DOI:
10.1039/D4MH00464G
(Communication)
Mater. Horiz., 2024,
11, 3662-3694
High-entropy alloy screening for halide perovskites†
Received
18th April 2024
, Accepted 10th May 2024
First published on 10th May 2024
Abstract
As the concept of high-entropy alloying (HEA) extends beyond metals, new materials screening methods are needed. Halide perovskites (HP) are a prime case study because greater stability is needed for photovoltaics applications, and there are 322 experimentally observed HP end-members, which leads to more than 1057 potential alloys. We screen HEAHP by first calculating the configurational entropy of 106 equimolar alloys with experimentally observed end-members. To estimate enthalpy at low computational cost, we turn to the delta-lattice parameter approach, a well-known method for predicting III–V alloy miscibility. To generalize the approach for non-cubic crystals, we introduce the parameter of unit cell volume coefficient of variation (UCV), which does a good job of predicting the experimental HP miscibility data. We use plots of entropy stabilization versus UCV to screen promising alloys and identify 102 HEAHP of interest.
New concepts
We demonstrate the new concept of using unit cell volume coefficient of variation to approximate the enthalpic penalty of a given high-entropy alloy candidate, and use it along with ideal sublattice configurational entropy to map promising high-entropy alloy halide perovskites. While lattice parameter differences have been used for 50 years to predict III–V alloy miscibility, we extend this approach to non-cubic crystals for the first time, and introduce it as a metric for high-entropy alloy materials screening. This new approach is particularly valuable for guiding the search for nonmetallic high-entropy alloys, which is in its infancy for covalent-bonded and semiconducting materials.
|
1. Introduction
Halide perovskites (HP) are a broad class of materials spanning 322 inorganic and hybrid organic–inorganic crystals. The prototypical ABX3 HP has oxidation states of A+, B2+ and X−. The HP's divalent metal (B2+) constituent is octahedrally coordinated to 6 halide ions (X−). These octahedra share corners to form a three-dimensional inorganic framework that surrounds the weakly-bonded A+ constituents in cuboctahedral sites.1,2 Entropy stabilization (ES) is an emerging method3–5 where components are added to a given material until its configurational entropy meaningfully alters its Gibbs free energy.
ES of HP is of interest for their many applications: for electrochemical energy storage materials, ES can enhance ion transport.6,7 For thermoelectrics, ES reduces thermal conductivity.8 For photovoltaics (PV), the enhanced stability of ES is desirable: the photoactive polytypes of the prototypical inorganic HP PV absorber CsPbI3 are metastable below ∼375 K.9 However, the negative impact of ES on charge carrier transport or recombination may limit its use to non-absorbing PV functions such as buffer layers, transport layers or mechanical anchors.10 For other HP applications such as light-emitting diodes (LEDs), lasers, neuromorphics, scintillators, etc., the role of entropy is less clear, but such an extensively inhabited class of crystals make HEAHP of general interest for engineering, such that the boundaries of what is possible, feasible, and useful warrant exploration.
Density functional theory (DFT) is currently being used to screen HEA boride, carbide, and carbonitride ceramics.11 We stress that computationally efficient prescreening methods are needed even for choosing alloys for DFT because HEA have large unit cells, and the 322 experimentally observed HP can combine to form 1057 alloys (considering equimolar compositions with up to 48 end-members). In our first screening, we report the 106 HEAHP consisting entirely of experimentally observed end-members. We then further screen by quantifying their ideal mixing ES and estimate enthalpic penalty using end-member unit cell volume coefficient of variation (UCV), identifying 102 alloys with promising UCV-ES tradeoffs.
2. Results
Metal alloys are the prototypical ES case because they commonly have single site lattice structures. This makes metals behave like ideal solid solutions, so their entropies increase dramatically as components are added: the configurational entropy of a 6-component equimolar mixture of (metal) elements on a single sublattice is −4.5 kJ mol−1 at 300 K.12 ES of oxides has been demonstrated in Mg0.2Co0.2Ni0.2Cu0.2Zn0.2O13 and many other oxides.14 Although MgO, NiO, CuO, and ZnO have different structures and a mean Gibbs energy of formation of −307 kJ mol−1,15 the thermodynamics of Mg0.2Co0.2Ni0.2Cu0.2Zn0.2O were predominated by entropy,13 despite possessing ES of only −2.0 kJ mol−1 at 300 K. By comparison, HP ES should be relatively large and easy to measure. We find 282 inorganic HP that have been experimentally observed16–24 and theory suggests that many more may exist,23–27 so a staggeringly wide combinatorial chemical space can be drawn on to realize this potential. Moreover, weak bonding allows entropy to dominate HP Gibbs energies.28
A given alloy composition change can be net stabilizing if that change's configurational ES outweighs any enthalpic destabilization. In order to screen for promising alloy compositions, we assume each sublattice (A cation, B cation, and X anion) behaves like an ideal solid solution12 to calculate the entropy of mixing (configurational entropy; S/R), as well as the ES term in the Gibbs energy equation at 300 K:
| 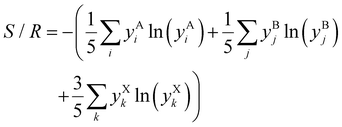 | (1) |
| 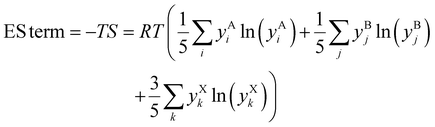 | (2) |
R is the gas constant,
T is temperature (K), and
yAi is the mole fraction of the
ith constituent on the A sublattice in ABX
3. Actual atomic distributions in (metal) HEA have been considered,
29,30 and simple scaling rules have been developed to predict HEA stability for metals.
31–37 Unlike metals, ABX
3 HP have covalent to ionic bonding and 3 distinct lattice sites (A, B, and X), which limits how much they can be stabilized with configurational entropy.
38
In contrast to ES, estimating enthalpy for screening HEAHP is challenging, leading to a tradeoff between accuracy and computational (or experimental) cost. Experimentally screening HEA is most accurate and most expensive. The next most accurate and expensive method combines DFT with the special quasirandom structures approach.39–44 Alternative approaches have been developed to screen alloys45–48 and HEA,49–53 but these are either too computationally expensive,49,51 need too much experimental data,53 or use experiment-free phase diagrams to predict HEA with machine learning, which has limited interpretability.50,52 An approach with even lower computational cost is to estimate mixing enthalpy, which is proportional to the difference between the lattice parameters of a III–V alloy's constitutive end-members.54 Based on the Hume–Rothery rules for metal alloying (minimize atomic radii differences, match crystal structures, keep valency constant, and keep electronegativity constant),55 Foster showed that lattice parameter differences could be used to predict miscibility in III–Vs and II–VIs.56 Foster and Stringfellow used this “delta-lattice parameter” approach to correctly group the miscibility of 9 ternary II–VI56 and 9 quaternary III–V57 alloy systems, respectively, and the method was recently extended to correctly group the miscibility of 18 ternary III–V alloy systems,58 confirming broad accuracy in spite of its low computational cost. To extend the delta-lattice parameter method to non-cubic structures, we draw on Zen's law: there is an empirical linear relation between molar volume and composition of a solid solution.59 (Zen's law simplifies to Vegard's law60 for cubic structures with similar molar volumes.) Therefore, an HP alloy's unit cell volume is its weighted mean (
w):
| 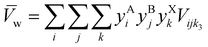 | (3) |
Here
Vijk3 is the unit cell volume of the end-member with the
ith,
jth and
kth constituent on the A, B and X sublattices, respectively. To reduce complexity, we consider only equimolar compositions, which have the greatest ES term. (A semiconductor alloy's density of states can shift its entropic minimum away from the equimolar composition,
61 so other compositions should be considered after the initial screening.) The equimolar unit cell volume's mean, standard deviation, and coefficient of variation are:
| 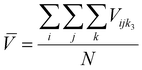 | (4) |
| 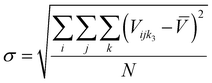 | (5) |
|  | (6) |
Here
![[V with combining macron]](https://www.rsc.org/images/entities/i_char_0056_0304.gif)
is equimolar unit cell volume,
σ is equimolar alloy unit cell volume standard deviation, UCV is equimolar alloy unit cell volume coefficient of variation, and
N is the number of end-members. Atomic radius differences,
62–64 lattice parameter differences,
65,66 and atomic position differences
67 have been previously parameterized to screen metal HEA. We instead parameterize unit cell volume to extend the approach to non-cubic crystals. Although perovskite lattice parameter was previously shown to correlate with ionic radii,
68,69 we use
eqn (6) for enthalpic penalty in HEA for the first time. We confirm agreement with 42 out of 45 room temperature miscibility gap data from III–V (
Fig. 1 and Table S1, ESI
†)
57,58 and II–VI (Table S1, ESI
†)
56 material systems. UCV correlates well with experimental III–V and II–VI mixing enthalpy (Table S1 and Fig. S1, ESI
†), although future work using the elastic modulus or melting temperature are expected to improve the fit.
70 Using the phase boundary for HP in
Fig. 2,
71 22 out of 26 experimental HEAHP data are grouped correctly. Mapping the boundary between single-phase and multiple phase alloys with UCV-ES plots also works for boride, carbide, and carbonitride ceramics: Fig. S2 and Tables S2, S3 (ESI
†) show correct grouping of 56 out of the 64 miscibility data (88% accuracy). Good agreement with such broad experimental data and no fitting parameters suggests the UCV approach has sufficient accuracy despite its low computational cost. UCV allows us to directly compare cubic and hettotype perovskites—the latter have distortions that reduce symmetry, but are more common (
e.g., CsPbI
3's metastable polymorphs).
1,2 There are more reports of single-phase inorganic HEAHP (Table S4, ESI
†)
71–219 and hybrid organic–inorganic HEAHP (Table S5, ESI
†),
220–285 but more investigation into single-phase boundaries is needed to confirm the broadscale applicability of UCV for screening HEAHP.
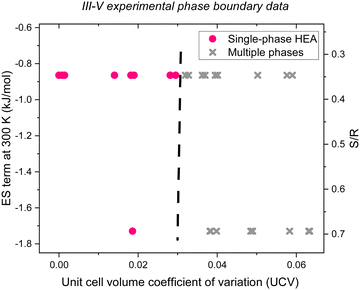 |
| Fig. 1 Experimental III–V single-phase alloy (pink circles) and multiple phase (gray Xs) data,57,58 confirming that plotting the ES term at 300 K (or S/R) as a function of UCV leads to a phase boundary near UCV of 0.03 (black dashed line) which is useful for screening HEA that have not yet been experimentally synthesized. | |
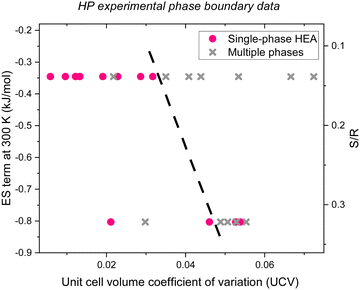 |
| Fig. 2 Experimental HP single-phase alloy (pink circles) and multiple phase (gray Xs) data,71 confirming that plotting the ES term at 300 K (or S/R) as a function of UCV leads to a phase boundary near UCV of 0.04 (black dashed line) which correctly groups 22 of the 26 data. Binary copper alloys are excluded because the synthesis method did not produce phase pure KCuF3.71 | |
Using DFT we calculate mixing enthalpy of 6 HEAHP compositions. To make the computations tractable we approximate a HEA's mixing enthalpy by calculating the energy of 8 distinct configurations of 40-atom unit cells and reference their mean to that alloy's constitutive end-members. The results in Fig. 3 and Table S6 (ESI†) confirm that UCV correlates with DFT mixing enthalpy for HEAHP.
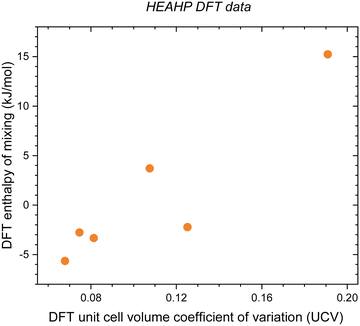 |
| Fig. 3 DFT enthalpy of mixing as a function of UCV from DFT for the HEAHP in Table S6 (ESI†), showing that UCV correlates with DFT mixing enthalpy. | |
It was argued that for thermoelectric devices ES can enhance crystal symmetry to preserve charge carrier transport despite the disordered nuclei that impede phonons and reduce thermal conductance.8,286 In CH3NH3PbI3 phonon lifetimes are shortened by the organic cation's entropy, which may improve charge carrier recombination properties.287 HP's peculiar semiconductor physics have been attributed to dynamic disorder,288 lattice softness and anharmonicity.289
Beyond PV absorbers, ES HP may be useful as oxygen evolution electrocatalysis,71 electrochemical energy storage,86 thermoelectrics,8 light emitting diodes, photodetectors, PV buffers, contacts, solid state radiation detectors, scintillators, fuel cells, lasers, high temperature electronic components, barocaloric materials for use in refrigeration, ferroelectrics, and neuromorphic computers.
The disordered nuclei in ES HP may alter phonons, possibly reducing thermal conductance. Restricted phonons can result in slow cooling of hot charge carriers, similar to what is already observed in HP as a result of light-induced lattice distortions.290,291 On the other hand, local bonding distortions in ES crystals should disrupt electron band energies, creating a distribution of local energy states similar to what was described for ion conductivity through ES materials.7 Thus, bulk 3D carrier transport may suffer, but there may good charge carrier transport along specific crystal directions.
2.1 Mixing on all sublattices
Assuming equimolar compositions on each sublattice (A+, B2+, and X− in ABX3), we calculate the 1
340
752 possible combinations of the 282 experimentally observed inorganic HP with 5 or more components (Table S7, ESI†). The compositions with the greatest ES are in Table 1. HP are mostly composed of halides, so most of the compounds in Table 1 have 4 halide components. The greatest ES, −3.22 kJ mol−1, is for CsB(Br,Cl,F,I)3 with 10 B-site components. The next greatest ES, −3.17 kJ mol−1, is for (Cs,K,Rb)(Ca,Cd,Sn)(Br,Cl,F,I)3, as well as CsB(Br,Cl,F,I)3 with 9 B-site components. (Cs,Rb)(Ca,Cd,Pb,Sn)(Br,Cl,F,I)3 has ES of −3.11 kJ mol−1. CsB(Br,Cl,I)3 with 15 B-site components has ES of −3.00 kJ mol−1.
