA/CB[n] in chemosensing in physiologically relevant biological systems have also been on the rise in recent years but there still remain many challenges to be overcome due to very low analyte concentrations as well as competitive substrate interferences. Bioanalytical and diagnostic applications are also of interest and significant headways have been made in these fields as well since the ADA/CB[n] system is capable of high selectivity and strong binding which then, with careful design, translates to specific interactions and bio-orthogonality in binding. For example, application of CB[n]-based molecular sensors as indicator displacement assays for ADA derivatives detection in a biological environment (e.g., in urine, human serum, blood serum, biofluids, etc.) has gained significant attention since the technique can provide an accurate and specific observable response, unlike direct binding assays.
Future implementation of the ADA/CB[n] in the fields of drug delivery and controlled drug release depends heavily on the design of bioactive guest molecules with an ADA scaffold as a structural feature responsible for enabling the binding event. Intracellular recognition and subsequent action of the bioactive molecule is ultimately the purpose of the supramolecular system where the CB[n] host acts as a vehicle. In areas like biosensing and other diagnostic uses in medicine and pharmaceutical research the challenge remains to identify suitable in vivo targets and then develop the supramolecular complex which utilizes the ADA–CB[n] interaction and which is capable of providing a specific, accurate and reliable response.
Other exciting areas where ADA/CB[n] shows a potential for future bioapplication are in hybrid heterogeneous nano-assemblies for drug and gene delivery, bioimaging, photodynamic cancer therapy, etc. In such elaborate supramolecular assemblies the ADA/CB[n] may be used for displacements purposes, as a cargo vehicle and beyond. Taking into account all the current applications of the ADA/CB[n] system as well as the possibilities currently in development, one cannot but conclude that the future looks supramolecular!
Author contributions
The manuscript was written through contributions of both authors. Both authors have given approval to the final version of the manuscript.
Conflicts of interest
The authors declare no competing financial interest.
Acknowledgements
These materials are based on work financed by the Croatian Science Foundation (HRZZ, UIP-2017-05-9653). The authors thank Dr. Ruža Frkanec for fruitful discussions and Vesna Uglješić, M. A. for designing and creating the table of contents image.
References
- S. Landa and V. Macháček, Sur l'adamantane, nouvel hydrocarbure extrait du naphte, Collect. Czech. Chem. Commun., 1933, 5, 1–5 CrossRef CAS.
- V. Prelog and R. Seiwerth, Über die Synthese des Adamantans, Ber. Dtsch. Chem. Ges., 1941, 74, 1644–1648 CrossRef.
- V. Prelog and R. Seiwerth, Über eine neue, ergiebigere Darstellung des Adamantans, Ber. Dtsch. Chem. Ges., 1941, 74, 1769–1772 CrossRef.
- P. v. R. Schleyer, A Simple Preparation of Adamantane, J. Am. Chem. Soc., 1957, 79, 3292 CrossRef CAS.
- P. v. R. Schleyer, M. M. Donaldson, R. D. Nicholas and C. Cupas, Adamantane, Org. Synth., 1962, 42, 8 CrossRef CAS.
- R. C. Fort and P. v. R. Schleyer, Adamantane: Consequences of the Diamondoid Structure, Chem. Rev., 1964, 64, 277–300 CrossRef CAS.
-
R. C. Fort Jr., Adamantane – the chemistry of diamond molecules, Marcel Dekker, Inc., New York, 1976 Search PubMed.
- C. J. Van der Schyf and W. J. Geldenhuys, Polycyclic compounds: Ideal drug scaffolds for the design of multiple mechanism drugs?, Neurotherapeutics, 2009, 6, 175–186 CrossRef CAS PubMed.
- G. Lamoureux and G. Artavia, Use of the Adamantane Structure in Medicinal Chemistry, Curr. Med. Chem., 2010, 17, 2967–2978 CrossRef CAS PubMed.
- J. Liu, D. Obando, V. Liao, T. Lifa and R. Codd, The many faces of the adamantyl group in drug design, Eur. J. Med. Chem., 2011, 46, 1949–1963 CrossRef CAS PubMed.
- L. Wanka, K. Iqbal and P. R. Schreiner, The Lipophilic Bullet Hits the Targets: Medicinal Chemistry of Adamantane Derivatives, Chem. Rev., 2013, 113, 3516–3604 CrossRef CAS PubMed.
- H. Schwertfeger, A. A. Fokin and P. R. Schreiner, Diamonds are a Chemist's Best Friend: Diamondoid Chemistry Beyond Adamantane, Angew. Chem., Int. Ed., 2008, 47, 1022–1036 CrossRef CAS PubMed.
- M. A. Gunawan, J.-C. Hierso, D. Poinsot, A. A. Fokin, N. A. Fokina, B. A. Tkachenko and P. R. Schreiner, Diamondoids: functionalization and subsequent applications of perfectly defined molecular cage hydrocarbons, New J. Chem., 2014, 38, 28–41 RSC.
- J. E. Dahl, S. G. Liu and R. M. K. Carlson, Isolation and Structure of Higher Diamondoids, Nanometer-Sized Diamond Molecules, Science, 2003, 299, 96–99 CrossRef CAS PubMed.
- I. K. Moiseev, N. V. Makarova and M. N. Zemtsova, Reactions of adamantanes in electrophilic media, Russ. Chem. Rev., 1999, 68, 1001–1020 CrossRef CAS.
- W. L. Davies, R. R. Grunert, R. F. Haff, J. W. McGahen, E. M. Neumayer, M. Paulshock, J. C. Watts, T. R. Wood, E. C. Hermann and C. E. Hoffmann, Antiviral Activity of 1-Adamantanamine (Amantadine), Science, 1964, 144, 862–863 CrossRef CAS PubMed.
- E. De Clercq, Antiviral agents active against influenza A viruses, Nat. Rev. Drug Discovery, 2006, 5, 1015–1025 CrossRef CAS PubMed.
- C. Wang, K. Takeuchi, L. H. Pinto and R. A. Lamb, Ion channel activity of influenza A virus M2 protein: characterization of the amantadine block, J. Virol., 1993, 67, 5585–5594 CrossRef CAS PubMed.
- V. L. Raghavendra Rao, A. Dogan, K. G. Todd, K. K. Bowen and R. J. Dempsey, Neuroprotection by memantine, a non-competitive NMDA receptor antagonist after traumatic brain injury in rats, Brain Res., 2001, 911, 96–100 CrossRef CAS PubMed.
- M. Šekutor, K. Mlinarić-Majerski, T. Hrenar, S. Tomić and I. Primožič, Adamantane-substituted guanylhydrazones: novel inhibitors of butyrylcholinesterase, Bioorg. Chem., 2012, 41–42, 28–34 CrossRef PubMed.
- M. Šekutor, A Multidisciplinary Approach to the Study of Adamantyl Aminoguanidines – A New Class of Potentially Bioactive Compounds, Synlett, 2015, 26, 2627–2632 CrossRef.
- V. Pardali, E. Giannakopoulou, D.-I. Balourdas, V. Myrianthopoulos, C. M. Taylor, M. Šekutor, K. Mlinarić-Majerski, M. J. Kelly and G. Zoidis, Lipophilic Guanylhydrazone Analogues as Promising Trypanocidal Agents: An Extended SAR Study, Curr. Pharm. Des., 2020, 26, 838–866 CrossRef CAS PubMed.
- M. Šekutor, A. Štimac, K. Mlinarić-Majerski and R. Frkanec, Syntheses and characterization of liposome-incorporated adamantyl aminoguanidines, Org. Biomol. Chem., 2014, 12, 6005–6013 RSC.
- A. Štimac, M. Tokić, A. Ljubetič, T. Vuletić, M. Šekutor, J. Požar, K. Leko, M. Hanževački, L. Frkanec and R. Frkanec, Functional self-assembled nanovesicles based on β-cyclodextrin, liposomes and adamantyl guanidines as potential nonviral gene delivery vectors, Org. Biomol. Chem., 2019, 17, 4640–4651 RSC.
- A. Štimac, M. Šekutor, K. Mlinarić-Majerski, L. Frkanec and R. Frkanec, Adamantane in Drug Delivery Systems and Surface Recognition, Molecules, 2017, 22, 297 CrossRef PubMed.
- E. Persch, O. Dumele and F. Diederich, Molecular Recognition in Chemical and Biological Systems, Angew. Chem., Int. Ed., 2015, 54, 3290–3327 CrossRef CAS PubMed.
- A. P. Marchand, K. A. Kumar, A. S. McKim, K. Mlinarić-Majerski and G. Kragol, Synthesis and alkali metal picrate extraction capabilities of novel cage-functionalized 17-crown-5 and 17-crown-6 ethers, Tetrahedron, 1997, 53, 3467–3474 CrossRef CAS.
- A. P. Marchand, S. Alihodžić, A. S. McKim, K. A. Kumar, K. Mlinarić-Majerski, T. Šumanovac and S. G. Bott, Synthesis and alkali metal picrate extraction capabilities of a 4-oxahexacyclo[5.4.1.02,6.03,10.05,9.08,11]dodecane-derived cryptand. A new ionophore for selective ion complexation, Tetrahedron Lett., 1998, 39, 1861–1864 CrossRef CAS.
- T. Hayashita, T. Higuchi, H. Sawano, A. P. Marchand, K. A. Kumar, S. G. Bott, K. Mlinarić-Majerski, T. Šumanovac, N. S. A. Elkarim, H.-S. Hwang, G. G. Talanova and R. A. Bartsch, Molecular design of lipophilic disalicylic acid compounds with varying spacers for selective lead(II) extraction, Talanta, 2000, 52, 385–396 CrossRef CAS PubMed.
- K. Mlinarić-Majerski and G. Kragol, Design, synthesis and cation-binding properties of novel adamantane- and 2-oxaadamantane-containing crown ethers, Tetrahedron, 2001, 57, 449–457 CrossRef.
- K. Mlinarić-Majerski and T. Šumanovac Ramljak, Synthesis and alkali metal binding properties of novel N-adamantylaza-crown ethers, Tetrahedron, 2002, 58, 4893–4898 CrossRef.
- A. P. Marchand, A. Hazlewood, Z. Huang, S. K. Vadlakonda, J.-D. R. Rocha, T. D. Power, K. Mlinarić-Majerski, L. Klaić, G. Kragol and J. C. Bryan, Stabilization of a K+-(bis-Cage-Annulated 20-Crown-6) Complex by Bidentate Picrate, Struct. Chem., 2003, 14, 279–288 CrossRef CAS.