Table 1 Inorganic HP compositions with the most negative ES term at 300 K whose end-members are all experimentally observed. We omit compositions with an ellipsis (…) that are analogous to the row above them and have the same A- and X-site occupation
Alloy composition |
ES term (kJ mol−1) |
S/R |
CsCa0.1Cd0.1Eu0.1Mg0.1Mn0.1Ni0.1Pb0.1Sn0.1Sr0.1Yb0.1Br0.75Cl0.75F0.75I0.75 |
−3.22 |
1.29 |
Cs0.33K0.33Rb0.33Ca0.33Cd0.33Sn0.33Br0.75Cl0.75F0.75I0.75 |
−3.17 |
1.27 |
CsCa0.11Cd0.11Eu0.11Mg0.11Mn0.11Ni0.11Pb0.11Sn0.11Sr0.11Br0.75Cl0.75F0.75I0.75 |
−3.17 |
1.27 |
… |
|
|
Cs0.5Rb0.5Ca0.25Cd0.25Pb0.25Sn0.25Br0.75Cl0.75F0.75I0.75 |
−3.11 |
1.25 |
CsCa0.13Cd0.13Eu0.13Mg0.13Mn0.13Ni0.13Pb0.13Sn0.13Br0.75Cl0.75F0.75I0.75 |
−3.11 |
1.25 |
… |
|
|
CsCa0.14Cd0.14Eu0.14Mg0.14Mn0.14Ni0.14Pb0.14Br0.75Cl0.75F0.75I0.75 |
−3.05 |
1.22 |
… |
|
|
CsAu0.07Ca0.07Cd0.07Eu0.07Ge0.07Mg0.07Mn0.07Ni0.07Pb0.07Sn0.07Sr0.07Ti0.07Tm0.07V0.07Yb0.07BrClI |
−3.00 |
1.20 |
Cs0.33K0.33Rb0.33Ca0.5Cd0.5Br0.75Cl0.75F0.75I0.75 |
−2.97 |
1.19 |
… |
|
|
Cs0.5K0.5Ca0.33Cd0.33Sn0.33Br0.75Cl0.75F0.75I0.75 |
−2.97 |
1.19 |
Cs0.5Rb0.5Ca0.33Cd0.33Pb0.33Br0.75Cl0.75F0.75I0.75 |
−2.97 |
1.19 |
… |
|
|
Next, we calculate most of the combinations of the 282 inorganic HP with known lattice parameters in Fig. 4, where the ES term at 300 K is plotted as a function of UCV. As Fig. 4(b) and Table 2 show, HP are mostly composed of halides, so the greatest ES comes from X-site mixing. However, X-site mixing drives UCV higher: when all 4 halides are used, the ES term reaches −3.17 kJ mol−1 but has UCV of 0.283 for Cs(Ca,Eu,Mg,Mn,Ni,Pb,Sn,Sr,Yb)(Br,Cl,F,I)3. When only 3 halides are used, an ES term of −2.96 kJ mol−1 is achieved at the much lower UCV of 0.156 for Cs(Au,Ca,Eu,Ge,Mg,Mn,Ni,Pb,Sn,Sr,Ti,Tm,V,Yb)(Br,Cl,I)3. When only 2 halides are used, an ES term of only −2.28 kJ mol−1 is possible, but at UCV of only 0.106 for (Cs,K,Rb,Tl)(Ca,Cd,Mn)(Br,Cl)3, while an ES term of −2.19 kJ mol−1 is reached at a UCV of only 0.073 for (Cs,Rb)(Ca,Ge,Pb,Sn,Sr)(Br,Cl)3. We examine 1-halide compounds in the next section. Other compounds with attractive UCV-ES term tradeoffs are in Fig. 4(b) and Table 3. These specific compositions demonstrate that in general, mixing Br, Cl, and I on the X-site, Cs and Rb on the A-site and Ge, Pb, and Sn on the B-site are all promising. Less obvious constituents include F on the X-site, K and Tl on the A-site and Ca, Cd, Eu, and Sr on the B-site. Former work found the prospect of using hetero-valent substitutes on the B site to be promising.292
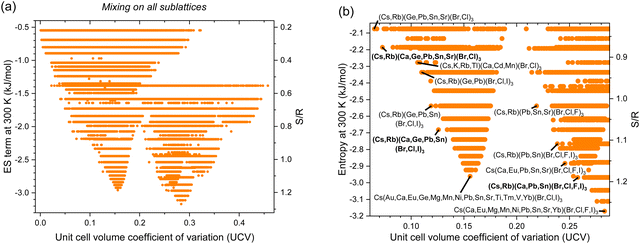 |
| Fig. 4 Entropy stabilization (ES term at 300 K) as a function of enthalpic penalties, or unit cell volume coefficient of variation (UCV), for all equimolar inorganic HP compositions with experimentally observed constitutive end-members with mixing on all sublattices: (a) all data and (b) zoomed in, with promising alloys labeled and in bold. | |
Table 2 Inorganic HP compositions with the greatest ES term at 300 K whose lattice parameters are known and end-members are all experimentally observed with mixing on all sublattices. We omit compositions with an ellipsis (…) that are analogous to the row above them and have the same A- and X-site occupation
Alloy composition |
ES term (kJ mol−1) |
S/R |
UCV |
CsCa0.11Eu0.11Mg0.11Mn0.11Ni0.11Pb0.11Sn0.11Sr0.11Yb0.11Br0.75Cl0.75F0.75I0.75 |
−3.17 |
1.27 |
0.283 |
CsCa0.13Eu0.13Mn0.13Ni0.13Pb0.13Sn0.13Sr0.13Yb0.13Br0.75Cl0.75F0.75I0.75 |
−3.11 |
1.25 |
0.276 |
… |
|
|
|
CsCa0.14Eu0.14Mn0.14Pb0.14Sn0.14Sr0.14Yb0.14Br0.75Cl0.75F0.75I0.75 |
−3.05 |
1.22 |
0.271 |
… |
|
|
|
Cs0.5Rb0.5Ca0.33Pb0.33Sn0.33Br0.75Cl0.75F0.75I0.75 |
−2.97 |
1.19 |
0.258 |
CsCa0.17Eu0.17Pb0.17Sn0.17Sr0.17Yb0.17Br0.75Cl0.75F0.75I0.75 |
−2.97 |
1.19 |
0.264 |
… |
|
|
|
Cs0.33K0.33Rb0.33Ca0.5Sn0.5Br0.75Cl0.75F0.75I0.75 |
−2.97 |
1.19 |
0.273 |
CsCa0.17Eu0.17Mn0.17Sn0.17Sr0.17Yb0.17Br0.75Cl0.75F0.75I0.75 |
−2.97 |
1.19 |
0.276 |
… |
|
|
|
CsAu0.07Ca0.07Eu0.07Ge0.07Mg0.07Mn0.07Ni0.07Pb0.07Sn0.07Sr0.07Ti0.07Tm0.07V0.07Yb0.07BrClI |
−2.96 |
1.19 |
0.156 |
Cs0.5Rb0.5Ca0.14Cd0.14Mn0.14Ni0.14Pb0.14Sn0.14Sr0.14BrClF |
−2.96 |
1.19 |
0.254 |
CsAu0.08Ca0.08Eu0.08Ge0.08Mg0.08Mn0.08Pb0.08Sn0.08Sr0.08Ti0.08Tm0.08V0.08Yb0.08BrClI |
−2.92 |
1.17 |
0.152 |
… |
|
|
|
CsCa0.08Cd0.08Eu0.08Fe0.08Hg0.08Mg0.08Mn0.08Ni0.08Pb0.08Pd0.08Sn0.08Sr0.08Yb0.08BrClF |
−2.92 |
1.17 |
0.268 |
CsAu0.08Ca0.08Eu0.08Ge0.08Mg0.08Mn0.08Pb0.08Sn0.08Sr0.08Tm0.08V0.08Yb0.08BrClI |
−2.88 |
1.16 |
0.149 |
… |
|
|
|
Cs0.5Rb0.5Ca0.17Ge0.17Pb0.17Sn0.17Ti0.17V0.17BrClI |
−2.88 |
1.16 |
0.152 |
CsAu0.08Eu0.08Ge0.08Mg0.08Mn0.08Pb0.08Sn0.08Sr0.08Ti0.08Tm0.08V0.08Yb0.08BrClI |
−2.88 |
1.16 |
0.152 |
… |
|
|
|
Cs0.5Rb0.5Ca0.17Cd0.17Ni0.17Pb0.17Sn0.17Sr0.17BrClF |
−2.88 |
1.16 |
0.246 |
… |
|
|
|
CsCa0.08Cd0.08Eu0.08Fe0.08Hg0.08Mn0.08Ni0.08Pb0.08Pd0.08Sn0.08Sr0.08Yb0.08BrClF |
−2.88 |
1.16 |
0.263 |
… |
|
|
|
Cs0.33K0.33Rb0.33Ca0.25Cd0.25Mn0.25Sn0.25BrClF |
−2.88 |
1.16 |
0.265 |
CsCa0.08Cd0.08Eu0.08Hg0.08Mg0.08Mn0.08Ni0.08Pb0.08Pd0.08Sn0.08Sr0.08Yb0.08BrClF |
−2.88 |
1.16 |
0.266 |
… |
|
|
|
CsCa0.2Eu0.2Pb0.2Sn0.2Sr0.2Br0.75Cl0.75F0.75I0.75 |
−2.88 |
1.16 |
0.254 |
… |
|
|
|
Table 3 Inorganic HP compositions with attractive UCV-ES term at 300 K tradeoffs whose lattice parameters are known and end-members are all experimentally observed with mixing on all sublattices
Alloy composition |
ES term (kJ mol−1) |
S/R |
UCV |
Cs0.5Rb0.5Ca0.33Pb0.33Sn0.33Br0.75Cl0.75F0.75I0.75 |
−2.97 |
1.19 |
0.258 |
CsCa0.2Eu0.2Pb0.2Sn0.2Sr0.2Br0.75Cl0.75F0.75I0.75 |
−2.88 |
1.15 |
0.254 |
Cs0.5Rb0.5Pb0.5Sn0.5Br0.75Cl0.75F0.75I0.75 |
−2.77 |
1.11 |
0.239 |
Cs0.5Rb0.5Ca0.25Ge0.25Pb0.25Sn0.25BrClI |
−2.68 |
1.08 |
0.126 |
Cs0.5Rb0.5Ge0.33Pb0.33Sn0.33BrClI |
−2.54 |
1.02 |
0.119 |
Cs0.5Rb0.5Pb0.33Sn0.33Sr0.33BrClF |
−2.54 |
1.02 |
0.219 |
Cs0.5Rb0.5Ge0.5Pb0.5BrClI |
−2.34 |
0.94 |
0.112 |
Cs0.25K0.25Rb0.25Tl0.25Ca0.33Cd0.33Mn0.33Br1.5Cl1.5 |
−2.28 |
0.91 |
0.106 |
Cs0.5Rb0.5Ca0.2Ge0.2Pb0.2Sn0.2Sr0.2Br1.5Cl1.5 |
−2.19 |
0.88 |
0.073 |
Cs0.5Rb0.5Ge0.25Pb0.25Sn0.25Sr0.25Br1.5Cl1.5 |
−2.07 |
0.83 |
0.065 |
2.2 Mixing on only A and B sublattices (ordered valence band)
HP valence band maximum is dominated by (X) halide with minor B cation contributions, while the conduction band minimum is mostly determined by the B cation with small X contributions.293 Therefore, to preserve order in the valence band and keep valence band energy constant to facilitate hole transport, A- and B-site cations can both be alloyed while the halide is kept pure (1 component on the X sublattice). In this case, the greatest ES term is only −1.68 kJ mol−1 for CsBCl3 with 29 B-site components (Fig. 5). Other noteworthy compositions are shown in Fig. 5(b) and Table 4. As discussed in the previous section, less halide mixing translates to less ES but also lower UCV. Halide segregation is a known issue in HP294 that could prevent the use of mixing on the X-site for ES. If that is a limitation, then the compounds in this section can still be used to achieve moderate ES at low enthalpic penalties (low UCV), all while maintaining an ordered valence band valuable for hole transport.
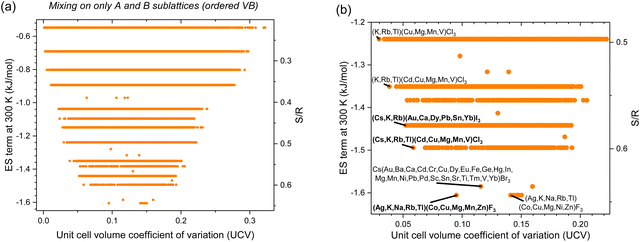 |
| Fig. 5 Entropy stabilization (ES term at 300 K) as a function of enthalpic penalties, or unit cell volume coefficient of variation (UCV), for equimolar inorganic HP compositions with experimentally observed constitutive end-members with mixing on only A and B sublattices (ordered valence band): (a) all data and (b) zoomed in, with promising alloys labeled and in bold. | |
Table 4 Inorganic HP compositions with attractive UCV-ES term at 300 K tradeoffs whose lattice parameters are known and end-members are all experimentally observed with mixing on only A and B sublattices (ordered valence band)
Alloy composition |
ES term (kJ mol−1) |
S/R |
UCV |
Ag0.2K0.2Na0.2Rb0.2Tl0.2Co0.2Cu0.2Mg0.2Mn0.2Zn0.2F3 |
−1.61 |
0.64 |
0.095 |
Ag0.2K0.2Na0.2Rb0.2Tl0.2Co0.2Cu0.2Mg0.2Ni0.2Zn0.2F3 |
−1.61 |
0.64 |
0.141 |
CsAu0.04Ba0.04Ca0.04Cd0.04Cr0.04Cu0.04Dy0.04Eu0.04Fe0.04Ge0.04Hg0.04In0.04Mg0.04Mn0.04 |
|
|
|
Ni0.04Pb0.04Pd0.04Sc0.04Sn0.04Sr0.04Ti0.04Tm0.04V0.04Yb0.04Br3 |
−1.59 |
0.64 |
0.116 |
Cs0.25K0.25Rb0.25Tl0.25Cd0.2Cu0.2Mg0.2Mn0.2V0.2Cl3 |
−1.49 |
0.60 |
0.059 |
Cs0.33K0.33Rb0.33Au0.17Ca0.17Dy0.17Pb0.17Sn0.17Yb0.17I3 |
−1.44 |
0.58 |
0.052 |
K0.33Rb0.33Tl0.33Cd0.2Cu0.2Mg0.2Mn0.2V0.2Cl3 |
−1.35 |
0.54 |
0.038 |
K0.33Rb0.33Tl0.33Cu0.25Mg0.25Mn0.25V0.25Cl3 |
−1.24 |
0.50 |
0.030 |
2.3 Mixing on only A and X sublattices (ordered conduction band)
To preserve order in the conduction band and conduction band energy alignment to facilitate electron transport, mixing on the A- and X-sites can be used. In this case, the greatest ES term is −2.77 kJ mol−1 for (Cs,K,Rb,Tl)Cd(Br,Cl,F,I)3. Other noteworthy compositions are shown in Fig. 6(b) and Table 5. We note that 3 of the compounds are entirely composed of end-members whose experimental band gaps are known. The compounds’ band gaps are estimated by averaging end-member values: 1.95 eV for CsSnBrClI, 2.31 eV for CsPbBrClI, and 2.48 eV for CsGeBrClI.