- I. Vujasinović, J. Veljković, K. Molčanov, B. Kojić-Prodić and K. Mlinarić-Majerski, Thiamacrocyclic Lactones: New Ag(I)-Ionophores, J. Org. Chem., 2008, 73, 9221–9227 CrossRef PubMed.
- T. Šumanovac Ramljak, K. Mlinarić-Majerski and B. Bertoša, Alkali metal ion complexation of adamantane functionalized diaza-bibracchial lariat ethers, Croat. Chem. Acta, 2012, 85, 559–568 CrossRef.
- M. Renić, N. Basarić and K. Mlinarić-Majerski, Adamantane–dipyrromethanes: novel anion receptors, Tetrahedron Lett., 2007, 48, 7873–7877 CrossRef.
- M. Alešković, I. Halasz, N. Basarić and K. Mlinarić-Majerski, Synthesis, structural characterization, and anion binding ability of sterically congested adamantane-calix[4]pyrroles and adamantane-calixphyrins, Tetrahedron, 2009, 65, 2051–2058 CrossRef.
- M. Alešković, N. Basarić, K. Mlinarić-Majerski, K. Molčanov, B. Kojić-Prodić, M. K. Kesharwani and B. Ganguly, Anion recognition through hydrogen bonding by adamantane-dipyrromethane receptors, Tetrahedron, 2010, 66, 1689–1698 CrossRef.
- V. Blažek, N. Bregović, K. Mlinarić-Majerski and N. Basarić, Phosphate selective alkylenebisurea receptors: structure-binding relationship, Tetrahedron, 2011, 67, 3846–3857 CrossRef.
- M. Alešković, N. Basarić, I. Halasz, X. Liang, W. Qin and K. Mlinarić-Majerski, Aryl substituted adamantane–dipyrromethanes: chromogenic and fluorescent anion sensors, Tetrahedron, 2013, 69, 1725–1734 CrossRef.
- V. Blažek, K. Molčanov, K. Mlinarić-Majerski, B. Kojić-Prodić and N. Basarić, Adamantane bisurea derivatives: anion binding in the solution and in the solid state, Tetrahedron, 2013, 69, 517–526 CrossRef.
- M. Alešković, N. Basarić, N. Došlić, V. Tomišić and K. Mlinarić-Majerski, HSO4− sensing based on proton transfer in H-bonding complexes, Supramol. Chem., 2014, 26, 850–855 CrossRef.
- M. Šekutor and K. Mlinarić-Majerski, Adamantyl aminoguanidines as receptors for oxo-anions, Tetrahedron Lett., 2014, 55, 6665–6670 CrossRef.
- V. Blažek Bregović, I. Halasz, N. Basarić and K. Mlinarić-Majerski, Anthracene adamantylbisurea receptors: switching of anion binding by photocyclization, Tetrahedron, 2015, 71, 9321–9327 CrossRef.
- M. Alešković, M. Glavaš, M. Šekutor and K. Mlinarić-Majerski, Synthesis and Anion Binding Assessment of Novel Adamantane Amidopyrroles, Croat. Chem. Acta, 2017, 90, 637–643 CrossRef.
- L. Cao, M. Šekutor, P. Y. Zavalij, K. Mlinarić-Majerski, R. Glaser and L. Isaacs, Cucurbit[7]uril·Guest Pair with an Attomolar Dissociation Constant, Angew. Chem., Int. Ed., 2014, 53, 988–993 CrossRef CAS PubMed.
- M. Šekutor, K. Molčanov, L. Cao, L. Isaacs, R. Glaser and K. Mlinarić-Majerski, Design, Synthesis, and X-ray Structural Analyses of Diamantane Diammonium Salts: Guests for Cucurbit[n]uril (CB[n]) Hosts, Eur. J. Org. Chem., 2014, 2014, 2533–2542 CrossRef.
- J. Hostaš, D. Sigwalt, M. Šekutor, H. Ajani, M. Dubecký, J. Řezáč, P. Y. Zavalij, L. Cao, C. Wohlschlager, K. Mlinarić-Majerski, L. Isaacs, R. Glaser and P. Hobza, A Nexus between Theory and Experiment: Non-Empirical Quantum Mechanical Computational Methodology Applied to Cucurbit[n]urilGuest Binding Interactions, Chem. – Eur. J., 2016, 22, 17226–17238 CrossRef PubMed.
- D. Sigwalt, M. Šekutor, L. Cao, P. Y. Zavalij, J. Hostaš, H. Ajani, P. Hobza, K. Mlinarić-Majerski, R. Glaser and L. Isaacs, Unraveling the Structure–Affinity Relationship between Cucurbit[n]urils (n = 7, 8) and Cationic Diamondoids, J. Am. Chem. Soc., 2017, 139, 3249–3258 CrossRef CAS PubMed.
- T. Šumanovac, M. Alešković, M. Šekutor, M. Matković, T. Baron, K. Mlinarić-Majerski, C. Bohne and N. Basarić, Photoelimination of nitrogen from adamantane and pentacycloundecane (PCU) diazirines: a spectroscopic study and supramolecular control, Photochem. Photobiol. Sci., 2019, 18, 1806–1822 CrossRef PubMed.
- M. Alešković, S. Roca, R. Jozepović, N. Bregović and M. Šekutor, Unravelling binding effects in cyclodextrin inclusion complexes with diamondoid ammonium salt guests, New J. Chem., 2022, 46, 13406–13414 RSC.
- D. King, T. Šumanovac, S. Murkli, P. R. Schreiner, M. Šekutor and L. Isaacs, Cucurbit[8]uril forms tight inclusion complexes with cationic triamantanes, New J. Chem., 2023, 47, 5338–5346 RSC.
- M. V. Rekharsky and Y. Inoue, Complexation Thermodynamics of Cyclodextrins, Chem. Rev., 1998, 98, 1875–1918 CrossRef CAS PubMed.
- D. Harries, D. C. Rau and V. A. Parsegian, Solutes Probe Hydration in Specific Association of Cyclodextrin and Adamantane, J. Am. Chem. Soc., 2005, 127, 2184–2190 CrossRef CAS PubMed.
- J. Carrazana, A. Jover, F. Meijide, V. H. Soto and J. Vázquez Tato, Complexation of Adamantyl Compounds by β-Cyclodextrin and Monoaminoderivatives, J. Phys. Chem. B, 2005, 109, 9719–9726 CrossRef CAS PubMed.
- J. Voskuhl, M. Waller, S. Bandaru, B. A. Tkachenko, C. Fregonese, B. Wibbeling, P. R. Schreiner and B. J. Ravoo, Nanodiamonds in sugar rings: an experimental and theoretical investigation of cyclodextrin–nanodiamond inclusion complexes, Org. Biomol. Chem., 2012, 10, 4524–4530 RSC.
- F. Schibilla, J. Voskuhl, N. A. Fokina, J. E. P. Dahl, P. R. Schreiner and B. J. Ravoo, Host–Guest Complexes of Cyclodextrins and Nanodiamonds as a Strong Non-Covalent Binding Motif for Self-Assembled Nanomaterials, Chem. – Eur. J., 2017, 23, 16059–16065 CrossRef CAS PubMed.
- K. Leko, M. Hanževački, Z. Brkljača, K. Pičuljan, R. Ribić and J. Požar, Solvophobically Driven Complexation of Adamantyl Mannoside with β-Cyclodextrin in Water and Structured Organic Solvents, Chem. – Eur. J., 2020, 26, 5208–5219 CrossRef CAS PubMed.
-
J. Szejtli and T. Osa, Comprehensive cyclodextrin chemistry, Pergamon, Oxford, UK, 1996, vol. 3 Search PubMed.
- J. Li and X. J. Loh, Cyclodextrin-based supramolecular architectures: Syntheses, structures, and applications for drug and gene delivery, Adv. Drug Delivery Rev., 2008, 60, 1000–1017 CrossRef CAS PubMed.
- R. Behrend, E. Meyer and F. Rusche, Ueber Condensationsproducte aus Glycoluril und Formaldehyd, Justus Liebigs Ann. Chem., 1905, 339, 1–37 CrossRef.
- W. A. Freeman, W. L. Mock and N. Y. Shih, Cucurbituril, J. Am. Chem. Soc., 1981, 103, 7367–7368 CrossRef CAS.
- J. Kim, I.-S. Jung, S.-Y. Kim, E. Lee, J.-K. Kang, S. Sakamoto, K. Yamaguchi and K. Kim, New Cucurbituril Homologues: Syntheses, Isolation, Characterization, and X-ray Crystal Structures of Cucurbit[n]uril (n = 5, 7, and 8), J. Am. Chem. Soc., 2000, 122, 540–541 CrossRef CAS.
- K. I. Assaf and W. M. Nau, Cucurbiturils: from synthesis to high-affinity binding and catalysis, Chem. Soc. Rev., 2015, 44, 394–418 RSC.
- A. Day, A. P. Arnold, R. J. Blanch and B. Snushall, Controlling Factors in the Synthesis of Cucurbituril and Its Homologues, J. Org. Chem., 2001, 66, 8094–8100 CrossRef CAS PubMed.
-
W. H. Huang, S. Liu and L. Isaacs, in Modern Supramolecular Chemistry: Strategies for Macrocycle Synthesis, ed. F. Diederich, P. J. Stang and R. R. Tykwinski, Wiley-VCH, Weinheim, 2008, p. 113 Search PubMed.
- R.-L. Lin, J.-X. Liu, K. Chen and C. Redshaw, Supramolecular chemistry of substituted cucurbit[n]urils, Inorg. Chem. Front., 2020, 7, 3217–3246 RSC.
- K. Kim, N. Selvapalam, Y. H. Ko, K. M. Park, D. Kim and J. Kim, Functionalized cucurbiturils and their applications, Chem. Soc. Rev., 2007, 36, 267–279 RSC.
- N. N. Andersen, M. Lisbjerg, K. Eriksen and M. Pittelkow, Hemicucurbit[n]urils and Their Derivatives - Synthesis and Applications, Isr. J. Chem., 2018, 58, 435–448 CrossRef CAS.
- X.-J. Cheng, L.-L. Liang, K. Chen, N.-N. Ji, X. Xiao, J.-X. Zhang, Y.-Q. Zhang, S.-F. Xue, Q.-J. Zhu, X.-L. Ni and Z. Tao, Twisted Cucurbit[14]uril, Angew. Chem., Int. Ed., 2013, 52, 7252–7255 CrossRef CAS PubMed.
- Q. Li, S.-C. Qiu, J. Zhang, K. Chen, Y. Huang, X. Xiao, Y. Zhang, F. Li, Y.-Q. Zhang, S.-F. Xue, Q.-J. Zhu, Z. Tao, L. F. Lindoy and G. Wei, Twisted Cucurbit[n]urils, Org. Lett., 2016, 18, 4020–4023 CrossRef CAS PubMed.
- L. Isaacs, S.-K. Park, S. Liu, Y. H. Ko, N. Selvapalam, Y. Kim, H. Kim, P. Y. Zavalij, G.-H. Kim, H.-S. Lee and K. Kim, The Inverted Cucurbit[n]uril Family, J. Am. Chem. Soc., 2005, 127, 18000–18001 CrossRef CAS PubMed.
- M. Stancl, J. Svec and V. Sindelar, Novel Supramolecular Hosts Based on Linear and Cyclic Oligomers of Glycoluril, Isr. J. Chem., 2011, 51, 592–599 CrossRef CAS.
-
W. L. Mock, in Supramolecular Chemistry II — Host Design and Molecular Recognition, ed. E. Weber, Springer, Berlin Heidelberg, 1995, vol. 175, pp. 1–24 Search PubMed.
- J. W. Lee, S. Samal, N. Selvapalam, H.-J. Kim and K. Kim, Cucurbituril Homologues and Derivatives: New Opportunities in Supramolecular Chemistry, Acc. Chem. Res., 2003, 36, 621–630 CrossRef CAS PubMed.
- L. Isaacs, Cucurbit[n]urils: from mechanism to structure and function, Chem. Commun., 2009, 619–629 RSC.
- J. Lagona, P. Mukhopadhyay, S. Chakrabarti and L. Isaacs, The Cucurbit[n]uril Family, Angew. Chem., Int. Ed., 2005, 44, 4844–4870 CrossRef CAS PubMed.
- W. M. Nau and O. A. Scherman, The World of Cucurbiturils - From Peculiarity to Commodity, Isr. J. Chem., 2011, 51, 492–494 CrossRef CAS.
- E. Masson, X. Ling, R. Joseph, L. Kyeremeh-Mensah and X. Lu, Cucurbituril chemistry: a tale of supramolecular success, RSC Adv., 2012, 2, 1213–1247 RSC.
- L. Isaacs, Stimuli responsive systems constructed using cucurbit[n]uril-type molecular containers, Acc. Chem. Res., 2014, 47, 2052–2062 CrossRef CAS PubMed.
- S. J. Barrow, S. Kasera, M. J. Rowland, J. del Barrio and O. A. Scherman, Cucurbituril-Based Molecular Recognition, Chem. Rev., 2015, 115, 12320–12406 CrossRef CAS PubMed.
- A. I. Day, R. J. Blanch, A. P. Arnold, S. Lorenzo, G. R. Lewis and I. Dance, A Cucurbituril-Based Gyroscane: A New Supramolecular Form, Angew. Chem., 2002, 114, 285–287 CrossRef.
- S. Liu, P. Y. Zavalij and L. Isaacs, Cucurbit[10]uril, J. Am. Chem. Soc., 2005, 127, 16798–16799 CrossRef CAS PubMed.
- M. Dračínský, C. S. Hurtado, E. Masson and J. Kaleta, Stuffed pumpkins: mechanochemical synthesis of host–guest complexes with cucurbit[7]uril, Chem. Commun., 2021, 57, 2132–2135 RSC.
- W. M. Nau, M. Florea and K. I. Assaf, Deep Inside Cucurbiturils: Physical Properties and Volumes of their Inner Cavity Determine the Hydrophobic Driving Force for Host-Guest Complexation, Isr. J. Chem., 2011, 51, 559–577 CrossRef CAS.
- P. Germain, J. M. Létoffé, M. P. Merlin and H. J. Buschmann, Thermal behaviour of hydrated and anhydrous Cucurbituril, Thermochim. Acta, 1998, 315, 87–92 CrossRef CAS.
- C. Márquez, R. R. Hudgins and W. M. Nau, Mechanism of
Host−Guest Complexation by Cucurbituril, J. Am. Chem. Soc., 2004, 126, 5806–5816 CrossRef PubMed.
- F. Biedermann and H.-J. Schneider, Experimental Binding Energies in Supramolecular Complexes, Chem. Rev., 2016, 116, 5216–5300 CrossRef CAS PubMed.
-
Mechanistic Aspects of Host–Guest Binding in Cucurbiturils: Physicochemical Properties, ed. J. Mohanty, S. D. Choudhury, N. Barooah, H. Pal and A. C. Bhasikuttan, Elsevier, 2017 Search PubMed.
- C. Marquez and W. M. Nau, Two Mechanisms of Slow Host-Guest Complexation between Cucurbit[6]uril and Cyclohexylmethylamine: pH-Responsive Supramolecular Kinetics, Angew. Chem., Int. Ed., 2001, 40, 3155–3160 CrossRef CAS PubMed.
- F. Biedermann and W. M. Nau, Noncovalent Chirality Sensing Ensembles for the Detection and Reaction Monitoring of Amino Acids, Peptides, Proteins, and Aromatic Drugs, Angew. Chem., Int. Ed., 2014, 53, 5694–5699 CrossRef CAS PubMed.
-
Cucurbituril-based Sensors and Assays, ed. A. Hennig and W. M. Nau, The Royal Society of Chemistry, 2019 Search PubMed.
- S. Sinn and F. Biedermann, Chemical Sensors Based on Cucurbit[n]uril Macrocycles, Isr. J. Chem., 2018, 58, 357–412 CrossRef CAS.
- R. N. Dsouza, U. Pischel and W. M. Nau, Fluorescent Dyes and Their Supramolecular Host/Guest Complexes with Macrocycles in Aqueous Solution, Chem. Rev., 2011, 111, 7941–7980 CrossRef CAS PubMed.
- A. I. Lazar, J. Rohacova and W. M. Nau, Comparison of Complexation-Induced pKa Shifts in the Ground and Excited States of Dyes as Well as Different Macrocyclic Hosts and Their Manifestation in Host-Retarded Excited-Dye Deprotonation, J. Phys. Chem. B, 2017, 121, 11390–11398 CrossRef CAS PubMed.
- A. Hennig, H. Bakirci and W. M. Nau, Label-free continuous enzyme assays with macrocycle-fluorescent dye complexes, Nat. Methods, 2007, 4, 629–632 CrossRef CAS PubMed.
- A. L. Koner and W. M. Nau, Cucurbituril Encapsulation of Fluorescent Dyes, Supramol. Chem., 2007, 19, 55–66 CrossRef CAS.
- N. Saleh, A. L. Koner and W. M. Nau, Activation and Stabilization of Drugs by Supramolecular pKa Shifts: Drug-Delivery Applications Tailored for Cucurbiturils, Angew. Chem., Int. Ed., 2008, 47, 5398–5401 CrossRef CAS PubMed.
- D. H. Macartney, Encapsulation of Drug Molecules by Cucurbiturils: Effects on their Chemical Properties in Aqueous Solution, Isr. J. Chem., 2011, 51, 600–615 CrossRef CAS.
- I. Ghosh and W. M. Nau, The strategic use of supramolecular pKa shifts to enhance the bioavailability of drugs, Adv. Drug Delivery Rev., 2012, 64, 764–783 CrossRef CAS PubMed.
- S. Mecozzi and J. J. Rebek, The 55% Solution: A Formula for Molecular Recognition in the Liquid State, Chem. – Eur. J., 1998, 4, 1016–1022 CrossRef CAS.
- L. M. Grimm, S. Spicher, B. Tkachenko, P. R. Schreiner, S. Grimme and F. Biedermann, The Role of Packing, Dispersion, Electrostatics, and Solvation in High-Affinity Complexes of Cucurbit[n]urils with Uncharged Polar Guests, Chem. – Eur. J., 2022, 28, e202200529 CrossRef CAS PubMed.
- F. Biedermann, V. D. Uzunova, O. A. Scherman, W. M. Nau and A. De Simone, Release of High-Energy Water as an Essential Driving Force for the High-Affinity Binding of Cucurbit[n]urils, J. Am. Chem. Soc., 2012, 134, 15318–15323 CrossRef CAS PubMed.
- F. Biedermann, M. Vendruscolo, O. A. Scherman, A. De Simone and W. M. Nau, Cucurbit[8]uril and Blue-Box: High-Energy Water Release Overwhelms Electrostatic Interactions, J. Am. Chem. Soc., 2013, 135, 14879–14888 CrossRef CAS PubMed.
- F. Biedermann, W. M. Nau and H.-J. Schneider, The Hydrophobic Effect Revisited-Studies with Supramolecular Complexes Imply High-Energy Water as a Noncovalent Driving Force, Angew. Chem., Int. Ed., 2014, 53, 11158–11171 CrossRef CAS PubMed.
- W. Liu, S. K. Samanta, B. D. Smith and L. Isaacs, Synthetic mimics of biotin/(strept)avidin, Chem. Soc. Rev., 2017, 46, 2391–2403 RSC.
- D. Shetty, J. K. Khedkar, K. M. Park and K. Kim, Can we beat the biotin–avidin pair?: cucurbit[7]uril-based ultrahigh affinity host–guest complexes and their applications, Chem. Soc. Rev., 2015, 44, 8747–8761 RSC.
- M. V. Rekharsky, T. Mori, C. Yang, Y. H. Ko, N. Selvapalam, H. Kim, D. Sobransingh, A. E. Kaifer, S. Liu, L. Isaacs, W. Chen, S. Moghaddam, M. K. Gilson, K. Kim and Y. Inoue, A synthetic host-guest system achieves avidin-biotin affinity by overcoming enthalpy-entropy compensation, Proc. Natl. Acad. Sci. U. S. A., 2007, 104, 20737–20742 CrossRef CAS PubMed.
- S. Moghaddam, C. Yang, M. Rekharsky, Y. H. Ko, K. Kim, Y. Inoue and M. K. Gilson, New ultrahigh affinity host-guest complexes of cucurbit[7]uril with bicyclo[2.2.2]octane and adamantane guests: thermodynamic analysis and evaluation of M2 affinity calculations, J. Am. Chem. Soc., 2011, 133, 3570–3581 CrossRef CAS PubMed.
- S. Liu, C. Ruspic, P. Mukhopadhyay, S. Chakrabarti, P. Y. Zavalij and L. Isaacs, The Cucurbit[n]uril Family: Prime Components for Self-Sorting Systems, J. Am. Chem. Soc., 2005, 127, 15959–15967 CrossRef CAS PubMed.
- Y. H. Ko, I. Hwang, D.-W. Lee and K. Kim, Ultrastable Host-Guest Complexes and Their Applications, Isr. J. Chem., 2011, 51, 506–514 CrossRef CAS.