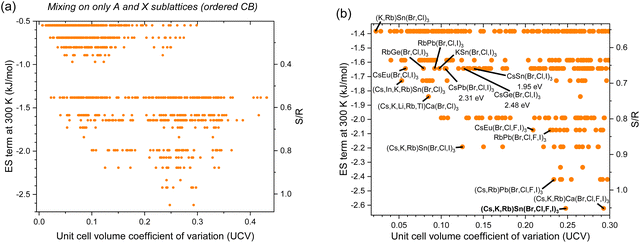 |
| Fig. 6 Entropy stabilization (ES term at 300 K) as a function of enthalpic penalties, or unit cell volume coefficient of variation (UCV), for equimolar inorganic HP compositions with experimentally observed constitutive end-members with mixing on only A and X sublattices (ordered conduction band): (a) all data and (b) zoomed in, with promising alloys labeled and in bold. | |
Table 5 Inorganic HP compositions with attractive UCV-ES term at 300 K tradeoffs whose lattice parameters are known and end-members are all experimentally observed with mixing on only A and X sublattices (ordered conduction band)
Alloy composition |
ES term (kJ mol−1) |
S/R |
UCV |
Cs0.33K0.33Rb0.33SnBr0.75Cl0.75F0.75I0.75 |
−2.62 |
1.05 |
0.248 |
Cs0.33K0.33Rb0.33CaBr0.75Cl0.75F0.75I0.75 |
−2.62 |
1.05 |
0.293 |
Cs0.5Rb0.5PbBr0.75Cl0.75F0.75I0.75 |
−2.42 |
0.97 |
0.234 |
K0.5Rb0.5SnBr0.75Cl0.75F0.75I0.75 |
−2.42 |
0.97 |
0.242 |
Cs0.33K0.33Rb0.33SnBrClI |
−2.19 |
0.88 |
0.125 |
CsEuBr0.75Cl0.75F0.75I0.75 |
−2.07 |
0.83 |
0.209 |
RbPbBr0.75Cl0.75F0.75I0.75 |
−2.07 |
0.83 |
0.230 |
RbSnBr0.75Cl0.75F0.75I0.75 |
−2.07 |
0.83 |
0.232 |
CsPbBr0.75Cl0.75F0.75I0.75 |
−2.07 |
0.83 |
0.236 |
Cs0.2K0.2Li0.2Rb0.2Tl0.2CaBr1.5Cl1.5 |
−1.84 |
0.74 |
0.085 |
Cs0.25In0.25K0.25Rb0.25SnBr1.5Cl1.5 |
−1.73 |
0.69 |
0.053 |
CsEuBrClI |
−1.64 |
0.66 |
0.058 |
RbGeBrClI |
−1.64 |
0.66 |
0.079 |
RbPbBrClI |
−1.64 |
0.66 |
0.092 |
KSnBrClI |
−1.64 |
0.66 |
0.098 |
CsPbBrClI (2.31 eV) |
−1.64 |
0.66 |
0.105 |
CsGeBrClI (2.48 eV) |
−1.64 |
0.66 |
0.127 |
CsSnBrClI (1.95 eV) |
−1.64 |
0.66 |
0.138 |
K0.5Rb0.5SnBr1.5Cl1.5 |
−1.38 |
0.55 |
0.023 |
2.4 Mixing on only A sublattice (ordered valence and conduction bands)
To preserve order in the valence and conduction bands and prevent changes in the valence and conduction band energy as well as band gap, alloying on only the A-site should be used. In this case, the greatest ES term is only −0.97 kJ mol−1 with UCV of 0.063 for (Cs,In,K,Li,Na,Rb,Tl)CaBr3. Other compositions of interest are shown in Fig. 7(b) and Table 6.
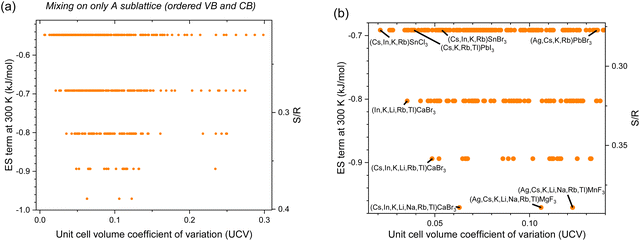 |
| Fig. 7 Entropy stabilization (ES term at 300 K) as a function of enthalpic penalties, or unit cell volume coefficient of variation (UCV), for equimolar inorganic HP compositions with experimentally observed constitutive end-members with mixing on only the A sublattice (ordered valence and conduction bands): (a) all data and (b) zoomed in, with promising alloys labeled and in bold. | |
Table 6 Inorganic HP compositions with attractive UCV-ES term at 300 K tradeoffs whose lattice parameters are known and end-members are all experimentally observed with mixing on only the A sublattice (ordered valence and conduction bands)
Alloy composition |
ES term (kJ mol−1) |
S/R |
UCV |
Cs0.14In0.14K0.14Li0.14Na0.14Rb0.14Tl0.14CaBr3 |
−0.97 |
0.39 |
0.063 |
Ag0.14Cs0.14K0.14Li0.14Na0.14Rb0.14Tl0.14MgF3 |
−0.97 |
0.39 |
0.106 |
Ag0.14Cs0.14K0.14Li0.14Na0.14Rb0.14Tl0.14MnF3 |
−0.97 |
0.39 |
0.123 |
Cs0.17In0.17K0.17Li0.17Rb0.17Tl0.17CaBr3 |
−0.89 |
0.36 |
0.049 |
In0.2K0.2Li0.2Rb0.2Tl0.2CaBr3 |
−0.80 |
0.32 |
0.035 |
Cs0.25In0.25K0.25Rb0.25SnCl3 |
−0.69 |
0.28 |
0.021 |
Cs0.25K0.25Rb0.25Tl0.25PbI3 |
−0.69 |
0.28 |
0.039 |
Cs0.25In0.25K0.25Rb0.25SnBr3 |
−0.69 |
0.28 |
0.053 |
Ag0.25Cs0.25K0.25Rb0.25PbBr3 |
−0.69 |
0.28 |
0.135 |
A-site and X-site segregation are both known issues in HP295 that could prevent the use of mixing on the A- and X-sites for ES. If those are limitations, then the compounds in this section can still be used to achieve weak ES at low enthalpic penalties (low UCV), all while maintaining ordered valence and conduction bands valuable for both hole and electron transport.
2.5 Organic A-site components (hybrid organic–inorganic HP)
The previous sections have only considered inorganic compounds, but there are at least 22 organic cations that can substitute on the A-site: methylammonium (MA; CH3NH3), formamidinium (FA; HC(NH2)2), guanidinium (GA; C(NH2)3), dimethylammonium (DMA; (CH3)2NH2), ethylammonium (EA; CH3CH2NH3), acetamidinium (ACA; CH3C(NH2)2), ammonium (NH4), hydrazinium (HA; N2H5), azetidinium (AZ; C3H6NH2), imidazolium (IM; C3N2H5), trimethylammonium (TMA; (CH3)3NH), tetramethylammonium (TEMA; (CH3)4N), arsonium, methylarsonium, methylphosphonium, aziridine, hydroxylammonium, phosphonium, antimonium, PF4, NH2CHPH2, and NH2CHAsH2.296 Therefore, hybrid organic–inorganic halide perovskites have an even larger chemical space that can be tapped for ES than the pure inorganics.
Previous work found ES in (Cs,FA)PbI3,297 (FA,GA)PbBr3,298 (Cs,FA,MA)PbI3,299 (Cs,FA,MA)Pb(Br,I)3,221,300 and (Cs,FA,MA,Rb)PbI3,220,222 and ES was also recently demonstrated in double HP28,301 and “hollow” HP.302 Long anneals of CH3NH3PbI3 were argued to maximize configurational entropy of the organic cation, which was found to stabilize the cubic polytype and improve electrical properties.303 Entropy stabilization of HP nanocrystals was recently demonstrated: Pb was substituted for Mg, Zn, and Cd in CH3NH3PbBr3 to enhance stability, while narrow band emission was retained.304 Unlike former reports,297,298 here we consider the maximum feasible configurational ES, which restricts our focus to alloys with a minimum of 5 components.
Mixing the 40 organic and 282 inorganic HP end-members, we find 14
270 hybrid organic–inorganic HEAHPs consisting of 5 or more experimentally observed end-members (Fig. 8 and Table S8, ESI†). Attractive UCV-ES term tradeoffs are in Table 7. In general, smaller ES are possible at a given UCV, relative to the inorganic HEAHPs in the previous sections. Table 7 mostly consists of well-studied alloys based on Cs, MA, and FA, but the less-studied Rb and K are also present. For PV-related Br, Cl, and I systems, the B-site constituents are Ge, Sn and Pb. The F systems are of interest for electrochemical applications and have smaller unit cells, so NH4 and Na are allowed, Cd, Fe, and Mn are prevalent while Tl, Co, Cu Fe, Mg, Ni and Zn are possible. (Cs,K,NH4,Rb,Tl)(Cd,Fe,Mn)(Cl,F)3 and (K,NH4,Rb,Tl)(Cd,Co,Cr,Cu,Fe,Mg,Mn,Ni,Sn,Zn)F3 have ES terms at 300 K of −2.39 and −1.84 kJ mol−1, respectively. While inorganic HEAHP have more negative ES terms as temperature increases, the organic components’ volatility may limit this effect for organic HEAHP.
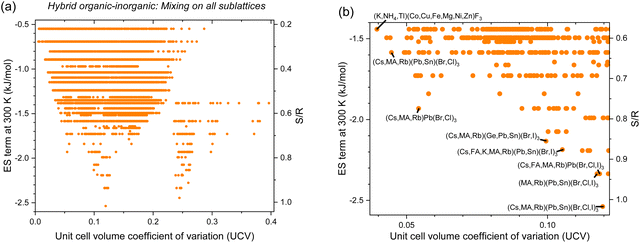 |
| Fig. 8 Entropy stabilization (ES term at 300 K) as a function of enthalpic penalties, or unit cell volume coefficient of variation (UCV), for all equimolar hybrid organic–inorganic HP compositions with experimentally observed constitutive end-members with mixing on all sublattices: (a) all data and (b) zoomed in, with promising alloys labeled and in bold. | |
Table 7 Hybrid organic–inorganic HP compositions with the greatest ES term at 300 K whose lattice parameters are known and end-members are all experimentally observed with mixing on all sublattices. Calculated band gaps are included along with the maximum experimental band gap bowing (the difference between the linearly-interpolated-band gap and the actual band gap) and references
Alloy composition |
ES term (kJ mol−1) |
S/R |
UCV |
Band gap (eV) |
Exp. bowing (eV) |
Cs0.33MA0.33Rb0.33Pb0.5Sn0.5BrClI |
−2.54 |
1.02 |
0.120 |
— |
— |
Cs0.2K0.2(NH4)0.2Rb0.2Tl0.2CdBrClF |
−2.45 |
0.98 |
0.245 |
— |
— |
MA0.5Rb0.5Pb0.5Sn0.5BrClI |
−2.34 |
0.94 |
0.118 |
— |
— |
Cs0.25FA0.25MA0.25Rb0.25PbBrClI |
−2.34 |
0.94 |
0.119 |
— |
— |
Cs0.5MA0.5Pb0.5Sn0.5BrClI |
−2.34 |
0.94 |
0.122 |
2.24 |
MA(Pb,Sn)(Br,I)3 ≤ 0.11;243 |
(Cs,MA)(Pb,Sn)I3 ≤ 0.11;243 |
(Cs,FA)PbI3 ≤ 0.02;249 |
MAPb(Br,Cl)3 ≤ 0.17242 |
Cs0.25K0.25MA0.25Rb0.25SnBrClI |
−2.34 |
0.94 |
0.124 |
— |
— |
… |
|
|
|
|
|
Cs0.25K0.25(NH4)0.25Rb0.25Cd0.33Fe0.33Mn0.33Cl1.5F1.5 |
−2.28 |
0.91 |
0.260 |
— |
— |
Cs0.33FA0.33Rb0.33PbBrClI |
−2.19 |
0.88 |
0.112 |
2.31 |
(Cs,Rb)PbBr3 = 0;125 |
(Cs,Rb)PbCl3 = 0125 |
… |
|
|
|
|
|
Cs0.33FA0.33MA0.33PbBrClI |
−2.19 |
0.88 |
0.122 |
2.28 |
(FA,MA)Pb(Br,I)3 ≤ 0.10;230,243,245,261 |
MAPb(Br,Cl)3 ≤ 0.17242 |
… |
|
|
|
|
|
Cs0.2FA0.2K0.2MA0.2Rb0.2Pb0.5Sn0.5Br1.5I1.5 |
−2.19 |
0.88 |
0.105 |
— |
— |
… |
|
|
|
|
|
Cs0.33MA0.33Rb0.33Ge0.33Pb0.33Sn0.33Br1.5I1.5 |
−2.13 |
0.86 |
0.099 |
— |
— |
… |
|
|
|
|
|
Cs0.5FA0.5PbBrClI |
−1.99 |
0.80 |
0.115 |
2.27 |
— |
… |
|
|
|
|
|
MAPb0.5Sn0.5BrClI |
−1.99 |
0.80 |
0.120 |
2.34 |
MA(Pb,Sn)(Br,I)3 ≤ 0.11;243 |
MAPb(Br,Cl)3 ≤ 0.17242 |
… |
|
|
|
|
|
Cs0.5MA0.5PbBrClI |
−1.99 |
0.80 |
0.121 |
2.31 |
MAPb(Br,I)3 ≤ 0.07;243,245 |
MAPb(Br,Cl)3 ≤ 0.17242 |
Cs0.5MA0.5SnBrClI |
−1.99 |
0.80 |
0.122 |
2.16 |
MASn(Br,I)3 ≤ 0.03243 |
FA0.5MA0.5PbBrClI |
−1.99 |
0.80 |
0.127 |
2.27 |
MAPb(Br,I)3 ≤ 0.07;243,245 |
MAPb(Br,Cl)3 ≤ 0.17;242 |
(FA,MA)PbI3 ≤ 0.02261 |
… |
|
|
|
|
|
Cs0.33MA0.33Rb0.33Pb0.5Sn0.5Br1.5Cl1.5 |
−1.93 |
0.77 |
0.054 |
— |
— |
… |
|
|
|
|
|
K0.25Na0.25(NH4)0.25Tl0.25Co0.14Cu0.14Fe0.14Mg0.14Mn0.14Ni0.14Zn0.14F3 |
−1.66 |
0.67 |
0.082 |
— |
— |
… |
|
|
|
|
|
Cs0.33MA0.33Rb0.33PbBr1.5Cl1.5 |
−1.59 |
0.64 |
0.045 |
— |
— |
2.6 Non-equimolar compositions
For non-equimolar compositions, weighted standard deviation (σw) and weighted coefficient of variation (UCVw) are: | 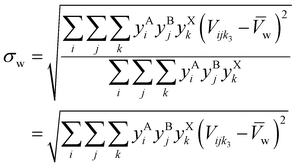 | (7) |
| 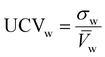 | (8) |
The boundary between single-phase and multiple phase compositions has been mapped experimentally for MAPb(Br,Cl,I)3.223 We calculate ES term at 300 K and UCVw for MAPb(Br,Cl,I)3 in Fig. 8(a) and (b). While UCVw predicts the general shape of the data, multiplying UCVw by a constant (C) and adding it to ES term accurately predicts 52 out of the 56 data (93%; Fig. 9(c)). ES′ combined effect on Gibbs energy (GES) is: | 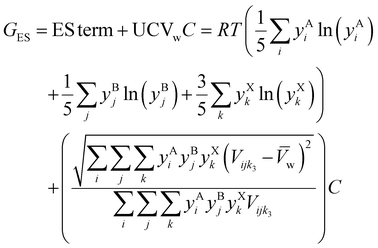 | (9) |
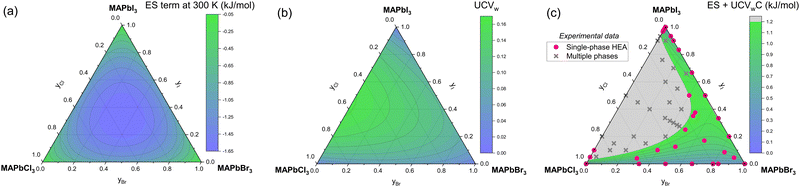 |
| Fig. 9 (a) ES term at 300 K contours, (b) UCVw contours, and (c) ES + UCVwC contours for MAPb(Br,Cl,I)3. Experimental HP single-phase alloy (pink circles) and multiple phase (gray Xs) data are in (c),223 confirming that C = 23 kJ mol−1 leads to a phase boundary at GES = 1.22 kJ mol−1 that correctly groups 52 of the 56 data (93%). | |
This new equation is Calphad with crystal structure inputs. Empirically fitting C to the MAPb(Br,Cl,I)3 data yields C of 23 kJ mol−1. On the other hand, a C value of 40 kJ mol−1 matches the experimental data for CsPb(Br,Cl,I)3 (Fig. S3, ESI†), suggesting enthalpic penalty plays more of a role in the latter. Altogether we accurately predict 75 out of the 83 ternary data (91%), showing that UCV-ES maps can rank alloys with different constituents and different compositions.
2.7 Known experimental band gaps
Of the 282 experimentally observed inorganic HP compounds, we find experimental band gaps for 19. Of the 1
340
752 alloy compositions we consider, 73 are entirely composed of end-members whose experimental band gaps are known. Fig. S4 and Table S9 (ESI†) show that they all contain Cs, most have Ge, Pb or Sn, and most band gaps are wider than 2 eV. Bowing can shift these band gap values, and experimental bowing data is in Table 7 and Fig. S9 (ESI†).
2.8 Overall accuracy
Finally, we note the high accuracy of UCV separating experimental miscibility data across crystal systems with a spectrum of bonding character: from weak ionic HP (89% of 109 data) to weak covalent II–VIs (83% of 18 data), covalent III–Vs (100% of 27 data), and finally to strong covalent boride, carbide, and carbonitride ceramics (88% of 64 data). Overall accuracy for the 218 data is 89.4%. For comparison, the accuracy of Materials Project DFT unit cell volumes relative to experiment is 92.6%.305 There are exceptions to UCV predicting miscibility: KCo0.2Fe0.2Mg0.2Ni0.2Zn0.2F3 in Fig. 2, Hf0.2Mo0.2Nb0.2Ta0.2W0.2C0.5N0.5 in Fig. S2 (ESI†), and CsPb0.5Zn0.5Cl3, CsPb0.5Zn0.5Br3, and CsPb0.5Zn0.5I3 in Table S4 (ESI†). These exceptions show that crystal structure and Gibbs energy are more nuanced than a single parameter can describe, but UCV captures 89% of HEA mixing behavior.
3. Conclusions
We take a low computational cost approach to screening HEA and employ it to identify promising inorganic and hybrid organic–inorganic HEAHP. Drawing from the pool of 322 experimentally observed HP, we compute configurational entropy stabilization (ES) of equimolar HEA. Starting with the delta-lattice parameter approach for predicting III–V miscibility, we introduce the more generally applicable unit cell volume coefficient of variation (UCV) to estimate enthalpic penalty of HEA. UCV predicts the existing experimental III–V, II–VI, boride, carbide, carbonitride, and HP data well. We screen the 1057 possible HEAHP to report the 106 alloys consisting entirely of experimentally observed end-members, then identify 102 HEAHP with promising UCV-ES tradeoffs. These results can serve as a first screen for guiding more costly calculations and experiments.
4. Methods
Throughout the literature, the boundary between what is considered perovskite and not considered perovskite is ambiguous.1,2 We limit our search to the 282 inorganic and 40 organic ABX3 compounds that have been experimentally observed and previously labeled as “perovskites” (Tables S1018–20,27,111,112,115,123,179,306–539 and S11 (ESI†),315,466,524,526,540–558 respectively). We exclude the 90 inorganic HP that have been proposed but not synthesized (Table S12, ESI†).23,24,307,430 In order to use a self-consistent database, where possible we use lattice parameters from the Materials Project559 for the Pnma orthorhombic perovskite structure (space group #62; 20 constituents per unit cell; 4 formula units per unit cell). Many HP have different structural symmetry (e.g., Pm
m cubic with 5 constituents per unit cell or 1 formula unit per unit cell), and in such cases we consider the unit cell volume for which the number of atoms would be 20 (for Pm
m the unit cell volume is multiplied by 4). Materials Project559 unit cell volumes are well correlated with Inorganic Crystal Structure Database (ICSD) values.305,560 We find lattice parameters for 265 of the inorganic HP. We also tabulate experimental band gaps where available. We first consider all possible equimolar alloys with 3 end-members, then check if a possible HEA consists entirely of experimentally observed end-members. If it does then we tabulate it after calculating the ES term at 300 K, UCV (if available), and mean band gap (if available). We provide example code with extensive comments as an ESI† file (Mathematica notebook). We execute the notebook on a personal computer using a built-in parallel do statement and consider alloys with up to 48 end-members. There are 1057 ways to combine 48 of the 322 end-members (322!/(48! (322-48)!) ∼ 1057), so to avoid checking every combination of the 9 A-site, 32 B-site and 4 X-site inorganic constituents and 10 additional A-site organic constituents, we examine the simpler alloy systems first to determine which complex alloys can possibly be built from the existing results. In other words, the computation can be simplified by only checking a higher order system's potential constituents if their constitutive lower order systems exist. Eventually, the number of constituents on a sublattice reaches a maximum, beyond which no more can be added without including an end-member that has not been experimentally observed, and then the search can stop. Here we examine only HP, but our approach has value for the closely related double perovskites301 and the 76 experimentally observed chalcogenide (sulfur, selenium, and tellurium) perovskites,24 although chalcogenide perovskites are less developed than the halides.561
DFT calculations: in order to verify that compositions with small (large) UCV are stable (unstable), we carry out geometric relaxations for the selected compositions in Table S6 (ESI†). We carry out these DFT calculations using the Vienna Ab initio Software Package (VASP, version 5.4),562,563 in the framework of the generalized gradient approximation (GGA), with the Perdew, Burke and Ernzerhof (PBE) functional.564 We use a plane wave energy cutoff of 400 eV and the following Brillouin zone grids, depending on the size of the supercell: 2 × 2 × 2 k-point grids (8 irreducible k points) for 2 × 2 × 2 supercells (40 atoms), 1 × 2 × 2 k-point grids for 4 × 2 × 2 supercells (80 atoms), and 1 × 1 × 1 k-point grids for 3 × 3 × 3 supercells (135 atoms). All the relaxations are started from ideal cubic perovskite structures and are fully relaxed (unit cell shape and atomic coordinates) using the conjugate-gradient algorithm until residual forces become smaller than 0.004 eV Å−1. The electronic relaxations at each ionic step are stopped when the energy difference between consecutive self-consistency iterations reaches 10−7 eV. In order to improve convergence to equilibrium, we scale the displacement steps by 0.1 and declare 180 bands (20 more than the default). To assess mixing effects of various ions on the A-, B-, and X-sites, we include 8 distinct configurations for each composition, and average the final energy and final cell volume across these configurations. The DFT mixing enthalpy is the mean DFT energy of the 8 HEA configurations referenced to the DFT energy of the HEA's end-members:
| 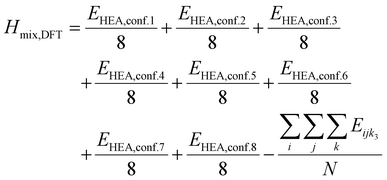 | (10) |
Conflicts of interest
There are no conflicts to declare.
Acknowledgements
This work was authored by the National Renewable Energy Laboratory, operated by Alliance for Sustainable Energy, LLC, for the U.S. Department of Energy (DOE) under contract no. DE-AC36-08GO28308. The views expressed in the article do not necessarily represent the views of the DOE or the U.S. Government. The U.S. Government retains and the publisher, by accepting the article for publication, acknowledges that the U.S. Government retains a nonexclusive, paid-up, irrevocable, worldwide license to publish or reproduce the published form of this work, or allow others to do so, for U.S. Government purposes. Funding was provided by the U.S. Army Research Office through grant no. W911NF2210273 (Dr Hugh C. DeLong).
References
- Q. A. Akkerman and L. Manna, What Defines a Halide Perovskite, ACS Energy Lett., 2020, 5(2), 604–610, DOI:10.1021/acsenergylett.0c00039.
- R. H. Mitchell, M. D. Welch and A. R. Chakhmouradian, Nomenclature of the perovskite supergroup: A hierarchical system of classification based on crystal structure and composition, Mineral. Mag., 2017, 81(3), 411–461, DOI:10.1180/minmag.2016.080.156.
- V. Dusastre, Gaining from mixing, Nat. Mater., 2023, 22(4), 401, DOI:10.1038/s41563-023-01530-3.
- Y. Wang, M. J. Robson, A. Manzotti and F. Ciucci, High-entropy perovskites materials for next-generation energy applications, Joule, 2023, 7(5), 848–854, DOI:10.1016/j.joule.2023.03.020.
- Z. Du, C. Wu, Y. Chen, Z. Cao, R. Hu, Y. Zhang, J. Gu, Y. Cui, H. Chen, Y. Shi, J. Shang, B. Li and S. Yang, High-Entropy Atomic Layers of Transition-Metal Carbides (MXenes), Adv. Mater., 2021, 33(39), 2101473, DOI:10.1002/adma.202101473.
- S. Schweidler, M. Botros, F. Strauss, Q. Wang, Y. Ma, L. Velasco, G. Cadilha Marques, A. Sarkar, C. Kübel, H. Hahn, J. Aghassi-Hagmann, T. Brezesinski and B. Breitung, High-entropy materials for energy and electronic applications, Nat. Rev. Mater., 2024, 9, 266–281, DOI:10.1038/s41578-024-00654-5.
- Y. Zeng, B. Ouyang, J. Liu, Y.-W. Byeon, Z. Cai, L. J. Miara, Y. Wang and G. Ceder, High-entropy mechanism to boost ionic conductivity, Science, 2022, 378(6626), 1320–1324, DOI:10.1126/science.abq1346.
- B. Jiang, Y. Yu, J. Cui, X. Liu, L. Xie, J. Liao, Q. Zhang, Y. Huang, S. Ning, B. Jia, B. Zhu, S. Bai, L. Chen, S. J. Pennycook and J. He, High-entropy-stabilized chalcogenides with high thermoelectric performance, Science, 2021, 371(6531), 830–834, DOI:10.1126/science.abe1292.
- H.-Y. Gu, W.-J. Yin and X.-G. Gong, Significant phonon anharmonicity drives phase transitions in CsPbI3, Appl. Phys. Lett., 2021, 119(19), 191101, DOI:10.1063/5.0072367.
- J. A. Steele, T. Braeckevelt, V. Prakasam, G. Degutis, H. Yuan, H. Jin, E. Solano, P. Puech, S. Basak, M. I. Pintor-Monroy, H. Van Gorp, G. Fleury, R. X. Yang, Z. Lin, H. Huang, E. Debroye, D. Chernyshov, B. Chen, M. Wei, Y. Hou, R. Gehlhaar, J. Genoe, S. De Feyter, S. M. J. Rogge, A. Walsh, E. H. Sargent, P. Yang, J. Hofkens, V. Van Speybroeck and M. B. J. Roeffaers, An embedded interfacial network stabilizes inorganic CsPbI3 perovskite thin films, Nat. Commun., 2022, 13(1), 7513, DOI:10.1038/s41467-022-35255-9.
- S. Divilov, H. Eckert, D. Hicks, C. Oses, C. Toher, R. Friedrich, M. Esters, M. J. Mehl, A. C. Zettel, Y. Lederer, E. Zurek, J.-P. Maria, D. W. Brenner, X. Campilongo, S. Filipović, W. G. Fahrenholtz, C. J. Ryan, C. M. DeSalle, R. J. Crealese, D. E. Wolfe, A. Calzolari and S. Curtarolo, Disordered enthalpy–entropy descriptor for high-entropy ceramics discovery, Nature, 2024, 625(7993), 66–73, DOI:10.1038/s41586-023-06786-y.
-
H. L. Lukas, S. G. Fries and B. Sundman, Computational thermodynamics: the Calphad method, Cambridge University Press, Cambridge, 2007, p. 324 Search PubMed.
- C. M. Rost, E. Sachet, T. Borman, A. Moballegh, E. C. Dickey, D. Hou, J. L. Jones, S. Curtarolo and J.-P. Maria, Entropy-stabilized oxides, Nat. Commun., 2015, 6(1), 8485, DOI:10.1038/ncomms9485.
- Y. Wang, J. Liu, Y. Song, J. Yu, Y. Tian, M. J. Robson, J. Wang, Z. Zhang, X. Lin, G. Zhou, Z. Wang, L. Shen, H. Zhao, S. Grasso and F. Ciucci, High-Entropy Perovskites for Energy Conversion and Storage: Design, Synthesis, and Potential Applications, Small Methods, 2023, 7(4), 2201138, DOI:10.1002/smtd.202201138.
-
P. Villars, Thermodynamic Properties of Compounds: Datasheet from Landolt-Börnstein – Group IV Physical Chemistry, Pure Substances, Springer-Verlag, Berlin, Heidelberg, 2022, vol. 19A3 DOI:10.1007/10551582_9.
- C. Li, X. Lu, W. Ding, L. Feng, Y. Gao and Z. Guo, Formability of ABX3 (X = F, Cl, Br, I) halide perovskites, Acta Crystallogr., Sect. B: Struct. Sci., 2008, 64(6), 702–707, DOI:10.1107/S0108768108032734.
- W. Travis, E. N. K. Glover, H. Bronstein, D. O. Scanlon and R. G. Palgrave, On the application of the tolerance factor to inorganic and hybrid halide perovskites: a revised system, Chem. Sci., 2016, 7(7), 4548–4556, 10.1039/C5SC04845A.
- Z.-G. Lin, L.-C. Tang and C.-P. Chou, Study on mid-IR NLO crystals CsGe(BrxCl1−x)3, Opt. Mater., 2008, 31(1), 28–34, DOI:10.1016/j.optmat.2008.01.004.
- T. Krishnamoorthy, H. Ding, C. Yan, W. L. Leong, T. Baikie, Z. Zhang, M. Sherburne, S. Li, M. Asta, N. Mathews and S. G. Mhaisalkar, Lead-free germanium iodide perovskite materials for photovoltaic applications, J. Mater. Chem. A, 2015, 3(47), 23829–23832, 10.1039/C5TA05741H.
- L. Debbichi, S. Lee, H. Cho, A. M. Rappe, K.-H. Hong, M. S. Jang and H. Kim, Mixed Valence Perovskite Cs2Au2I6: A Potential Material for Thin-Film Pb-Free Photovoltaic Cells with Ultrahigh Efficiency, Adv. Mater., 2018, 30(12), 1707001, DOI:10.1002/adma.201707001.