- M. A. Alnajjar, W. M. Nau and A. Hennig, A reference scale of cucurbit[7]uril binding affinities, Org. Biomol. Chem., 2021, 19, 8521–8529 RSC.
-
Cyclodextrins and Their Complexes: Chemistry, Analytical Methods, Applications, ed. H. Dodziuk, Wiley-VCH, Weinheim, Germany, 2006 Search PubMed.
- J. Murray, K. Kim, T. Ogoshi, W. Yao and B. C. Gibb, The aqueous supramolecular chemistry of cucurbit[n]urils, pillar[n]arenes and deep-cavity cavitands, Chem. Soc. Rev., 2017, 46, 2479–2496 RSC.
-
Calixarenes Revisited, ed. C. D. Gutsche, The Royal Society of Chemistry, Hertfordshire, UK, 1998 Search PubMed.
- Z. Ahmad, A. Shah, M. Siddiq and H.-B. Kraatz, Polymeric micelles as drug delivery vehicles, RSC Adv., 2014, 4, 17028–17038 RSC.
- M. Ghezzi, S. Pescina, C. Padula, P. Santi, E. Del Favero, L. Cantù and S. Nicoli, Polymeric micelles in drug delivery: An insight of the techniques for their characterization and assessment in biorelevant conditions, J. Controlled Release, 2021, 332, 312–336 CrossRef CAS PubMed.
- A. S. Chauhan, Dendrimers for Drug Delivery, Molecules, 2018, 23, 938 CrossRef PubMed.
- T. M. Allen and P. R. Cullis, Liposomal drug delivery systems: From concept to clinical applications, Adv. Drug Delivery Rev., 2013, 65, 36–48 CrossRef CAS PubMed.
-
F. Cramer, K. Freudenberg and H. Plieninger, Verfahren zur Herstellung von Einschlussverbindungen physiologisch wirksamer organischer Verbindungen, DE895769C, 1951.
- T. Loftsson and M. E. Brewster, Pharmaceutical applications of cyclodextrins: basic science and product development, J. Pharm. Pharmacol., 2010, 62, 1607–1621 CrossRef CAS PubMed.
- K. Uekama, F. Hirayama and T. Irie, Cyclodextrin Drug Carrier Systems, Chem. Rev., 1998, 98, 2045–2076 CrossRef CAS PubMed.
- A. Gu and N. J. Wheate, Macrocycles as drug-enhancing excipients in pharmaceutical formulations, J. Inclusion Phenom. Macrocyclic Chem., 2021, 100, 55–69 CrossRef CAS.
- D. Garcia Jimenez, V. Poongavanam and J. Kihlberg, Macrocycles in Drug Discovery–Learning from the Past for the Future, J. Med. Chem., 2023, 66, 5377–5396 CrossRef CAS PubMed.
- F. Giordanetto and J. Kihlberg, Macrocyclic Drugs and Clinical Candidates: What Can Medicinal Chemists Learn from Their Properties?, J. Med. Chem., 2014, 57, 278–295 CrossRef CAS PubMed.
-
Inclusion Complexes in Drug Delivery and Drug Targeting: Formation, Characterization, and Biological Applications, ed. R. K. K. Sanku, O. O. Karakus, M. Ilies and M. A. Ilies, American Chemical Society, Washington, DC, 2019 Search PubMed.
-
H. Yin, Z. Wang and R. Wang, in Handbook of Macrocyclic Supramolecular Assembly, ed. Y. Liu, Y. Chen and H.-Y. Zhang, Springer Singapore, Singapore, 2019, pp. 1–25 Search PubMed.
- S. Yi and A. E. Kaifer, Determination of the Purity of Cucurbit[n]uril (n = 7, 8) Host Samples, J. Org. Chem., 2011, 76, 10275–10278 CrossRef CAS PubMed.
-
K. Kim, S. Samal, R. N. Kumar, N. Selvapalam and D.-H. Oh, Processes of preparing glycolurils and cucurbiturils using microwave, WO05/103053, 2005.
- N. J. Wheate, N. Patel and O. B. Sutcliffe, Microwave synthesis of cucurbit[n]urils, Future Med. Chem., 2010, 2, 231–236 CrossRef CAS PubMed.
- W. Xu and C. Li, Efficient synthesis of cucurbiturils and their derivatives using mechanochemical high-speed ball milling (HSBM), High Perform. Polym., 2020, 33, 509–518 CrossRef.
- D. A. Uhlenheuer, K. Petkau and L. Brunsveld, Combining supramolecular chemistry with biology, Chem. Soc. Rev., 2010, 39, 2817–2826 RSC.
- C. Hou, X. Zeng, Y. Gao, S. Qiao, X. Zhang, J. Xu and J. Liu, Cucurbituril As A Versatile Tool to Tune the Functions of Proteins, Isr. J. Chem., 2017, 58, 286–295 CrossRef.
- Y. H. Liu, Y. M. Zhang, H. J. Yu and Y. Liu, Cucurbituril-Based Biomacromolecular Assemblies, Angew. Chem., Int. Ed., 2021, 60, 3870–3880 CrossRef CAS PubMed.
- G. Cheng, J. Luo, Y. Liu, X. Chen, Z. Wu and T. Chen, Cucurbituril-Oriented Nanoplatforms in Biomedical Applications, ACS Appl. Bio Mater., 2020, 3, 8211–8240 CrossRef CAS PubMed.
- J. Krämer, R. Kang, L. M. Grimm, L. De Cola, P. Picchetti and F. Biedermann, Molecular Probes, Chemosensors, and Nanosensors for Optical Detection of Biorelevant Molecules and Ions in Aqueous Media and Biofluids, Chem. Rev., 2022, 122, 3459–3636 CrossRef PubMed.
- H. Nie, Z. Wei, X.-L. Ni and Y. Liu, Assembly and Applications of Macrocyclic-Confinement-Derived Supramolecular Organic Luminescent Emissions from Cucurbiturils, Chem. Rev., 2022, 122, 9032–9077 CrossRef CAS PubMed.
- Q. Wu, Q. Lei, H.-C. Zhong, T.-B. Ren, Y. Sun, X.-B. Zhang and L. Yuan, Fluorophore-based host–guest assembly complexes for imaging and therapy, Chem. Commun., 2023, 59, 3024–3039 RSC.
-
F. Liu and S. Liu, in Handbook of Macrocyclic Supramolecular Assembly, ed. Y. Liu, Y. Chen and H.-Y. Zhang, Springer Singapore, Singapore, 2020, pp. 731–757 Search PubMed.
- O. A. Gerasko, E. A. Kovalenko and V. P. Fedin, Macrocyclic cavitands cucurbit[n]urils: prospects for application in biochemistry, medicine and nanotechnology, Russ. Chem. Rev., 2016, 85, 795–816 CrossRef CAS.
- H. Yin, Q. Cheng, D. Bardelang and R. Wang, Challenges and Opportunities of Functionalized Cucurbiturils for Biomedical Applications, JACS Au, 2023, 3, 2356–2377 CrossRef CAS PubMed.
- B. Hazarika and V. P. Singh, Macrocyclic supramolecular biomaterials in anti-cancer therapeutics, Chin. Chem. Lett., 2023, 34, 108220 CrossRef CAS.
- J. Chen, R. J. Hooley and W. Zhong, Applications of Synthetic Receptors in Bioanalysis and Drug Transport, Bioconjugate Chem., 2022, 33, 2245–2253 CrossRef CAS PubMed.
- S. Walker, R. Oun, F. J. McInnes and N. J. Wheate, The Potential of Cucurbit[n]urils in Drug Delivery, Isr. J. Chem., 2011, 51, 616–624 CrossRef CAS.
-
Cucurbiturils in Drug Binding and Delivery, ed. D. H. Macartney, Elsevier, 2017 Search PubMed.
-
A. I. Day and J. G. Collins, in Supramolecular Chemistry: From Molecules to Nanomaterials, ed. P. A. Gale and J. W. Steed, John Wiley & Sons, Ltd, Chichester, UK, 2012, pp. 983–1000 Search PubMed.
- D. Das, K. I. Assaf and W. M. Nau, Applications of Cucurbiturils in Medicinal Chemistry and Chemical Biology, Front. Chem., 2019, 7, 619 CrossRef CAS PubMed.
- C.-L. Deng, S. L. Murkli and L. D. Isaacs, Supramolecular hosts as in vivo sequestration agents for pharmaceuticals and toxins, Chem. Soc. Rev., 2020, 49, 7516–7532 RSC.
- C. Sabin, S. Sam, A. Hrishikes, B. Salin, P. N. Vigneshkumar, J. George and F. John, Supramolecular Drug Delivery Systems Based on Host-Guest Interactions for Nucleic Acid Delivery, ChemistrySelect, 2022, 7, e202203644 CrossRef CAS.
- N. Barooah, J. Mohanty and A. C. Bhasikuttan, Cucurbituril-Based Supramolecular Assemblies: Prospective on Drug Delivery, Sensing, Separation, and Catalytic Applications, Langmuir, 2022, 38, 6249–6264 CrossRef CAS PubMed.
- N. J. Wheate and C. Limantoro, Cucurbit[n]urils as excipients in pharmaceutical dosage forms, Supramol. Chem., 2016, 28, 849–856 CrossRef CAS.
- E. Y. Chernikova and D. V. Berdnikova, Cucurbiturils in nucleic acids research, Chem. Commun., 2020, 56, 15360–15376 RSC.
- H. Yin, X. Zhang, J. Wei, S. Lu, D. Bardelang and R. Wang, Recent advances in supramolecular antidotes, Theranostics, 2021, 11, 1513–1526 CrossRef CAS PubMed.
- H. Yin, D. Bardelang and R. Wang, Macrocycles and Related Hosts as Supramolecular Antidotes, Trends Chem., 2021, 3, 1–4 CrossRef CAS.
- J. Vázquez, P. Remón, R. N. Dsouza, A. I. Lazar, J. F. Arteaga, W. M. Nau and U. Pischel, A Simple Assay for Quality Binders to Cucurbiturils, Chem. – Eur. J., 2014, 20, 9897–9901 CrossRef PubMed.
- F. Wu, L.-H. Wu, X. Xiao, Y.-Q. Zhang, S.-F. Xue, Z. Tao and A. I. Day, Locating the Cyclopentano Cousins of the Cucurbit[n]uril Family, J. Org. Chem., 2011, 77, 606–611 CrossRef PubMed.