- M. R. Filip and F. Giustino, Computational Screening of Homovalent Lead Substitution in Organic–Inorganic Halide Perovskites, J. Phys. Chem. C, 2016, 120(1), 166–173, DOI:10.1021/acs.jpcc.5b11845.
- M. Kar and T. Körzdörfer, Computational high throughput screening of inorganic cation based halide perovskites for perovskite only tandem solar cells, Mater. Res. Express, 2020, 7(5), 055502, DOI:10.1088/2053-1591/ab8c0d.
- S. Lu, Q. Zhou, L. Ma, Y. Guo and J. Wang, Rapid Discovery of Ferroelectric Photovoltaic Perovskites and Material Descriptors via Machine Learning, Small Methods, 2019, 3(11), 1900360, DOI:10.1002/smtd.201900360.
- Q. Xu, Z. Li, M. Liu and W.-J. Yin, Rationalizing Perovskite Data for Machine Learning and Materials Design, J. Phys. Chem. Lett., 2018, 9(24), 6948–6954, DOI:10.1021/acs.jpclett.8b03232.
- C. J. Bartel, C. Sutton, B. R. Goldsmith, R. Ouyang, C. B. Musgrave, L. M. Ghiringhelli and M. Scheffler, New tolerance factor to predict the stability of perovskite oxides and halides, Sci. Adv., 2019, 5(2), eaav0693, DOI:10.1126/sciadv.aav0693.
- G. H. Gu, J. Jang, J. Noh, A. Walsh and Y. Jung, Perovskite synthesizability using graph neural networks, npj Comput. Mater., 2022, 8(1), 71, DOI:10.1038/s41524-022-00757-z.
- G. Pilania, P. V. Balachandran, C. Kim and T. Lookman, Finding New Perovskite Halides via Machine Learning, Front. Mater., 2016, 3, DOI:10.3389/fmats.2016.00019.
- X. Wang, J. Yang, X. Wang, M. Faizan, H. Zou, K. Zhou, B. Xing, Y. Fu and L. Zhang, Entropy-Driven Stabilization of Multielement Halide Double-Perovskite Alloys, J. Phys. Chem. Lett., 2022, 13(22), 5017–5024, DOI:10.1021/acs.jpclett.2c01180.
- I. Toda-Caraballo, J. S. Wróbel, S. L. Dudarev, D. Nguyen-Manh and P. E. J. Rivera-Díaz-del-Castillo, Interatomic spacing distribution in multicomponent alloys, Acta Mater., 2015, 97, 156–169, DOI:10.1016/j.actamat.2015.07.010.
- B. Wu, Y. Zhao, H. Ali, R. Chen, H. Chen, J. Wen, Y. Liu, L. Liu, K. Yang, L. Zhang, Z. He, Q. Yao, H. Zhang, B. Sa, C. Wen, Y. Qiu, H. Xiong, M. Lin, Y. Liu, C. Wang and H. Su, A reasonable approach to describe the atom distributions and configurational entropy in high entropy alloys based on site preference, Intermetallics, 2022, 144, 107489, DOI:10.1016/j.intermet.2022.107489.
- O. N. Senkov, G. B. Wilks, D. B. Miracle, C. P. Chuang and P. K. Liaw, Refractory high-entropy alloys, Intermetallics, 2010, 18(9), 1758–1765, DOI:10.1016/j.intermet.2010.05.014.
- A. Takeuchi, K. Amiya, T. Wada, K. Yubuta, W. Zhang and A. Makino, Entropies in Alloy Design for High-Entropy and Bulk Glassy Alloys, Entropy, 2013, 15(9), 3810–3821, DOI:10.3390/e15093810.
- A. Takeuchi and A. Inoue, Calculations of Mixing Enthalpy and Mismatch Entropy for Ternary Amorphous Alloys, Mater. Trans., JIM, 2000, 41(11), 1372–1378, DOI:10.2320/matertrans1989.41.1372.
- Z. Wang, Y. Huang, Y. Yang, J. Wang and C. T. Liu, Atomic-size effect and solid solubility of multicomponent alloys, Scr. Mater., 2015, 94, 28–31, DOI:10.1016/j.scriptamat.2014.09.010.
- Y. F. Ye, Q. Wang, J. Lu, C. T. Liu and Y. Yang, The generalized thermodynamic rule for phase selection in multicomponent alloys, Intermetallics, 2015, 59, 75–80, DOI:10.1016/j.intermet.2014.12.011.
- Y. F. Ye, Q. Wang, J. Lu, C. T. Liu and Y. Yang, Design of high entropy alloys: A single-parameter thermodynamic rule, Scr. Mater., 2015, 104, 53–55, DOI:10.1016/j.scriptamat.2015.03.023.
- O. N. Senkov, J. D. Miller, D. B. Miracle and C. Woodward, Accelerated exploration of multi-principal element alloys with solid solution phases, Nat. Commun., 2015, 6(1), 6529, DOI:10.1038/ncomms7529.
- S. J. McCormack and A. Navrotsky, Thermodynamics of high entropy oxides, Acta Mater., 2021, 202, 1–21, DOI:10.1016/j.actamat.2020.10.043.
- Z. Rák, J. P. Maria and D. W. Brenner, Evidence for Jahn-Teller compression in the (Mg, Co, Ni, Cu, Zn)O entropy-stabilized oxide: A DFT study, Mater. Lett., 2018, 217, 300–303, DOI:10.1016/j.matlet.2018.01.111.
- Z. Rak, C. M. Rost, M. Lim, P. Sarker, C. Toher, S. Curtarolo, J.-P. Maria and D. W. Brenner, Charge compensation and electrostatic transferability in three entropy-stabilized oxides: Results from density functional theory calculations, J. Appl. Phys., 2016, 120(9), 095105, DOI:10.1063/1.4962135.
- Y.-P. Wang, G.-Y. Gan, W. Wang, Y. Yang and B.-Y. Tang,
Ab Initio Prediction of Mechanical and Electronic Properties of Ultrahigh Temperature High-Entropy Ceramics (Hf0.2Zr0.2Ta0.2M0.2Ti0.2)B2 (M = Nb, Mo, Cr), Phys. Status Solidi B, 2018, 255(8), 1800011, DOI:10.1002/pssb.201800011.
- B. Ye, T. Wen, M. C. Nguyen, L. Hao, C.-Z. Wang and Y. Chu, First-principles study, fabrication and characterization of (Zr0.25Nb0.25Ti0.25V0.25)C high-entropy ceramics, Acta Mater., 2019, 170, 15–23, DOI:10.1016/j.actamat.2019.03.021.
- A. Zunger, S. H. Wei, L. G. Ferreira and J. E. Bernard, Special quasirandom structures, Phys. Rev. Lett., 1990, 65(3), 353–356, DOI:10.1103/PhysRevLett.65.353.
- J. Yang, P. Manganaris and A. Mannodi-Kanakkithodi, A high-throughput computational dataset of halide perovskite alloys, Digital Discovery, 2023, 2(3), 856–870, 10.1039/D3DD00015J.
- J. D. Gale, GULP: A computer program for the symmetry-adapted simulation of solids, J. Chem. Soc., Faraday Trans., 1997, 93(4), 629–637, 10.1039/A606455H.
- Y.-X. Guo, Y.-B. Zhuang, J. Shi and J. Cheng, ChecMatE: A workflow package to automatically generate machine learning potentials and phase diagrams for semiconductor alloys, J. Chem. Phys., 2023, 159(9), 094801, DOI:10.1063/5.0166858.
- G. V. Lewis and C. R. A. Catlow, Potential models for ionic oxides, J. Phys. C-Solid State Phys., 1985, 18(6), 1149, DOI:10.1088/0022-3719/18/6/010.
- W. Smith and T. R. Forester, DL_POLY_2.0: A general-purpose parallel molecular dynamics simulation package, J. Mol. Graphics, 1996, 14(3), 136–141, DOI:10.1016/S0263-7855(96)00043-4.
- G. Anand, A. P. Wynn, C. M. Handley and C. L. Freeman, Phase stability and distortion in high-entropy oxides, Acta Mater., 2018, 146, 119–125, DOI:10.1016/j.actamat.2017.12.037.
- C. Oses, C. Toher and S. Curtarolo, Data-driven design of inorganic materials with the Automatic Flow Framework for Materials Discovery, MRS Bull., 2018, 43(9), 670–675, DOI:10.1557/mrs.2018.207.
- P. Sarker, T. Harrington, C. Toher, C. Oses, M. Samiee, J.-P. Maria, D. W. Brenner, K. S. Vecchio and S. Curtarolo, High-entropy high-hardness metal carbides discovered by entropy descriptors, Nat. Commun., 2018, 9(1), 4980, DOI:10.1038/s41467-018-07160-7.
- C. Toher, C. Oses, D. Hicks and S. Curtarolo, Unavoidable disorder and entropy in multi-component systems, npj Comput. Mater., 2019, 5(1), 69, DOI:10.1038/s41524-019-0206-z.
- Y. Zhong, H. Sabarou, X. Yan, M. Yang, M. C. Gao, X. Liu and R. D. Sisson, Exploration of high entropy ceramics (HECs) with computational thermodynamics – A case study with LaMnO3±δ, Mater. Des., 2019, 182, 108060, DOI:10.1016/j.matdes.2019.108060.
- G. B. Stringfellow, Calculation of regular solution interaction parameters in semiconductor solid solutions, J. Phys. Chem. Solids, 1973, 34(10), 1749–1751, DOI:10.1016/S0022-3697(73)80140-4.
- F. Otto, Y. Yang, H. Bei and E. P. George, Relative effects of enthalpy and entropy on the phase stability of equiatomic high-entropy alloys, Acta Mater., 2013, 61(7), 2628–2638, DOI:10.1016/j.actamat.2013.01.042.
- L. M. Foster, A Lattice Parameter Criterion for Miscibility Gaps in the III–V and II–VI Pseudobinary Solid Solutions, J. Electrochem. Soc., 1974, 121(12), 1662, DOI:10.1149/1.2401764.
- G. B. Stringfellow, Miscibility gaps in quaternary III/V alloys, J. Cryst. Growth, 1982, 58(1), 194–202, DOI:10.1016/0022-0248(82)90226-3.
- G. Han, I. W. Yeu, J. Park, K. H. Ye, S.-C. Lee, C. S. Hwang and J.-H. Choi, Effect of local strain energy to predict accurate phase diagram of III–V pseudobinary systems: case of Ga(As,Sb) and (In,Ga)As, J. Phys. D: Appl. Phys., 2021, 54(4), 045104, DOI:10.1088/1361-6463/abbf78.
- E.-A. Zen, Validity of “Vegard's law”, Am. Mineral., 1956, 41(5–6), 523–524 CAS.
- L. Vegard and H. Dale, VIII. Untersuchungen über Mischkristalle und Legierungen, Z. Kristallogr. – Cryst. Mater., 1928, 67(1–6), 148–162, DOI:10.1524/zkri.1928.67.1.148.
- S. D. Baranovskii, A. V. Nenashev, D. Hertel, F. Gebhard and K. Meerholz, Energy Scales of Compositional Disorder in Alloy Semiconductors, ACS Omega, 2022, 7(50), 45741–45751, DOI:10.1021/acsomega.2c05426.
- M. Rittiruam, J. Noppakhun, S. Setasuban, N. Aumnongpho, A. Sriwattana, S. Boonchuay, T. Saelee, C. Wangphon, A. Ektarawong, P. Chammingkwan, T. Taniike, S. Praserthdam and P. Praserthdam, High-throughput materials screening algorithm based on first-principles density functional theory and artificial neural network for high-entropy alloys, Sci. Rep., 2022, 12(1), 16653, DOI:10.1038/s41598-022-21209-0.
- Y. Zhang, Y. J. Zhou, J. P. Lin, G. L. Chen and P. K. Liaw, Solid-Solution Phase Formation Rules for Multi-component Alloys, Adv. Eng. Mater., 2008, 10(6), 534–538, DOI:10.1002/adem.200700240.
- X. D. Xu, S. Guo, T. G. Nieh, C. T. Liu, A. Hirata and M. W. Chen, Effects of mixing enthalpy and cooling rate on phase formation of AlxCoCrCuFeNi high-entropy alloys, Materialia, 2019, 6, 100292, DOI:10.1016/j.mtla.2019.100292.
- W. Q. Feng, S. M. Zheng, Y. Qi and S. Q. Wang, Periodic Maximum Entropy Random Structure Models for High-Entropy Alloys, Mater. Sci. Forum, 2017, 898, 611–621, DOI:10.4028/www.scientific.net/MSF.898.611.
- S.-m Zheng, W.-q Feng and S.-q Wang, Elastic properties of high entropy alloys by MaxEnt approach, Comput. Mater. Sci., 2018, 142, 332–337, DOI:10.1016/j.commatsci.2017.09.060.
- S. Yang and Y. Zhong,
Ab Initio Modeling of fcc Fe-Co-Cr-Ni High Entropy Alloys with Full Composition Range, J. Phase Equilib. Diffus., 2021, 42(5), 656–672, DOI:10.1007/s11669-021-00905-w.
- L. Q. Jiang, J. K. Guo, H. B. Liu, M. Zhu, X. Zhou, P. Wu and C. H. Li, Prediction of lattice constant in cubic perovskites, J. Phys. Chem. Solids, 2006, 67(7), 1531–1536, DOI:10.1016/j.jpcs.2006.02.004.
- C. Ye, J. Yang, L. Yao and N. Chen, Regularities of formation and lattice distortion of perovskite-type compounds, Chin. Sci. Bull., 2002, 47(6), 458–460, DOI:10.1360/02tb9105.
- H. Ohtani, K. Kojima, K. Ishida and T. Nishizawa, Miscibility gap in II–VI alloy semiconductor systems, J. Alloys Compd., 1992, 182(1), 103–114, DOI:10.1016/0925-8388(92)90579-X.
- T. Wang, H. Chen, Z. Yang, J. Liang and S. Dai, High-Entropy Perovskite Fluorides: A New Platform for Oxygen Evolution Catalysis, J. Am. Chem. Soc., 2020, 142(10), 4550–4554, DOI:10.1021/jacs.9b12377.
- Y. Huang, R. Ding, D. Ying, Y. Huang, C. Tan, T. Yan, X. Sun and E. Liu, A F-deficient and high-Mn ternary perovskite fluoride anode with a dominant conversion mechanism
for advanced Li-ion batteries, Chem. Commun., 2021, 57(62), 7705–7708, 10.1039/D1CC00910A.
- Y.-F. Huang, R. Ding, D.-F. Ying, Y.-X. Huang, T. Yan, C.-N. Tan, X.-J. Sun and E.-H. Liu, A novel Li-ion supercapattery by K-ion vacant ternary perovskite fluoride anode with pseudocapacitive conversion/insertion dual mechanisms, Rare Met., 2022, 41(7), 2491–2504, DOI:10.1007/s12598-022-01979-2.
- Z. Jia, R. Ding, W. Yu, Y. Li, A. Wang, M. Liu, F. Yang, X. Sun and E. Liu, Unraveling the Charge Storage and Activity-Enhancing Mechanisms of Zn-Doping Perovskite Fluorides and Engineering the Electrodes and Electrolytes for Wide-Temperature Aqueous Supercabatteries, Adv. Funct. Mater., 2022, 32(1), 2107674, DOI:10.1002/adfm.202107674.
- Z. Jia, W. Shi, R. Ding, W. Yu, Y. Li, C. Tan, X. Sun and E. Liu, Conversion-type NiCoMn triple perovskite fluorides for advanced aqueous supercapacitors, batteries and supercapatteries, Chem. Commun., 2021, 57(64), 7962–7965, 10.1039/D1CC02488D.
- Y. Li, R. Ding, Z. Jia, W. Yu, A. Wang, M. Liu, F. Yang, Y. Zhang, Q. Fang, M. Yan, J. Xie, X. Sun and E. Liu, Unlocking the intrinsic mechanisms of A-site K/Na doped perovskite fluorides pseudocapacitive cathode materials for enhanced aqueous zinc-based batteries, Energy Storage Mater., 2023, 57, 334–345, DOI:10.1016/j.ensm.2023.02.020.
- H. Mao, L. Wang, J. Li, X. Jiang, S. Xue, M. Li, J. Zhu, B. Fan, T. Xu, G. Shao, H. Xu, H. Wang, R. Zhang and H. Lu, High-Entropy Cs(Pb1/3Mn1/3Ni1/3)Br3 Perovskite Nanocrystals Prepared by High Energy Ball Milling and their Luminescence Properties, Part. Part. Syst. Charact., 2022, 39(9), 2200073, DOI:10.1002/ppsc.202200073.
- W. Shi, W. Yu, R. Ding, Z. Jia, Y. Li, Y. Huang, C. Tan, X. Sun and E. Liu, Bipolar redox electrolyte-synergistically mediated NiCoMn-811 high-Ni ternary perovskite fluorides for advanced supercapacitors in both alkaline and neutral media, J. Mater. Chem. A, 2021, 9(15), 9624–9633, 10.1039/D1TA01156A.
- C. Tan, R. Ding, Y. Huang, T. Yan, Y. Huang, F. Yang, X. Sun, P. Gao and E. Liu, A vacancy-rich perovskite fluoride K0.79Ni0.25Co0.36Mn0.39F2.83@rGO anode for advanced Na-based dual-ion batteries, Chem. Commun., 2021, 57(47), 5830–5833, 10.1039/D1CC01477C.
- A. Wang, R. Ding, Y. Li, M. Liu, F. Yang, Y. Zhang, Q. Fang, M. Yan, J. Xie, Z. Chen, Z. Yan, Y. He, J. Guo, X. Sun and E. Liu, Redox Electrolytes-Assisting Aqueous Zn-Based Batteries by Pseudocapacitive Multiple Perovskite Fluorides Cathode and Charge Storage Mechanisms, Small, 2023, 2302333, DOI:10.1002/smll.202302333.
- T. Wang, J. Fan, C.-L. Do-Thanh, X. Suo, Z. Yang, H. Chen, Y. Yuan, H. Lyu, S. Yang and S. Dai, Perovskite Oxide–Halide Solid Solutions: A Platform for Electrocatalysts, Angew. Chem., Int. Ed., 2021, 60(18), 9953–9958, DOI:10.1002/anie.202101120.
- X. Wang, G. Liu, C. Tang, H. Tang, W. Zhang, Z. Ju, J. Jiang, Q. Zhuang and Y. Cui, A novel high entropy perovskite fluoride anode with 3D cubic framework for advanced lithium-ion battery, J. Alloys Compd., 2023, 934, 167889, DOI:10.1016/j.jallcom.2022.167889.
- T. Yan, R. Ding, Y. Huang, D. Ying, C. Tan, Y. Huang, F. Yang, X. Sun, P. Gao and E. Liu, A novel sodium-ion supercabattery based on vacancy defective Ni–Co–Mn ternary perovskite fluoride electrode materials, J. Mater. Chem. A, 2021, 9(25), 14276–14284, 10.1039/D1TA02894D.
- T. Yan, Y. Huang, R. Ding, W. Shi, D. Ying, Z. Jia, C. Tan, Y. Huang, X. Sun and E. Liu, Pseudocapacitive trimetallic NiCoMn-111 perovskite fluorides for advanced Li-ion supercabatteries, Nanoscale Adv., 2021, 3(19), 5703–5710, 10.1039/D1NA00329A.
- F. Yang, R. Ding, Z. Jia, W. Yu, Y. Li, A. Wang, M. Liu, J. Xie, M. Yan, Q. Fang, Y. Zhang, X. Sun and E. Liu, High specific energy and power sodium-based dual-ion supercabatteries by pseudocapacitive Ni-Zn-Mn ternary perovskite fluorides@reduced graphene oxides anodes with conversion-alloying-intercalation triple mechanisms, Energy Storage Mater., 2022, 53, 222–237, DOI:10.1016/j.ensm.2022.08.049.
- D. Ying, Y. Li, R. Ding, W. Shi, Q. Xu, Y. Huang, Z. Jia, W. Yu, X. Sun, P. Gao, E. Liu and X. Wang, Nanosilver-Promoted Trimetallic Ni–Co–Mn Perovskite Fluorides for Advanced Aqueous Supercabatteries with Pseudocapacitive Multielectrons Phase Conversion Mechanisms, Adv. Funct. Mater., 2021, 31(24), 2101353, DOI:10.1002/adfm.202101353.
- W. Yu, R. Ding, Z. Jia, Y. Li, A. Wang, M. Liu, F. Yang, X. Sun and E. Liu, Pseudocapacitive Co-Free Trimetallic Ni-Zn-Mn Perovskite Fluorides Enable Fast-Rechargeable Zn-Based Aqueous Batteries, Adv. Funct. Mater., 2022, 32(19), 2112469, DOI:10.1002/adfm.202112469.
- J. Jung, Y. Yun, S. W. Yang, H. G. Oh, A. Y. Jeon, Y. Nam, Y.-W. Heo, W.-S. Chae and S. Lee, Ternary diagrams of phase, stability, and optical properties of cesium lead mixed-halide perovskites, Acta Mater., 2023, 246, 118661, DOI:10.1016/j.actamat.2022.118661.
- W. Zhang, H. Liu, Y. Qu, J. Cui, W. Zhang, T. Shi and H.-L. Wang, B-Site Co-Doping Coupled with Additive Passivation Pushes the Efficiency of Pb–Sn Mixed Inorganic Perovskite Solar Cells to Over 17%, Adv. Mater., 2023, 2309193, DOI:10.1002/adma.202309193.
- S. Baek, S. Kim, J. Y. Noh, J. H. Heo, S. H. Im, K.-H. Hong and S.-W. Kim, Development of Mixed-Cation CsxRb1–xPbX3 Perovskite Quantum Dots and Their Full-Color Film with High Stability and Wide Color Gamut, Adv. Opt. Mater., 2018, 6(15), 1800295, DOI:10.1002/adom.201800295.
- M. Zirak, E. Moyen, H. Alehdaghi, A. Kanwat, W.-C. Choi and J. Jang, Anion- and Cation-Codoped All-Inorganic Blue-Emitting Perovskite Quantum Dots for Light-Emitting Diodes, ACS Appl. Nano Mater., 2019, 2(9), 5655–5662, DOI:10.1021/acsanm.9b01187.
- L. Stand, M. Zhuravleva, B. Chakoumakos, H. Wei, J. Johnson, V. Martin, M. Loyd, D. Rutstrom, W. McAlexander, Y. Wu, M. Koschan and C. L. Melcher, Characterization of mixed halide scintillators: CsSrBrI2:Eu, CsCaBrI2:Eu and CsSrClBr2:Eu, J. Lumin., 2019, 207, 70–77, DOI:10.1016/j.jlumin.2018.10.108.
- T. Wen, G. Gu, B. Wang, W. Zhang and R. Wang, Cyan-rich sunlight-like spectra from Mn2+-doped CsCd(Cl1−yBry)3 perovskites with dual tunable emissions and high stability, J. Mater. Chem. C, 2023, 11(21), 6989–6998, 10.1039/D2TC05455H.
- Y. Ji, M. Wang, Z. Yang, H. Wang, M. A. Padhiar, J. Shi, H. Qiu and A. S. Bhatti,
In Situ Synthesis of UltraStable TiO2 Coating Rb+-Doped Red Emitting CsPbBrI2 Perovskite Quantum Dots, J. Phys. Chem. C, 2022, 126(3), 1542–1551, DOI:10.1021/acs.jpcc.1c09945.
- Y.-H. Lin, Z.-H. Qiu, S.-H. Wang, X.-H. Zhang and S.-F. Wu, All-inorganic RbxCs1−xPbBrI2 perovskite nanocrystals with wavelength-tunable properties for red light-emitting, Inorg. Chem. Commun., 2019, 103, 47–52, DOI:10.1016/j.inoche.2019.03.007.
- S. Lee, J. Moon, J. Ryu, B. Parida, S. Yoon, D.-G. Lee, J. S. Cho, S. Hayase and D.-W. Kang, Inorganic narrow bandgap CsPb0.4Sn0.6I2.4Br0.6 perovskite solar cells with exceptional efficiency, Nano Energy, 2020, 77, 105309, DOI:10.1016/j.nanoen.2020.105309.
- N. Li, Z. Zhu, J. Li, A. K.-Y. Jen and L. Wang, Inorganic CsPb1−xSnxIBr2 for Efficient Wide-Bandgap Perovskite Solar Cells, Adv. Energy Mater., 2018, 8(22), 1800525, DOI:10.1002/aenm.201800525.
- Y. Shang, X. Li, W. Lian, X. Jiang, X. Wang, T. Chen, Z. Xiao, M. Wang, Y. Lu and S. Yang, Lead acetate as a superior lead source enables highly efficient and stable all-inorganic lead-tin perovskite solar cells, Chem. Eng. J., 2023, 457, 141246, DOI:10.1016/j.cej.2022.141246.
- Q. Wen, C. Duan, F. Zou, D. Luo, J. Li, Z. Liu, J. Wang and K. Yan, All-inorganic CsPb1−xSnxI2Br perovskites mediated by dicyandiamide additive for efficient 4-terminal tandem solar cell, Chem. Eng. J., 2023, 452, 139697, DOI:10.1016/j.cej.2022.139697.
- W. Zhang, H. Liu, X. Qi, Y. Yu, Y. Zhou, Y. Xia, J. Cui, Y. Shi, R. Chen and H.-L. Wang, Oxalate Pushes Efficiency of CsPb0.7Sn0.3IBr2 Based All-Inorganic Perovskite Solar Cells to over 14%, Adv. Sci., 2022, 9(11), 2106054, DOI:10.1002/advs.202106054.
- Z. Zhang, L. Dai, M. Zhang, H. Ban, Z. Liu, H. Yu, A. Gu, X.-L. Zhang, S. Chen, Y. Wang, Y. Shen and M. Wang, Surface Modification in CsPb0.5Sn0.5I2Br Inorganic Perovskite Solar Cells: Effects of Bifunctional Dipolar Molecules on Photovoltaic Performance, ACS Appl. Mater. Interfaces, 2023, 15(30), 36594–36601, DOI:10.1021/acsami.3c07018.
- F. Yang, D. Hirotani, G. Kapil, M. A. Kamarudin, C. H. Ng, Y. Zhang, Q. Shen and S. Hayase, All-Inorganic CsPb1−xGexI2Br Perovskite with Enhanced Phase Stability and Photovoltaic Performance, Angew. Chem., Int. Ed., 2018, 57(39), 12745–12749, DOI:10.1002/anie.201807270.
- Y. Hu, Y. Zhang, C. Yang, J. Li and L. Wang, The cation–anion co-exchange in CsPb1−xFex(Br1−yCly)3 nanocrystals prepared using a hot injection method, RSC Adv., 2020, 10(55), 33080–33085, 10.1039/D0RA06238C.
- C. Wu, Y. Li, Z. Xia, C. Ji, Y. Tang, J. Zhang, C. Ma and J. Gao, Enhancing Photoluminescence of
CsPb(ClxBr1−x)3 Perovskite Nanocrystals by Fe2+ Doping, Nanomaterials, 2023, 13(3), 533, DOI:10.3390/nano13030533.
- V. Naresh and N. Lee, Zn(II)-Doped Cesium Lead Halide Perovskite Nanocrystals with High Quantum Yield and Wide Color Tunability for Color-Conversion Light-Emitting Displays, ACS Appl. Nano Mater., 2020, 3(8), 7621–7632, DOI:10.1021/acsanm.0c01254.
- H. Sun, J. Zhang, X. Gan, L. Yu, H. Yuan, M. Shang, C. Lu, D. Hou, Z. Hu, Y. Zhu and L. Han, Pb-Reduced CsPb0.9Zn0.1I2Br Thin Films for Efficient Perovskite Solar Cells, Adv. Energy Mater., 2019, 9(25), 1900896, DOI:10.1002/aenm.201900896.
- I. N. Belyaev and E. A. Shurginov, Zh. Neorg. Khim., 1970, 15, 1401 CAS.
- H. M. Ghaithan, S. M. H. Qaid, Z. A. Alahmed, M. Hezam, A. Lyras, M. Amer and A. S. Aldwayyan, Anion Substitution Effects on the Structural, Electronic, and Optical Properties of Inorganic CsPb(I1–xBrx)3 and CsPb(Br1–xClx)3 Perovskites: Theoretical and Experimental Approaches, J. Phys. Chem. C, 2021, 125(1), 886–897, DOI:10.1021/acs.jpcc.0c07983.
- H. M. Ghaithan, S. M. H. Qaid, K. K. AlHarbi, A. F. Bin Ajaj, B. A. Al-Asbahi and A. S. Aldwayyan, Amplified Spontaneous Emission from Thermally Evaporated High-Quality Thin Films of CsPb(Br1–xYx)3 (Y = I, Cl) Perovskites, Langmuir, 2022, 38(28), 8607–8613, DOI:10.1021/acs.langmuir.2c00861.
- T. C. Jellicoe, J. M. Richter, H. F. J. Glass, M. Tabachnyk, R. Brady, S. E. Dutton, A. Rao, R. H. Friend, D. Credgington, N. C. Greenham and M. L. Böhm, Synthesis and Optical Properties of Lead-Free Cesium Tin Halide Perovskite Nanocrystals, J. Am. Chem. Soc., 2016, 138(9), 2941–2944, DOI:10.1021/jacs.5b13470.
- L. Peedikakkandy and P. Bhargava, Composition dependent optical, structural and photoluminescence characteristics of cesium tin halide perovskites, RSC Adv., 2016, 6(24), 19857–19860, 10.1039/C5RA22317B.
- D. E. Scaife, P. F. Weller and W. G. Fisher, Crystal preparation and properties of cesium tin(II) trihalides, J. Solid State Chem., 1974, 9(3), 308–314, DOI:10.1016/0022-4596(74)90088-7.
- S. Sharma, N. Weiden and A. Weiss, Phase Diagrams Of Quasibinary Systems Of The Type: Abx3-A′bx3; Abx3-Ab′x3 And Abx3-Abx′3; X = Halogen, Z. Phys. Chem., 1992, 175(1), 63–80 CrossRef CAS.