- Y. Zhao, V. Mandadapu, H. Iranmanesh, J. E. Beves and A. I. Day, The Inheritance Angle: A Determinant for the Number of Members in the Substituted Cucurbit[n]uril Family, Org. Lett., 2017, 19, 4034–4037 CrossRef CAS PubMed.
- S. Pandey, D. V. D. W. Kankanamalage, X. Zhou, C. Hu, L. Isaacs, J. Jayawickramarajah and H. Mao, Chaperone-Assisted Host–Guest Interactions Revealed by Single-Molecule Force Spectroscopy, J. Am. Chem. Soc., 2019, 141, 18385–18389 CrossRef CAS PubMed.
- X. Yang, K. Varini, M. Godard, F. Gassiot, R. Sonnette, G. Ferracci, B. Pecqueux, V. Monnier, L. Charles, S. Maria, M. Hardy, O. Ouari, M. Khrestchatisky, P. Lécorché, G. Jacquot and D. Bardelang, Preparation and In Vitro Validation of a Cucurbit[7]uril-Peptide Conjugate Targeting the LDL Receptor, J. Med. Chem., 2023, 66, 8844–8857 CrossRef CAS PubMed.
- L. Kociscakova, C. Rando, M. Kozlikova, M. Machacek, V. Novakova, V. Šindelář and P. Zimcik, Monomerization of Phthalocyanines in Water via Their Supramolecular Interactions with Cucurbiturils, J. Org. Chem., 2023, 88, 988–1002 CrossRef CAS PubMed.
- D. W. Frank, J. E. Gray and R. N. Weaver, Cyclodextrin nephrosis in the rat, Am. J. Pathol., 1976, 83, 367–382 CAS.
- V. J. Stella and Q. He, Cyclodextrins, Toxicol. Pathol., 2008, 36, 30–42 CrossRef CAS PubMed.
- V. D. Uzunova, C. Cullinane, K. Brix, W. M. Nau and A. I. Day, Toxicity of cucurbit[7]uril and cucurbit[8]uril: an exploratory in vitro and in vivo study, Org. Biomol. Chem., 2010, 8, 2037–2042 RSC.
- G. Hettiarachchi, D. Nguyen, J. Wu, D. Lucas, D. Ma, L. Isaacs and V. Briken, Toxicology and drug delivery by cucurbit[n]uril type molecular containers, PLoS One, 2010, 5, e10514 CrossRef PubMed.
- R. Oun, R. S. Floriano, L. Isaacs, E. G. Rowan and N. J. Wheate, The ex vivo neurotoxic, myotoxic and cardiotoxic activity of cucurbituril-based macrocyclic drug delivery vehicles, Toxicol. Res., 2014, 3, 447–455 CrossRef CAS PubMed.
- H. Chen, J. Y. W. Chan, X. Yang, I. W. Wyman, D. Bardelang, D. H. Macartney, S. M. Y. Lee and R. Wang, Developmental and organ-specific toxicity of cucurbit[7]uril: in vivo study on zebrafish models, RSC Adv., 2015, 5, 30067–30074 RSC.
- X. Zhang, X. Xu, S. Li, L.-H. Wang, J. Zhang and R. Wang, A systematic evaluation of the biocompatibility of cucurbit[7]uril in mice, Sci. Rep., 2018, 8, 8819 CrossRef PubMed.
- A. Aktanova, T. Abramova, E. Pashkina, O. Boeva, L. Grishina, E. Kovalenko and V. Kozlov, Assessment of the Biocompatibility of Cucurbiturils in Blood Cells, Nanomaterials, 2021, 11, 1356 CrossRef CAS PubMed.
- E. Pashkina, A. Aktanova, E. Blinova, I. Mirzaeva, E. Kovalenko, N. Knauer, A. Ermakov and V. Kozlov, Evaluation of the Immunosafety of Cucurbit[n]uril on Peripheral Blood Mononuclear Cells In Vitro, Molecules, 2020, 25, 3388 CrossRef CAS PubMed.
- Y. Chen, Z. Huang, J.-F. Xu, Z. Sun and X. Zhang, Cytotoxicity Regulated by Host–Guest Interactions: A Supramolecular Strategy to Realize Controlled Disguise and Exposure, ACS Appl. Mater. Interfaces, 2016, 8, 22780–22784 CrossRef CAS PubMed.
- S. A. Fahmy, J. Brüßler, M. Alawak, M. M. H. El-Sayed, U. Bakowsky and T. Shoeib, Chemotherapy Based on Supramolecular Chemistry: A Promising Strategy in Cancer Therapy, Pharmaceutics, 2019, 11, 292 CrossRef CAS PubMed.
- S. Shukla, B. Sagar, A. K. Sood, A. Gaur, S. Batra and S. Gulati, Supramolecular Chemotherapy with Cucurbit[n]urils as Encapsulating Hosts, ACS Appl. Bio Mater., 2023, 6, 2089–2101 CrossRef CAS PubMed.
- D. Prashar, Y. Shi, D. Bandyopadhyay, J. C. Dabrowiak and Y.-Y. Luk, Adamantane–platinum conjugate hosted in β-cyclodextrin: Enhancing transport and cytotoxicity by noncovalent modification, Bioorg. Med. Chem. Lett., 2011, 21, 7421–7425 CrossRef CAS PubMed.
- M. Sojka, J. Chyba, S. S. Paul, K. Wawrocka, K. Hönigová, B. J. R. Cuyacot, A. C. Castro, T. Vaculovič, J. Marek, M. Repisky, M. Masařík, J. Novotný and R. Marek, Supramolecular Coronation of Platinum(II) Complexes by Macrocycles: Structure, Relativistic DFT Calculations, and Biological Effects, Inorg. Chem., 2021, 60, 17911–17925 CrossRef CAS PubMed.
- J. Pejchal, P. Jošt, L. Múčková, R. Andrýs, M. Lísa and J. Zdarova Karasova, A systematic evaluation of the cucurbit[7]uril pharmacokinetics and toxicity after a single dose and short-term repeated administration in mice, Arch. Toxicol., 2022, 96, 1411–1421 CrossRef CAS PubMed.
- S. Ganapati and L. Isaacs, Acyclic Cucurbit[n]uril-type Receptors: Preparation, Molecular Recognition Properties and Biological Applications, Isr. J. Chem., 2018, 58, 250–263 CrossRef CAS PubMed.
- K. M. Park, K. Suh, H. Jung, D.-W. Lee, Y. Ahn, J. Kim, K. Baek and K. Kim, Cucurbituril-based nanoparticles: a new efficient vehicle for targeted intracellular delivery of hydrophobic drugs, Chem. Commun., 2009, 71–73 RSC.
- J. Kim, Y. Ahn, K. M. Park, Y. Kim, Y. H. Ko, D. H. Oh and K. Kim, Carbohydrate Wheels: Cucurbituril-Based Carbohydrate Clusters, Angew. Chem., Int. Ed., 2007, 46, 7393–7395 CrossRef CAS PubMed.
- P. Montes-Navajas, M. González-Béjar, J. C. Scaiano and H. García, Cucurbituril complexes cross the cell membrane, Photochem. Photobiol. Sci., 2009, 8, 1743–1747 CrossRef CAS PubMed.
- M. Li, A. Lee, S. Kim, A. Shrinidhi, K. M. Park and K. Kim, Cucurbit[7]uril-conjugated dyes as live cell imaging probes: investigation on their cellular uptake and excretion pathways, Org. Biomol. Chem., 2019, 17, 6215–6220 RSC.
- T. Dutta, S. Banerjee and A. L. Koner, Regulating endoplasmic reticulum localization of a fluorescent NBD adamantane conjugate by Cucurbit [7]uril, Chem. Phys. Impact, 2021, 3, 100051 CrossRef.
- W. Ong and A. E. Kaifer, Salt Effects on the Apparent Stability of the Cucurbit[7]uril−Methyl Viologen Inclusion Complex, J. Org. Chem., 2004, 69, 1383–1385 CrossRef CAS PubMed.
- S. Walker, R. Kaur, F. J. McInnes and N. J. Wheate, Synthesis, Processing and Solid State Excipient Interactions of Cucurbit[6]uril and Its Formulation into Tablets for Oral Drug Delivery, Mol. Pharmaceutics, 2010, 7, 2166–2172 CrossRef CAS PubMed.
- D. Ma, G. Hettiarachchi, D. Nguyen, B. Zhang, J. B. Wittenberg, P. Y. Zavalij, V. Briken and L. Isaacs, Acyclic cucurbit[n]uril molecular containers enhance the solubility and bioactivity of poorly soluble pharmaceuticals, Nat. Chem., 2012, 4, 503–510 CrossRef CAS PubMed.
- Y. Zhao, D. P. Buck, D. L. Morris, M. H. Pourgholami, A. I. Day and J. G. Collins, Solubilisation and cytotoxicity of albendazole encapsulated in cucurbit[n]uril, Org. Biomol. Chem., 2008, 6, 4509–4515 RSC.
- A. L. Koner, I. Ghosh, N. i. Saleh and W. M. Nau, Supramolecular encapsulation of benzimidazole-derived drugs by cucurbit[7]uril, Can. J. Chem., 2011, 89, 139–147 CrossRef CAS.
- N. Dong, S.-F. Xue, Q.-J. Zhu, Z. Tao, Y. Zhao and L.-X. Yang, Cucurbit[n]urils (n=7, 8) binding of camptothecin and the effects on solubility and reactivity of the anticancer drug, Supramol. Chem., 2008, 20, 663–671 CrossRef.
- F. J. McInnes, N. G. Anthony, A. R. Kennedy and N. J. Wheate, Solid state stabilisation of the orally delivered drugs atenolol, glibenclamide, memantine and paracetamol through their complexation with cucurbit[7]uril, Org. Biomol. Chem., 2010, 8, 765–773 RSC.
- N. J. Wheate, V. Vora, N. G. Anthony and F. J. McInnes, Host–guest complexes of the antituberculosis drugs pyrazinamide and isoniazid with cucurbit[7]uril, J. Inclusion Phenom. Macrocyclic Chem., 2010, 68, 359–367 CrossRef CAS.
- Y. Jin Jeon, S.-Y. Kim, Y. Ho Ko, S. Sakamoto, K. Yamaguchi and K. Kim, Novel molecular drug carrier: encapsulation of oxaliplatin in cucurbit[7]uril and its effects on stability and reactivity of the drug, Org. Biomol. Chem., 2005, 3, 2122 RSC.
- Y. Zhao, M. S. Bali, C. Cullinane, A. I. Day and J. G. Collins, Synthesis, cytotoxicity and cucurbituril binding of triamine linked dinuclear platinum complexes, Dalton Trans., 2009, 5190–5198 RSC.