- Z.-G. Lin, L.-C. Tang and C.-P. Chou, Infrared properties of CsGe(BrxCl1−x)3, nonlinear optical rhombohedral semiconductor, J. Phys.: Condens. Matter, 2007, 19(47), 476209, DOI:10.1088/0953-8984/19/47/476209.
- Z.-G. Lin, L.-C. Tang and C.-P. Chou, Characterization and properties of infrared NLO crystals: AGeX3 (A = Rb, Cs; X = Cl, Br), J. Cryst. Growth, 2008, 310(13), 3224–3229, DOI:10.1016/j.jcrysgro.2008.03.018.
- S. R. Lüthi and M. J. Riley, Ni(II)-Doped CsCdBrCl2:
Variation of Spectral and Structural Properties via Mixed-Halide Coordination, Inorg. Chem., 2001, 40(2), 196–207, DOI:10.1021/ic000899l.
- M. P. Plokker, D. A. Biner, N. Dusoswa, P. Dorenbos, K. W. Krämer and E. Van Der Kolk, Photoluminescence and excited states dynamics of Tm2+-doped CsCa(Cl/Br)3 and CsCa(Br/I)3 perovskites, J. Phys.: Mater., 2021, 4(4), 045004, DOI:10.1088/2515-7639/ac24ed.
- H. Näsström, P. Becker, J. A. Márquez, O. Shargaieva, R. Mainz, E. Unger and T. Unold, Dependence of phase transitions on halide ratio in inorganic CsPb(BrxI1−x)3 perovskite thin films obtained from high-throughput experimentation, J. Mater. Chem. A, 2020, 8(43), 22626–22631, 10.1039/D0TA08067E.
- L. Yuan, M. Yuan, H. Xu, C. Hou and X. Meng, Moisture-stimulated reversible thermochromic CsPbI3−xBrx films: In-situ spectroscopic-resolved structure and optical properties, Appl. Surf. Sci., 2022, 573, 151484, DOI:10.1016/j.apsusc.2021.151484.
- G. Natta and L. Passerini, Isomorfismo, polimorfismo e morfotropia I. Composti del tipo ABX3, Gazz. Chim. Ital., 1928, 58, 472–484 CAS.
- N. Bontemps, C. Grisolia, M. Nerozzi and B. Briat, Optical and magneto-optical study of the magnetic properties of RbFeCl3, RbFeBr3, and disordered materials of intermediate composition, J. Appl. Phys., 1982, 53(3), 2710–2712, DOI:10.1063/1.330940.
- A. Harrison and D. Visser, Magnetic ordering effects in the random mixed one-dimensional ferromagnet-antiferromagnet system RbFeCl3−xBrx, J. Phys.: Condens. Matter, 1989, 1(4), 733, DOI:10.1088/0953-8984/1/4/008.
- H. P. Beck, M. Schramm and R. Haberkorn, The InSnCl3-Type Arrangement: II. High Pressure Synthesis of TlPbCl3 and of Solid Solutions Containing Rb or Br, J. Solid State Chem., 1999, 146(2), 351–354, DOI:10.1006/jssc.1999.8361.
- D. Amgar, T. Binyamin, V. Uvarov and L. Etgar, Near ultra-violet to mid-visible band gap tuning of mixed cation RbxCs1−xPbX3 (X = Cl or Br) perovskite nanoparticles, Nanoscale, 2018, 10(13), 6060–6068, 10.1039/C7NR09607K.
- M. R. Linaburg, E. T. McClure, J. D. Majher and P. M. Woodward, Cs1–xRbxPbCl3 and Cs1–xRbxPbBr3 Solid Solutions: Understanding Octahedral Tilting in Lead Halide Perovskites, Chem. Mater., 2017, 29(8), 3507–3514, DOI:10.1021/acs.chemmater.6b05372.
- W. Jia, Q. Wei, S. Ge, C. Peng, T. Huang, S. Yao, Y. Tian, T. Chang, R. Zeng and B. Zou, Polaronic Magnetic Excitons and Photoluminescence in Mn2+-Doped CsCdBr3 Metal Halides, J. Phys. Chem. C, 2021, 125(32), 18031–18039, DOI:10.1021/acs.jpcc.1c05127.
- F. Lahoz, P. J. Alonso, B. Villacampa and R. Alcala, Spectroscopic properties of Mn2+ ions in mixed fluoroperovskites, Radiat. Eff. Defects Solids, 1995, 135(1–4), 163–167, DOI:10.1080/10420159508229827.
- F. Lahoz, M. Díaz, B. Villacampa, R. Cases and R. Alcalá, Influence of the host lattice on the photoluminescence of Ni2+ ions in Rb1−xCsxCaF3 and RbCa1−xCdxF3 crystals, J. Appl. Phys., 1997, 82(10), 5121–5125, DOI:10.1063/1.366314.
- P. Ghigna, M. Scavini, C. Mazzoli, M. Brunelli, C. Laurenti and C. Ferrero, Experimental disentangling of orbital and lattice energy scales by inducing cooperative Jahn-Teller melting in KCu1−xMgxF3 solid solutions, Phys. Rev. B: Condens. Matter Mater. Phys., 2010, 81(7), 073107, DOI:10.1103/PhysRevB.81.073107.
- C. Oliva, M. Scavini, S. Cappelli, C. Bottalo, C. Mazzoli and P. Ghigna, Melting of Orbital Ordering in KMgxCu1−xF3 Solid Solution, J. Phys. Chem. B, 2007, 111(21), 5976–5983, DOI:10.1021/jp067539p.
- P. A. Fleury, W. Hayes and H. J. Guggenheim, Magnetic scattering of light in K(NiMg)F3, J. Phys. C-Solid State Phys., 1975, 8(13), 2183, DOI:10.1088/0022-3719/8/13/027.
- A. Karmakar, A. Bhattacharya, G. M. Bernard, A. Mar and V. K. Michaelis, Revealing the Local Sn and Pb Arrangements in CsSnxPb1−xBr3 Perovskites with Solid-State NMR Spectroscopy, ACS Mater. Lett., 2021, 3(3), 261–267, DOI:10.1021/acsmaterialslett.0c00596.
- X. Zhang, W. Cao, W. Wang, B. Xu, S. Liu, H. Dai, S. Chen, K. Wang and X. W. Sun, Efficient light-emitting diodes based on green perovskite nanocrystals with mixed-metal cations, Nano Energy, 2016, 30, 511–516, DOI:10.1016/j.nanoen.2016.10.039.
- T. Suzuki, H. Guo, I. Kawasaki, I. Watanabe, T. Goto, K. Katayama and H. Tanaka, Disappearance of Gapped Mott Insulating Phase Neighboring Bose Glass Phase in Tl1−xKxCuCl3 Detected by Longitudinal-Field Muon Spin Relaxation, J. Phys. Soc. Jpn., 2014, 83(8), 084703, DOI:10.7566/JPSJ.83.084703.
- T. Suzuki, F. Yamada, I. Watanabe, T. Goto, A. Oosawa and H. Tanaka, Bond-randomness effect on the quantum spin system Tl1−xKxCuCl3 probed by muon-spin-relaxation method, Phys. B, 2009, 404(5), 590–593, DOI:10.1016/j.physb.2008.11.108.
- F. Yamada, H. Tanaka, T. Ono and H. Nojiri, Transition from Bose glass to a condensate of triplons in Tl1−xKxCuCl3, Phys. Rev. B: Condens. Matter Mater. Phys., 2011, 83(2), 020409, DOI:10.1103/PhysRevB.83.020409.
- P. Chartrand and A. D. Pelton, Thermodynamic Evaluation and Optimization of the LiCl–NaCl–KCl–RbCl–CsCl–MgCl2–CaCl2–SrCl2 System Using The Modified Quasichemical Model, Can. Metall. Q., 2000, 39(4), 405–420, DOI:10.1179/cmq.2000.39.4.405.
- Y. Chornodolskyy, G. Stryganyuk, S. Syrotyuk, A. Voloshinovskii and P. Rodnyi, Features of the core–valence luminescence and electron energy band structure of A1−xCsxCaCl3 (A = K,Rb) crystals, J. Phys.: Condens. Matter, 2007, 19(47), 476211, DOI:10.1088/0953-8984/19/47/476211.
- K. Takahashi, M. Koshimizu, Y. Fujimoto, T. Yanagida and K. Asai, Auger-free luminescence characteristics of Rb1−xCsxCaCl3, J. Ceram. Soc. Jpn., 2018, 126(10), 755–760, DOI:10.2109/jcersj2.18051.
- V. Vaněček, J. Páterek, R. Král, R. Kučerková, V. Babin, J. Rohlíček, R. Cala’, N. Kratochwil, E. Auffray and M. Nikl, (INVITED) Ultraviolet cross-luminescence in ternary chlorides of alkali and alkaline-earth metals, Opt. Mater.: X, 2021, 12, 100103, DOI:10.1016/j.omx.2021.100103.
- Q. Dong, B. Tian, W. Zhang and L. He, Facile synthesis of KaCs1−aPbBr3@ molecular sieve SBA-15 composite with improved luminescence and wet/thermal stability, Colloids Surf., A, 2022, 648, 129258, DOI:10.1016/j.colsurfa.2022.129258.
- G. Shao, S. Liu, L. Ding, Z. Zhang, W. Xiang and X. Liang, KxCs1−xPbBr3 NCs glasses possessing super optical properties and stability for white light emitting diodes, Chem. Eng. J., 2019, 375, 122031, DOI:10.1016/j.cej.2019.122031.
-
S. H. S. S. Dintakurti, Phase evolution and local structure in [Cs, MA][Pb, Sr][Cl, Br]3 perovskites, Doctoral Thesis, Nanyang Technological University, 2021 Search PubMed.
- J.-M. Dance, J. Grannec and A. Tressand, C. R. Acad. Sci., 1975, C281, 91 Search PubMed.
- M. Hu, M. Chen, P. Guo, H. Zhou, J. Deng, Y. Yao, Y. Jiang, J. Gong, Z. Dai, Y. Zhou, F. Qian, X. Chong, J. Feng, R. D. Schaller, K. Zhu, N. P. Padture and Y. Zhou, Sub-1.4eV bandgap inorganic perovskite solar cells with long-term stability, Nat. Commun., 2020, 11(1), 151, DOI:10.1038/s41467-019-13908-6.
- M. Hu, Y. Zhang, J. Gong, H. Zhou, X. Huang, M. Liu, Y. Zhou and S. Yang, Surface Sn(IV) Hydrolysis Improves Inorganic Sn–Pb Perovskite Solar Cells, ACS Energy Lett., 2023, 8(2), 1035–1041, DOI:10.1021/acsenergylett.2c02435.
- M. Liu, H. Pasanen, H. Ali-Löytty, A. Hiltunen, K. Lahtonen, S. Qudsia, J.-H. Smått, M. Valden, N. V. Tkachenko and P. Vivo, B-Site Co-Alloying with Germanium Improves the Efficiency and Stability of All-Inorganic Tin-Based Perovskite Nanocrystal Solar Cells, Angew. Chem., Int. Ed., 2020, 59(49), 22117–22125, DOI:10.1002/anie.202008724.
- F. Borsa, D. J. Benard, W. C. Walker and A. Baviera, NMR and birefringence study of structural transitions in disordered crystals: RbxK1−xMnF3, Phys. Rev. B: Solid State, 1977, 15(1), 84–94, DOI:10.1103/PhysRevB.15.84.
- J. C. Cousseins, Etude dans l’etat solide de quelques fluorures ternaires: Synthese; identification; filiation; miscibilite, Rev. Chim. Miner., 1964, 1, 573–616 CAS.
- J. Kapusta, Ph Daniel and A. Ratuszna, Vibrational investigation and phase transitions in the KMnF3 doped perovskite crystals (Li+, Na+, Rb+ and Cs+), J. Phys.: Condens. Matter, 2002, 14(21), 5433, DOI:10.1088/0953-8984/14/21/317.
- P. Todorović, D. Ma, B. Chen, R. Quintero-Bermudez, M. I. Saidaminov, Y. Dong, Z.-H. Lu and E. H. Sargent, Spectrally Tunable and Stable Electroluminescence Enabled by Rubidium Doping of CsPbBr3 Nanocrystals, Adv. Opt. Mater., 2019, 7(24), 1901440, DOI:10.1002/adom.201901440.
- H. Wu, Y. Yang, D. Zhou, K. Li, J. Yu, J. Han, Z. Li, Z. Long, J. Ma and J. Qiu, Rb+ cations enable the change of luminescence properties in perovskite (RbxCs1−xPbBr3) quantum dots, Nanoscale, 2018, 10(7), 3429–3437, 10.1039/C7NR07776A.
- F. Lahoz, B. Villacampa, R. Alcalá, C. Marquina and M. R. Ibarra, Cubic-to-tetragonal structural phase transition in Rb1−xCsxCaF3 solid solutions: Thermal expansion and EPR studies, Phys. Rev. B: Condens. Matter Mater. Phys., 1997, 55(13), 8148–8154, DOI:10.1103/PhysRevB.55.8148.
- J. Deng, J. Xun, W. Shen, M. Li and R. He, Phase Regulation of CsPb2Br5/CsPbBr3 Perovskite Nanocrystals by Doping with Divalent Cations: Implications for Optoelectronic Devices with Enhanced Stability and Reduced Toxicity, ACS Appl. Nano Mater., 2021, 4(9), 9213–9222, DOI:10.1021/acsanm.1c01737.
- D. B. Straus and R. J. Cava, Tuning the Band Gap in the Halide Perovskite CsPbBr3 through Sr Substitution, ACS Appl. Mater. Interfaces, 2022, 14(30), 34884–34890, DOI:10.1021/acsami.2c09275.
- H. Tanaka, K. Iio and K. Nagata, Magnetic properties of Rb1−xKxNiCl3, RbVBr3 and CsNiI3, J. Magn. Magn. Mater., 1992, 104–107, 829–830, DOI:10.1016/0304-8853(92)90380-7.
- A. Bernasconi, A. Rizzo, A. Listorti, A. Mahata, E. Mosconi, F. De Angelis and L. Malavasi, Synthesis, Properties, and Modeling of Cs1−xRbxSnBr3 Solid Solution: A New Mixed-Cation Lead-Free All-Inorganic Perovskite System, Chem. Mater., 2019, 31(9), 3527–3533, DOI:10.1021/acs.chemmater.9b00837.
- P. Daniel, J. Toulouse and M. Rousseau, Phase transitions in mixed disordered crystals Rb1−xKxCaF3 (0 < x < 1) investigated by Raman spectroscopy, Eur. Phys. J.: Appl. Phys., 1999, 5(1), 33–44, DOI:10.1051/epjap:1999109.
- A. Jouanneaux, Ph Daniel and G. Bushnell-Wye, Structural instabilities in disordered perovskites studied by synchrotron radiation powder diffraction. Proposition for a phase diagram, J. Phys.: Condens. Matter, 1998, 10(24), 5485, DOI:10.1088/0953-8984/10/24/024.
- N. V. Rebrova, A. Y. Grippa, I. A. Boiaryntseva, P. Berastegui, T. E. Gorbacheva, Y. N. Datsko, A.