- N. J. Wheate, Improving platinum(II)-based anticancer drug delivery using cucurbit[n]urils, J. Inorg. Biochem., 2008, 102, 2060–2066 CrossRef CAS PubMed.
- I. W. Wyman and D. H. Macartney, Host–guest complexations of local anaesthetics by cucurbit[7]uril in aqueous solution, Org. Biomol. Chem., 2010, 8, 247–252 RSC.
- H. Chen, J. Y. W. Chan, S. Li, J. J. Liu, I. W. Wyman, S. M. Y. Lee, D. H. Macartney and R. Wang,
In vivo reversal of general anesthesia by cucurbit[7]uril with zebrafish models, RSC Adv., 2015, 5, 63745–63752 RSC.
- S. Kemp, N. J. Wheate, F. H. Stootman and J. R. Aldrich-Wright, The Host-Guest Chemistry of Proflavine with Cucurbit[6,7,8]urils, Supramol. Chem., 2007, 19, 475–484 CrossRef CAS.
- H. Cong, C.-R. Li, S.-F. Xue, Z. Tao, Q.-J. Zhu and G. Wei, Cucurbituril-resisted acylation of the anti-tuberculosis drug isoniazidvia a supramolecular strategy, Org. Biomol. Chem., 2011, 9, 1041–1046 RSC.
- H. Feng, J. Kan, C. Redshaw, B. Bian, Z. Tao and X. Xiao, Supramolecular drug inclusion complex constructed from cucurbit[7]uril and the hepatitis B drug Adefovir, Supramol. Chem., 2018, 31, 260–267 CrossRef.
- D. R. Boraste, G. Chakraborty, A. K. Ray, G. S. Shankarling and H. Pal, Supramolecular host-guest interaction of antibiotic drug ciprofloxacin with cucurbit[7]uril macrocycle: Modulations in photophysical properties and enhanced photostability, J. Photochem. Photobiol., A, 2018, 358, 26–37 CrossRef CAS.
- N. J. Wheate, D. P. Buck, A. I. Day and J. G. Collins, Cucurbit[n]uril binding of platinum anticancer complexes, Dalton Trans., 2006, 451–458 RSC.
- M. A. Gamal-Eldin and D. H. Macartney, Cucurbit[7]uril host–guest complexes and [2]pseudorotaxanes with N-methylpiperidinium, N-methylpyrrolidinium, and N-methylmorpholinium cations in aqueous solution, Org. Biomol. Chem., 2013, 11, 1234–1241 RSC.
- D. H. Macartney, Cucurbit[n]uril type hosts for the reversal of steroidal neuromuscular blocking agents, Future Med. Chem., 2013, 5, 2075–2089 CrossRef CAS PubMed.
- D. Shaya and L. Isaacs, Acyclic Cucurbit[n]uril-Type Containers as Receptors for Neuromuscular Blocking Agents: Structure-Binding Affinity Relationships, Croat. Chem. Acta, 2019, 92, 163–171 CrossRef CAS PubMed.
- C. P. Carvalho, V. D. Uzunova, J. P. Da Silva, W. M. Nau and U. Pischel, A photoinduced pH jump applied to drug release from cucurbit[7]uril, Chem. Commun., 2011, 47, 8793–8795 RSC.
- Y. Wang and I. J. Dmochowski, Cucurbit[6]uril is an ultrasensitive 129Xe NMR contrast agent, Chem. Commun., 2015, 51, 8982–8985 RSC.
- L. Liu, Controlled release from cucurbituril, J. Inclusion Phenom. Macrocyclic Chem., 2016, 87, 1–12 CrossRef.
- J. Vázquez, M. A. Romero, R. N. Dsouza and U. Pischel, Phototriggered release of amine from a cucurbituril macrocycle, Chem. Commun., 2016, 52, 6245–6248 RSC.
- N. Basílio and U. Pischel, Drug Delivery by Controlling a Supramolecular Host-Guest Assembly with a Reversible Photoswitch, Chem. – Eur. J., 2016, 22, 15208–15211 CrossRef PubMed.
- M. A. Romero, R. J. Fernandes, A. J. Moro, N. Basílio and U. Pischel, Light-induced cargo release from a cucurbit[8]uril host by means of a sequential logic operation, Chem. Commun., 2018, 54, 13335–13338 RSC.
- S. Zhang, L. Grimm, Z. Miskolczy, L. Biczók, F. Biedermann and W. M. Nau, Binding affinities of cucurbit[n]urils with cations, Chem. Commun., 2019, 55, 14131–14134 RSC.
- S. D. Choudhury, J. Mohanty, H. Pal and A. C. Bhasikuttan, Cooperative Metal Ion Binding to a Cucurbit[7]uril−Thioflavin T Complex: Demonstration of a Stimulus-Responsive Fluorescent Supramolecular Capsule, J. Am. Chem. Soc., 2010, 132, 1395–1401 CrossRef CAS PubMed.
- W. M. Nau, Under control, Nat. Chem., 2010, 2, 248–250 CrossRef CAS PubMed.
- P. Remón, D. González, M. A. Romero, N. Basílio and U. Pischel, Chemical signal cascading in a supramolecular network, Chem. Commun., 2020, 56, 3737–3740 RSC.
- H. Bai, H. Yuan, C. Nie, B. Wang, F. Lv, L. Liu and S. Wang, A Supramolecular Antibiotic Switch for Antibacterial Regulation, Angew. Chem., Int. Ed., 2015, 54, 13208–13213 CrossRef CAS PubMed.
- G. Ghale, A. G. Lanctôt, H. T. Kreissl, M. H. Jacob, H. Weingart, M. Winterhalter and W. M. Nau, Chemosensing Ensembles for Monitoring Biomembrane Transport in Real Time, Angew. Chem., Int. Ed., 2014, 53, 2762–2765 CrossRef CAS PubMed.
- P. Ferreira, B. Ventura, A. Barbieri, J. P. Da Silva, C. A. T. Laia, A. J. Parola and N. Basílio, A Visible–Near-Infrared Light-Responsive Host–Guest Pair with Nanomolar Affinity in Water, Chem. – Eur. J., 2019, 25, 3477–3482 CrossRef CAS PubMed.
- Y. Chen, Z. Huang, H. Zhao, J.-F. Xu, Z. Sun and X. Zhang, Supramolecular Chemotherapy: Cooperative Enhancement of Antitumor Activity by Combining Controlled Release of Oxaliplatin and Consuming of Spermine by Cucurbit[7]uril, ACS Appl. Mater. Interfaces, 2017, 9, 8602–8608 CrossRef CAS PubMed.
- N. Barooah, A. Kunwar, R. Khurana, A. C. Bhasikuttan and J. Mohanty, Stimuli-Responsive Cucurbit[7]uril-Mediated BSA Nanoassembly for Uptake and Release of Doxorubicin, Chem. – Asian J., 2017, 12, 122–129 CrossRef CAS PubMed.
- A. A. Gokaltun, L. Fan, L. Mazzaferro, D. Byrne, M. L. Yarmush, T. Dai, A. Asatekin and O. B. Usta, Supramolecular hybrid hydrogels as rapidly on-demand dissoluble, self-healing, and biocompatible burn dressings, Bioact. Mater., 2023, 25, 415–429 CAS.
- N. Das Saha, S. Pradhan, R. Sasmal, A. Sarkar, C. M. Berač, J. C. Kölsch, M. Pahwa, S. Show, Y. Rozenholc, Z. Topçu, V. Alessandrini, J. Guibourdenche, V. Tsatsaris, N. Gagey-Eilstein and S. S. Agasti, Cucurbit[7]uril Macrocyclic Sensors for Optical Fingerprinting: Predicting Protein Structural Changes to Identifying Disease-Specific Amyloid Assemblies, J. Am. Chem. Soc., 2022, 144, 14363–14379 CrossRef CAS PubMed.
- C. L. Maikawa, A. I. d'Aquino, E. T. Vuong, B. Su, L. Zou, P. C. Chen, L. T. Nguyen, A. A. A. Autzen, J. L. Mann, M. J. Webber and E. A. Appel, Affinity-Directed Dynamics of Host–Guest Motifs for Pharmacokinetic Modulation via Supramolecular PEGylation, Biomacromolecules, 2021, 22, 3565–3573 CrossRef CAS PubMed.
- N. Dubel, S. Liese, F. Scherz and O. Seitz, Exploring the Limits of Bivalency by DNA-Based Spatial Screening, Angew. Chem., Int. Ed., 2018, 58, 907–911 CrossRef PubMed.
- O. Seitz, Templated chemistry for bioorganic synthesis and chemical biology, J. Pept. Sci., 2019, 25, e3198 CrossRef PubMed.
- S. B. Yeldell and O. Seitz, Nucleic acid constructs for the interrogation of multivalent protein interactions, Chem. Soc. Rev., 2020, 49, 6848–6865 RSC.
- L. Zou, A. S. Braegelman and M. J. Webber, Spatially Defined Drug Targeting by in Situ Host-Guest Chemistry in a Living Animal, ACS Cent. Sci., 2019, 5, 1035–1043 CrossRef CAS PubMed.
- S. Ghosh and L. Isaacs, Biological Catalysis Regulated by Cucurbit[7]uril Molecular Containers, J. Am. Chem. Soc., 2010, 132, 4445–4454 CrossRef CAS PubMed.
- X. Zhou, X. Su, P. Pathak, R. Vik, B. Vinciguerra, L. Isaacs and J. Jayawickramarajah, Host-Guest Tethered DNA Transducer: ATP Fueled Release of a Protein Inhibitor from Cucurbit[7]uril, J. Am. Chem. Soc., 2017, 139, 13916–13921 CrossRef CAS PubMed.
- Z. Yu, M. Ai, S. K. Samanta, F. Hashiya, J. Taniguchi, S. Asamitsu, S. Ikeda, K. Hashiya, T. Bando, G. N. Pandian, L. Isaacs and H. Sugiyama, A synthetic transcription factor pair mimic for precise recruitment of an epigenetic modifier to the targeted DNA locus, Chem. Commun., 2020, 56, 2296–2299 RSC.
- S.-R. Wang, J.-Q. Wang, G.-H. Xu, L. Wei, B.-S. Fu, L.-Y. Wu, Y.-Y. Song, X.-R. Yang, C. Li, S.-M. Liu and X. Zhou, The Cucurbit[7]Uril-Based Supramolecular Chemistry for Reversible B/Z-DNA Transition, Adv. Sci., 2018, 5, 1800231 CrossRef PubMed.
- S.-R. Wang, Y.-Y. Song, L. Wei, C.-X. Liu, B.-S. Fu, J.-Q. Wang, X.-R. Yang, Y.-N. Liu, S.-M. Liu, T. Tian and X. Zhou, Cucurbit[7]uril-Driven Host–Guest Chemistry for Reversible Intervention of 5-Formylcytosine-Targeted Biochemical Reactions, J. Am. Chem. Soc., 2017, 139, 16903–16912 CrossRef CAS PubMed.
- D. V. D. W. Kankanamalage, J. H. T. Tran, N. Beltrami, K. Meng, X. Zhou, P. Pathak, L. Isaacs, A. L. Burin, M. F. Ali and J. Jayawickramarajah, DNA Strand Displacement Driven by Host–Guest Interactions, J. Am. Chem. Soc., 2022, 144, 16502–16511 CrossRef CAS PubMed.
- J. An, S. Kim, A. Shrinidhi, J. Kim, H. Banna, G. Sung, K. M. Park and K. Kim, Purification of protein therapeutics via high-affinity supramolecular host–guest interactions, Nat. Biomed. Eng., 2020, 4, 1044–1052 CrossRef CAS PubMed.
- L. Li, M. Liu, L. Yue, R. Wang, N. Zhang, Y. Liang, L. Zhang, L. Cheng, J. Xia and R. Wang, Host–Guest Protein Assembly for Affinity Purification of Methyllysine Proteomes, Anal. Chem., 2020, 92, 9322–9329 CrossRef CAS PubMed.
- C. Sun, Z. Wang, L. Yue, Q. Huang, Q. Cheng and R. Wang, Supramolecular Induction of Mitochondrial Aggregation and Fusion, J. Am. Chem. Soc., 2020, 142, 16523–16527 CrossRef CAS PubMed.
- C. Gao, Q. Cheng, J. Li, J. Chen, Q. Wang, J. Wei, Q. Huang, S. M. Y. Lee, D. Gu and R. Wang, Supramolecular Macrophage-Liposome Marriage for Cell-Hitchhiking Delivery and Immunotherapy of Acute Pneumonia and Melanoma, Adv. Funct. Mater., 2021, 31, 2102440 CrossRef CAS.
- A. C. Sedgwick, J. T. Brewster, T. Wu, X. Feng, S. D. Bull, X. Qian, J. L. Sessler, T. D. James, E. V. Anslyn and X. Sun, Indicator displacement assays (IDAs): the past, present and future, Chem. Soc. Rev., 2021, 50, 9–38 RSC.
- W. Li, N. W. Kuehne, E. Dallin, R. Gordon and F. Hof, A supramolecular indicator displacement assay for acetyl amantadine, a proxy biomarker for spermidine/spermine N1-acetyltransferase (SSAT) activity, Can. J. Chem., 2016, 94, 969–975 CrossRef CAS.
- X. Du, X. Liu, H. Su, X. Cheng, L. Li, H. Gu, X. Xing, D. Qiu and H. Hao, A simple AIEgen photosensitizer with cucurbit[7]uril selective detection amantadine and application in mitochondrion imaging, Microchem. J., 2022, 182, 107942 CrossRef CAS.
- M. del Pozo, Á. Fernández and C. Quintana, On-line competitive host-guest interactions in a turn-on fluorometric method to amantadine determination in human serum and pharmaceutical formulations, Talanta, 2018, 179, 124–130 CrossRef CAS PubMed.
- G. Jiang, W. Zhu, Q. Chen, X. Li, G. Zhang, Y. Li, X. Fan and J. Wang, Selective fluorescent probes for spermine and 1-adamantanamine based on the supramolecular structure formed between AIE-active molecule and cucurbit[n]urils, Sens. Actuators, B, 2018, 261, 602–607 CrossRef CAS.
- S. Sinn, E. Spuling, S. Bräse and F. Biedermann, Rational design and implementation of a cucurbit[8]uril-based indicator-displacement assay for application in blood serum, Chem. Sci., 2019, 10, 6584–6593 RSC.
- N. M. Kumar, P. Picchetti, C. Hu, L. M. Grimm and F. Biedermann, Chemiluminescent Cucurbit[n]uril-Based Chemosensor for the Detection of Drugs in Biofluids, ACS Sens., 2022, 7, 2312–2319 CrossRef CAS PubMed.
- C. Hu, L. Grimm, A. Prabodh, A. Baksi, A. Siennicka, P. A. Levkin, M. M. Kappes and F. Biedermann, Covalent cucurbit[7]uril–dye conjugates for sensing in aqueous saline media and biofluids, Chem. Sci., 2020, 11, 11142–11153 RSC.
- C. Hu, T. Jochmann, P. Chakraborty, M. Neumaier, P. A. Levkin, M. M. Kappes and F. Biedermann, Further Dimensions for Sensing in Biofluids: Distinguishing Bioorganic Analytes by the Salt-Induced Adaptation of a Cucurbit[7]uril-Based Chemosensor, J. Am. Chem. Soc., 2022, 144, 13084–13095 CrossRef CAS PubMed.
- L. Zhu, Z. Zhao, X. Zhang, H. Zhang, F. Liang and S. Liu, A Highly Selective and Strong Anti-Interference Host-Guest Complex as Fluorescent Probe for Detection of Amantadine by Indicator Displacement Assay, Molecules, 2018, 23, 947 CrossRef PubMed.
- Z. Xie, M. Yang, L. Luo, Y. Lv, K. Song, S. Liu, D. Chen and J. Wang, Nanochannel sensor for sensitive and selective adamantanamine detection based on host-guest competition, Talanta, 2020, 219, 121213 CrossRef CAS PubMed.
- C. S. H. Domínguez and P. Hernández, Use of Graphene and Cucurbit[7]uril Electrodes for the Determination of Amantadine in Biological Fluids, Am. J. Anal. Chem., 2015, 06, 623–630 CrossRef.
- S. Cao, P. Wang, X. Zeng, Z. Tao and X.-L. Ni, Cucurbituril-assisted formation of tunable carbon dots from single organic precursors in water, Org. Chem. Front., 2021, 8, 224–230 RSC.
- B. Gong, B.-K. Choi, J.-Y. Kim, D. Shetty, Y. H. Ko, N. Selvapalam, N. K. Lee and K. Kim, High Affinity Host–Guest FRET Pair for Single-Vesicle Content-Mixing Assay: Observation of Flickering Fusion Events, J. Am. Chem. Soc., 2015, 137, 8908–8911 CrossRef CAS PubMed.
- M. Li, A. Lee, K. L. Kim, J. Murray, A. Shrinidhi, G. Sung, K. M. Park and K. Kim, Autophagy Caught in the Act: A Supramolecular FRET Pair Based on an Ultrastable Synthetic Host-Guest Complex Visualizes Autophagosome-Lysosome Fusion, Angew. Chem., Int. Ed., 2018, 57, 2120–2125 CrossRef CAS PubMed.
- A. Lee, M. Li, Y. H. Ko, S. Park, J. Seo, K. M. Park and K. Kim, Visualization of lipophagy using a supramolecular FRET pair, Chem. Commun., 2021, 57, 12179–12182 RSC.
- K. L. Kim, G. Sung, J. Sim, J. Murray, M. Li, A. Lee, A. Shrinidhi, K. M. Park and K. Kim, Supramolecular latching system based on ultrastable synthetic binding pairs as versatile tools for protein imaging, Nat. Commun., 2018, 9, 1712 CrossRef PubMed.
- M. Li, S. Kim, A. Lee, A. Shrinidhi, Y. H. Ko, H. G. Lim, H. H. Kim, K. B. Bae, K. M. Park and K. Kim, Bio-orthogonal Supramolecular Latching inside Live Animals and Its Application for in Vivo Cancer Imaging, ACS Appl. Mater. Interfaces, 2019, 11, 43920–43927 CrossRef CAS PubMed.
- J. Murray, J. Sim, K. Oh, G. Sung, A. Lee, A. Shrinidhi, A. Thirunarayanan, D. Shetty and K. Kim, Enrichment of Specifically Labeled Proteins by an Immobilized Host Molecule, Angew. Chem., Int. Ed., 2017, 56, 2395–2398 CrossRef CAS PubMed.
- R. Sasmal, N. Das Saha, M. Pahwa, S. Rao, D. Joshi, M. S. Inamdar, V. Sheeba and S. S. Agasti, Synthetic Host-Guest Assembly in Cells and Tissues: Fast, Stable, and Selective Bioorthogonal Imaging via Molecular Recognition, Anal. Chem., 2018, 90, 11305–11314 CrossRef CAS PubMed.
- Y. Li, Y. Dong, X. Miao, Y. Ren, B. Zhang, P. Wang, Y. Yu, B. Li, L. Isaacs and L. Cao, Shape-Controllable and Fluorescent Supramolecular Organic Frameworks Through Aqueous Host–Guest Complexation, Angew. Chem., Int. Ed., 2018, 57, 729–733 CrossRef CAS PubMed.
- K. M. Park, J. Murray and K. Kim, Ultrastable Artificial Binding Pairs as a Supramolecular Latching System: A Next Generation Chemical Tool for Proteomics, Acc. Chem. Res., 2017, 50, 644–646 CrossRef CAS PubMed.
- A. L. Tang, L.-L. Yang, S. Tan, S.-L. Fan, W.-J. Ma, J.-Y. Chen, P. Yang, X. Zhou, L.-W. Liu, P.-Y. Wang and S. Yang, Engineering a novel rhodamine-based fluorescent probe using host-guest interactions for reversible, selective, and sensitive detection of herbicide paraquat, Sens. Actuators, B, 2023, 383, 133556 CrossRef CAS.
- M. G. Strebl, J. Yang, L. Isaacs and J. M. Hooker, Adamantane/Cucurbituril: A Potential Pretargeted Imaging Strategy in Immuno-PET, Mol. Imaging, 2018, 17, 1536012118799838 CrossRef PubMed.
- I. J. J. Vilma, H. A. Courtney, B. Kavita, K. Amritjyot, J. S. David, J. Y. Paul, D. C. Brandon and L. H. Jacob, Pretargeting with Cucurbituril–Adamantane Host–Guest Pair in Xenograft Models, J. Nucl. Med., 2023, 64, 1–7 CrossRef PubMed.
- F.-F. Shen, Y. Chen, X. Dai, H.-Y. Zhang, B. Zhang, Y. Liu and Y. Liu, Purely organic light-harvesting phosphorescence energy transfer by β-cyclodextrin pseudorotaxane for mitochondria targeted imaging, Chem. Sci., 2020, 12, 1851–1857 RSC.
- X. Ma and Y. Zhao, Biomedical Applications of Supramolecular Systems Based on Host–Guest Interactions, Chem. Rev., 2015, 115, 7794–7839 CrossRef CAS PubMed.
- M. J. Webber and R. Langer, Drug delivery by supramolecular design, Chem. Soc. Rev., 2017, 46, 6600–6620 RSC.
- G. Yu and X. Chen, Host-Guest Chemistry in Supramolecular Theranostics, Theranostics, 2019, 9, 3041–3074 CrossRef CAS PubMed.
- L. Wang, L.-l. Li, Y.-s. Fan and H. Wang, Host-Guest Supramolecular Nanosystems for Cancer Diagnostics and Therapeutics, Adv. Mater., 2013, 25, 3888–3898 CrossRef CAS PubMed.
- S. Deshayes and R. Gref, Synthetic and bioinspired cage nanoparticles for drug delivery, Nanomedicine, 2014, 9, 1545–1564 CrossRef CAS PubMed.
- O. A. A. Alabrahim, S. A. Fahmy and H. M. E.-S. Azzazy, Stimuli-Responsive Cucurbit[n]uril-Based Supramolecular Nanocarriers for Delivery of Chemotherapeutics, ACS Appl. Nano Mater., 2023, 6, 3139–3158 CrossRef CAS.
- J. Chen, Q. Huang, Q. Wang, Y. Ding, S. Lu, L.-H. Wang, S. Li and R. Wang, Supramolecular Luminol–AIEgen Nanoparticles for Deep-Tissue-Inflammation Imaging, ACS Appl. Nano Mater., 2022, 5, 5993–6000 CrossRef CAS.
- H.-B. Cheng, Y.-M. Zhang, Y. Liu and J. Yoon, Turn-On Supramolecular Host-Guest Nanosystems as Theranostics for Cancer, Chem, 2019, 5, 553–574 CAS.
- S. Li, Y. Gao, Y. Ding, A. Xu and H. Tan, Supramolecular nano drug delivery systems mediated via host-guest chemistry of cucurbit[n]uril (n = 6 and 7), Chin. Chem. Lett., 2021, 32, 313–318 CrossRef CAS.
- C. M. A. Gangemi, R. Puglisi, A. Pappalardo and G. Trusso Sfrazzetto, Supramolecular complexes for nanomedicine, Bioorg. Med. Chem. Lett., 2018, 28, 3290–3301 CrossRef CAS PubMed.
- A. S. Braegelman and M. J. Webber, Integrating Stimuli-Responsive Properties in Host-Guest Supramolecular Drug Delivery Systems, Theranostics, 2019, 9, 3017–3040 CrossRef CAS PubMed.
- Z. Li, N. Song and Y.-W. Yang, Stimuli-Responsive Drug-Delivery Systems Based on Supramolecular Nanovalves, Matter, 2019, 1, 345–368 CrossRef.
- D. Yang, M. Liu, X. Xiao, Z. Tao and C. Redshaw, Polymeric self-assembled cucurbit[n]urils: Synthesis, structures and applications, Coord. Chem. Rev., 2021, 434, 213733 CrossRef CAS.
- F. Huang, J. Liu and Y. Liu, Engineering living cells with cucurbit[7]uril-based supramolecular polymer chemistry: from cell surface engineering to manipulation of subcellular organelles, Chem. Sci., 2022, 13, 8885–8894 RSC.
- C. Sun, H. Zhang, S. Li, X. Zhang, Q. Cheng, Y. Ding, L.-H. Wang and R. Wang, Polymeric Nanomedicine with “Lego” Surface Allowing Modular Functionalization and Drug Encapsulation, ACS Appl. Mater. Interfaces, 2018, 10, 25090–25098 CrossRef CAS PubMed.
- D. Xia, P. Wang, X. Ji, N. M. Khashab, J. L. Sessler and F. Huang, Functional Supramolecular Polymeric Networks: The Marriage of Covalent Polymers and Macrocycle-Based Host–Guest Interactions, Chem. Rev., 2020, 120, 6070–6123 CrossRef CAS PubMed.
- C. B. Rodell, J. E. Mealy and J. A. Burdick, Supramolecular Guest–Host Interactions for the Preparation of Biomedical Materials, Bioconjugate Chem., 2015, 26, 2279–2289 CrossRef CAS PubMed.
- Y.-W. Yang, Y.-L. Sun and N. Song, Switchable Host–Guest Systems on Surfaces, Acc. Chem. Res., 2014, 47, 1950–1960 CrossRef CAS PubMed.
- Z. Wu, N. Song, R. Menz, B. Pingali, Y.-W. Yang and Y. Zheng, Nanoparticles functionalized with supramolecular host–guest systems for nanomedicine and healthcare, Nanomedicine, 2015, 10, 1493–1514 CrossRef CAS PubMed.
- L. L. Tan, M. Wei, L. Shang and Y. W. Yang, Cucurbiturils-Mediated Noble Metal Nanoparticles for Applications in Sensing, SERS, Theranostics, and Catalysis, Adv. Funct. Mater., 2020, 31, 2007277 CrossRef.
- S. K. Samanta, D. Moncelet, B. Vinciguerra, V. Briken and L. Isaacs, Metal Organic Polyhedra: A Click-and-Clack Approach Toward Targeted Delivery, Helv. Chim. Acta, 2018, 101, e1800057 CrossRef PubMed.
- S. K. Samanta, J. Quigley, B. Vinciguerra, V. Briken and L. Isaacs, Cucurbit[7]uril Enables Multi-Stimuli-Responsive Release from the Self-Assembled Hydrophobic Phase of a Metal Organic Polyhedron, J. Am. Chem. Soc., 2017, 139, 9066–9074 CrossRef CAS PubMed.
- B. Xie, H. Zhao, R. Zhang, Y. Ding, C. Gao, Y. He and R. Wang, Bacteria-mimetic nanomedicine for targeted eradication of intracellular MRSA, J. Controlled Release, 2023, 357, 371–378 CrossRef CAS PubMed.
- C. Kim, S. S. Agasti, Z. Zhu, L. Isaacs and V. M. Rotello, Recognition-mediated activation of therapeutic gold nanoparticles inside living cells, Nat. Chem., 2010, 2, 962–966 CrossRef CAS PubMed.
- G. Y. Tonga, Y. Jeong, B. Duncan, T. Mizuhara, R. Mout, R. Das, S. T. Kim, Y.-C. Yeh, B. Yan, S. Hou and V. M. Rotello, Supramolecular regulation of bioorthogonal catalysis in cells using nanoparticle-embedded transition metal catalysts, Nat. Chem., 2015, 7, 597–603 CrossRef CAS PubMed.
- C. R. Thomas, D. P. Ferris, J.-H. Lee, E. Choi, M. H. Cho, E. S. Kim, J. F. Stoddart, J.-S. Shin, J. Cheon and J. I. Zink, Noninvasive Remote-Controlled Release of Drug Molecules in Vitro Using Magnetic Actuation of Mechanized Nanoparticles, J. Am. Chem. Soc., 2010, 132, 10623–10625 CrossRef CAS PubMed.
- L. Yue, C. Sun, C. H. T. Kwong and R. Wang, Cucurbit[7]uril-functionalized magnetic nanoparticles for imaging-guided cancer therapy, J. Mater. Chem. B, 2020, 8, 2749–2753 RSC.
- B. Xie, H. Zhao, Y.-F. Ding, Z. Wang, C. Gao, S. Li, K. Zhang, S. W. Ip, H. Yu and R. Wang, Supramolecularly Engineered Conjugate of Bacteria and Cell Membrane-Coated Magnetic Nanoparticles for Enhanced Ferroptosis and Immunotherapy of Tumors, Adv. Sci., 2023, 10, 2304407 CrossRef CAS PubMed.
- Y.-F. Ding, Z. Wang, C. H. T. Kwong, Y. Zhao, G. S. P. Mok, H.-Z. Yu and R. Wang, Platelet-mimicking supramolecular nanomedicine with precisely integrated prodrugs for cascade amplification of synergistic chemotherapy, J. Controlled Release, 2023, 360, 82–92 CrossRef CAS PubMed.
- K. M. Park, M. Y. Hur, S. K. Ghosh, D. R. Boraste, S. Kim and K. Kim, Cucurbit[n]uril-based amphiphiles that self-assemble into functional nanomaterials for therapeutics, Chem. Commun., 2019, 55, 10654–10664 RSC.
- K. Nisa, I. A. Lone, W. Arif, P. Singh, S. U. Rehmen and R. Kumar, Applications of supramolecular assemblies in drug delivery and photodynamic therapy, RSC Med. Chem., 2023 10.1039/D3MD00396E.
- M. Zhang, L. Cao and L. Isaacs, Cucurbit[6]uril–cucurbit[7]uril heterodimer promotes controlled self-assembly of supramolecular networks and supramolecular micelles by self-sorting of amphiphilic guests, Chem. Commun., 2014, 50, 14756–14759 RSC.
- P. Zhu, Y. Zhang, P. Lv, X. Liao, Y. Zhao and B. Yang, Functional supramolecular micelles driven by the amphiphilic complex of biotin-acyclic cucurbituril and cannabidiol for cell-targeted drug delivery, Int. J. Pharm., 2022, 625, 122048 CrossRef CAS PubMed.
- X. Huang, T. Chen, N. Mu, H. W. Lam, C. Sun, L. Yue, Q. Cheng, C. Gao, Z. Yuan and R. Wang, Supramolecular micelles as multifunctional theranostic agents for synergistic photodynamic therapy and hypoxia-activated chemotherapy, Acta Biomater., 2021, 131, 483–492 CrossRef CAS PubMed.
- R. P. Narayanan, A. Prasad, A. Buchberger, L. Zou, J. Bernal-Chanchavac, T. MacCulloch, N. E. Fahmi, H. Yan, F. Zhang, M. J. Webber and N. Stephanopoulos, High-Affinity Host–Guest Recognition for Efficient Assembly and Enzymatic Responsiveness of DNA Nanostructures, Small DOI:10.1002/smll.202307585.
- Z. Zhang, X. Wang, X. Wei, S. W. Zheng, B. J. Lenhart, P. Xu, J. Li, J. Pan, H. Albrecht and C. Liu, Multiplex quantitative detection of SARS-CoV-2 specific IgG and IgM antibodies based on DNA-assisted nanopore sensing, Biosens. Bioelectron., 2021, 181, 113134 CrossRef CAS PubMed.
|
This journal is © The Royal Society of Chemistry 2024 |
Click here to see how this site uses Cookies. View our privacy policy here.