L. Rebrov, C. Dujardin, R. Calà, L. Martinazzoli, E. Auffray and V. V. Kononets, Effects of europium concentration on luminescent and scintillation performance of Cs0.2Rb0.8Ca1−xEuxBr3 (0 ≤ x ≤ 0.08) crystals, J. Rare Earths, 2022, 40(1), 29–33, DOI:10.1016/j.jre.2020.08.012.
- N. V. Rebrova, A. Y. Grippa, I. A. Boiaryntseva, P. Berastegui, T. E. Gorbacheva, V. Y. Pedash, S. N. Galkin, V. V. Kononets, Y. N. Datsko and V. L. Cherginets, Crystal growth and characterization of Eu2+ doped Cs1−xRbxCaBr3, J. Alloys Compd., 2020, 816, 152594, DOI:10.1016/j.jallcom.2019.152594.
- M. Hidaka, Z. Y. Zhou and S. Yamashita, Structural phase transitions in KCdF3 and K0.5Rb0.5CdF3, Phase Transitions, 1990, 20(1–2), 83–94, DOI:10.1080/01411599008206869.
- S. He, Q. Qiang, T. Lang, M. Cai, T. Han, H. You, L. Peng, S. Cao, B. Liu, X. Jing and B. Jia, Highly Stable Orange-Red Long-Persistent Luminescent CsCdCl3:Mn2+ Perovskite Crystal, Angew. Chem., Int. Ed., 2022, 61(48), e202208937, DOI:10.1002/anie.202208937.
- W. Jia, Q. Wei, S. Yao, S. Ge, C. Peng, L. Wang, X. Zhong, H. peng and B. Zou, Magnetic coupling for highly efficient and tunable emission in CsCdX3:Mn perovskites, J. Lumin., 2023, 257, 119657, DOI:10.1016/j.jlumin.2022.119657.
- S. Wu, L. Yuan, G. Chen, C. Peng and Y. Jin, All-inorganic Mn2+-doped metal halide perovskite crystals for the late-time detection of X-ray afterglow imaging, Nanoscale, 2023, 15(33), 13628–13634, 10.1039/D3NR02208K.
- L. Men, B. A. Rosales, N. E. Gentry, S. D. Cady and J. Vela, Lead-Free Semiconductors: Soft Chemistry, Dimensionality Control, and Manganese-Doping of Germanium Halide Perovskites, ChemNanoMat, 2019, 5(3), 334–339, DOI:10.1002/cnma.201800497.
- A. Ratuszna and J. Kapusta, Structural phase transitions in KMnF3 doped by Li+, Na+ and Rb+, Phase Transitions, 1997, 62(3), 181–198, DOI:10.1080/01411599708220068.
- M. W. Shafer and T. R. McGuire, Preparation and properties of ferrimagnets in the RbMgF3–RbCoF3 system, J. Phys. Chem. Solids, 1969, 30(8), 1989–1997, DOI:10.1016/0022-3697(69)90177-2.
- M. Chen, M.-G. Ju, H. F. Garces, A. D. Carl, L. K. Ono, Z. Hawash, Y. Zhang, T. Shen, Y. Qi, R. L. Grimm, D. Pacifici, X. C. Zeng, Y. Zhou and N. P. Padture, Highly stable and efficient all-inorganic lead-free perovskite solar cells with native-oxide passivation, Nat. Commun., 2019, 10(1), 16, DOI:10.1038/s41467-018-07951-y.
- A. Kama, S. Tirosh, A. Itzhak, M. Ejgenberg and D. Cahen, New Pb-Free Stable Sn–Ge Solid Solution Halide Perovskites Fabricated by Spray Deposition, ACS Appl. Energy Mater., 2022, 5(3), 3638–3646, DOI:10.1021/acsaem.1c04115.
- H. Lv, X. Tang and M. Chen, Ionic Doping of CsPbI3 Perovskite Nanocrystals Improves Luminescence and Stability in Patterned Large-Area Light-Emitting Diodes, ACS Appl. Nano Mater., 2023, 6(20), 18918–18925, DOI:10.1021/acsanm.3c03358.
- W. J. L. Buyers, D. E. Pepper and R. J. Elliott, Theory of spin waves in disordered antiferromagnets. I. Application to (Mn, Co)F2 and K(Mn, Co)F3, J. Phys. C-Solid State Phys., 1972, 5(18), 2611, DOI:10.1088/0022-3719/5/18/011.
- I. P. Dzyub, Cluster Theory of Spin Excitations of Mixed Antiferromagnets. Application to MncCo1−cF2 and KMncCo1−cF3, Phys. Status Solidi B, 1974, 66(1), 339–347, DOI:10.1002/pssb.2220660138.
- H. Komura, M. M. Shapiro and R. Stevenson, Exciton-magnon cross excitation in the mixed substitutional system KMn1−xCoxF3, Phys. Rev. B: Solid State, 1974, 9(8), 3266–3269, DOI:10.1103/PhysRevB.9.3266.
- A. R. Chakhmouradian, K. Ross, R. H. Mitchell and I. Swainson, The crystal chemistry of synthetic potassium-bearing neighborite, (Na1−xKx)MgF3, Phys. Chem. Miner., 2001, 28(4), 277–284, DOI:10.1007/s002690100151.
- P. Chartrand and A. D. Pelton, Thermodynamic evaluation and optimization of the LiF-NaF-KF-MgF2-CaF2 system using the modified quasi-chemical model, Metall. Mater. Trans. A, 2001, 32(6), 1385–1396, DOI:10.1007/s11661-001-0228-1.
- C. D. Martin, S. Chaudhur, C. P. Grey and J. B. Parise, Effect of A-site cation radius on ordering of BX6 octahedra in (K,Na)MgF3 perovskite, Am. Mineral., 2005, 90(10), 1522–1533, DOI:10.2138/am.2005.1693.
- Y. Zhao, Crystal Chemistry and Phase Transitions of Perovskite in P–T–X Space: Data for (KxNa1−x)MgF3 Perovskites, J. Solid State Chem., 1998, 141(1), 121–132, DOI:10.1006/jssc.1998.7927.
- R. E. Schmidt, M. Welsch, S. Kummer-Dörner and D. Babel, Einkristallstrukturuntersuchungen an hexagonalen Fluorperowskiten AMIIF3 (MII
=
Mg, Mn, Fe, Co, Ni), Z. Anorg. Allg. Chem., 1999, 625(4), 637–642, DOI:10.1002/(SICI)1521-3749(199904)625:4<637::AID-ZAAC637>3.0.CO;2-N.
- X. Huang, J. Hu, C. Bi, J. Yuan, Y. Lu, M. Sui and J. Tian, B-site doping of CsPbI3 quantum dot to stabilize the cubic structure for high-efficiency solar cells, Chem. Eng. J., 2021, 421, 127822, DOI:10.1016/j.cej.2020.127822.
- I. D. Skurlov, A. V. Sokolova, D. A. Tatarinov, P. S. Parfenov, D. A. Kurshanov, A. O. Ismagilov, A. V. Koroleva, D. V. Danilov, E. V. Zhizhin, S. V. Mikushev, A. N. Tcypkin, A. V. Fedorov and A. P. Litvin, Engineering the Optical Properties of CsPbBr3 Nanoplatelets through Cd2+ Doping, Materials, 2022, 15(21), 7676, DOI:10.3390/ma15217676.
- I. D. Skurlov, W. Yin, A. O. Ismagilov, A. N. Tcypkin, H. Hua, H. Wang, X. Zhang, A. P. Litvin and W. Zheng, Improved One- and Multiple-Photon Excited Photoluminescence from Cd2+-Doped CsPbBr3 Perovskite NCs, Nanomaterials, 2022, 12(1), 151, DOI:10.3390/nano12010151.
- M. Retuerto, Z. Yin, T. J. Emge, P. W. Stephens, M.-R. Li, T. Sarkar, M. C. Croft, A. Ignatov, Z. Yuan, S. J. Zhang, C. Jin, R. Paria Sena, J. Hadermann, G. Kotliar and M. Greenblatt, Hole Doping and Structural Transformation in CsTl1–xHgxCl3, Inorg. Chem., 2015, 54(3), 1066–1075, DOI:10.1021/ic502400d.
- C.-Q. Lin, M.-L. Liu, Z. Yang, H. Wang and C.-Y. Pan, Mn2+ doped CsPbBr3 perovskite quantum dots with high quantum yield and stability for flexible array displays, J. Solid State Chem., 2023, 327, 124295, DOI:10.1016/j.jssc.2023.124295.
- Z. Yang, X. Yuan, Y. Song, M. Chen, K. Xing, S. Cao, J. Zheng and J. Zhao, Thickness-Dependent Photoluminescence Properties of Mn-Doped CsPbBr3 Perovskite Nanoplatelets Synthesized at Room Temperature, J. Phys. Chem. C, 2023, 127(43), 21227–21234, DOI:10.1021/acs.jpcc.3c05639.
- D. J. Lockwood, G. J. Coombs and R. A. Cowley, Light scattering from the mixed antiferromagnet KNixMn1−xF3, J. Phys. C-Solid State Phys., 1979, 12(21), 4611, DOI:10.1088/0022-3719/12/21/025.
- D. Skrzypek, Critical Behaviour of the Magnetic Resonance in KNixMn1−xF3, Phys. Status Solidi B, 1990, 157(2), 695–700, DOI:10.1002/pssb.2221570222.
- D. Skrzypek, Antiferromagnetic resonance in KNixMn1−xF3 crystals, Phys. B, 1995, 205(3), 279–284, DOI:10.1016/0921-4526(94)00611-X.
- A. Harrison, D. Visser, P. Day, W. Knop and M. Steiner, Magnetic ordering effects in the random singlet-magnetic ground state system Rb(1−x)CsxFeCl3, J. Phys. C-Solid State Phys., 1986, 19(34), 6811, DOI:10.1088/0022-3719/19/34/018.
- A. Ratuszna, The crystal structures of (KxNa1−x)MnF3 perovskite-type compounds, Phase Transitions, 1984, 4(3), 217–223, DOI:10.1080/01411598408218596.
- A. Ratuszna, Determination of structure distortions in KMnF3 doped with Na+, Z. Kristallogr., 1988, 185, 521 Search PubMed.
- A. Ratuszna, P. Daniel, J. Kapusta and M. Rousseau, Experimental evidence for glasslike behavior in a KMnF3: Na+ crystal from x-ray diffraction and Raman scattering, Phys. Rev. B: Condens. Matter Mater. Phys., 1998, 57(17), 10470–10475, DOI:10.1103/PhysRevB.57.10470.
- W. E. Vehse, F. A. Sherrill and C. R. Riley, Lattice Constants for KMg(1−x)MnxF3 Crystals, J. Appl. Phys., 1972, 43(3), 1320–1321, DOI:10.1063/1.1661275.
- N. Elliott and L. Pauling, The Crystal Structure of Cesium Aurous Auric Chloride, Cs2AuAuCl6, and Cesium Argentous Auric Chloride, Cs2AgAuCl6, J. Am. Chem. Soc., 1938, 60(8), 1846–1851, DOI:10.1021/ja01275a037.
- P. Chartrand and A. D. Pelton, Thermodynamic Evaluation and Optimization of the LiCl–NaCl–KCl–RbCl–CsCl–MgCl2–CaCl2–SrCl2–BaCl2 System Using the Modified Quasichemical Model, Can. Metall. Q., 2001, 40(1), 13–32, DOI:10.1179/cmq.2001.40.1.13.
- J. Y. Buzare and P. Foucher, Disorder and phase transitions in Rb1−xKxCaF3: an electron paramagnetic resonance investigation, J. Phys.: Condens. Matter, 1991, 3(15), 2535, DOI:10.1088/0953-8984/3/15/009.
- J. W. Flocken, R. W. Smith, J. R. Hardy, E. S. Stevenson and J. Swearingen, Phase transitions in mixed alkali calcium trifluoride solid solutions, Mater. Res. Bull., 1996, 31(9), 1093–1099, DOI:10.1016/0025-5408(96)00099-2.
- A. Ratuszna, P. Daniel and M. Rousseau, Optical and X-ray evidence of structural phase transitions in mixed (Rb1−xKx)CaF3 crystals, Phase Transitions, 1995, 54(1), 43–59, DOI:10.1080/01411599508200403.
- M. Rousseau, P. Daniel, J. Toulouse and B. Hennion, Evidence of disorder in the perovskite crystals RbCaF3 and Rb0.65K0.35CaF3 investigation of phonon acoustic modes by inelastic neutron scattering, Phys. B, 1997, 234–236, 139–141, DOI:10.1016/S0921-4526(96)00928-3.
- L. Shen, Z. Zhang, Y. Zhao, H. Yang, L. Yuan, Y. Chen, W. Xiang and X. Liang, Synthesis and optical properties of novel mixed-metal cation CsPb1−xTixBr3-based perovskite glasses for W-LED, J. Am. Ceram. Soc., 2020, 103(1), 382–390, DOI:10.1111/jace.16760.
- Z. Wu, Q. Zhang, B. Li, Z. Shi, K. Xu, Y. Chen, Z. Ning and Q. Mi, Stabilizing the CsSnCl3 Perovskite Lattice by B-Site Substitution for Enhanced Light Emission, Chem. Mater., 2019, 31(14), 4999–5004, DOI:10.1021/acs.chemmater.9b00433.
- T. Inami, T. Asano, Y. Ajiro and T. Goto, Magnetization process of the one-dimensional Ising-like antiferromagnet with nonmagnetic impurity, CsCo1−xMgxCl3, Phys. B, 1994, 201, 204–207, DOI:10.1016/0921-4526(94)91084-7.
- H. Ohta, S. Imagawa, M. Motokawa and H. Ikeda, Electron Paramagnetic Resonance of CsCoxMg1−xCl3 and the Determination of Exchange Interactions, J. Phys. Soc. Jpn., 1993, 62(7), 2481–2489, DOI:10.1143/JPSJ.62.2481.
- E. Mei, Y. Chen, Y. Chen, Q. He, Y. Tong, P. Yu, X. Liang and W. Xiang, Ba-doped CsPbBr3 with high quantum efficiency for wide color gamut on white light-emitting diodes, Appl. Phys. Lett., 2021, 119(25), 251103, DOI:10.1063/5.0070326.
- H. Liu, Z. Wu, J. Shao, D. Yao, H. Gao, Y. Liu, W. Yu, H. Zhang and B. Yang, CsPbxMn1–xCl3 Perovskite Quantum Dots with High Mn Substitution Ratio, ACS Nano, 2017, 11(2), 2239–2247, DOI:10.1021/acsnano.6b08747.
- X. Zhang, F. Wang, Y. Wang, X. Wu, Q. Ou and S. Zhang, Boosting the Photoluminescence Quantum Yield and Stability of Lead-Free CsEuCl3 Nanocrystals via Ni2+ Doping, J. Phys. Chem. Lett., 2023, 14(24), 5580–5585, DOI:10.1021/acs.jpclett.3c01046.
- Y. Hu, X. Zhang, C. Yang, J. Li and L. Wang, Fe2+ doped in CsPbCl3 perovskite nanocrystals: impact on the luminescence and magnetic properties, RSC Adv., 2019, 9(57), 33017–33022, 10.1039/C9RA07069A.
- G. Elbinger, A. Funke, P. Kleinert, P. Rosemann and W. Keilig, Präparation und Eigenschaften von Metallfluoridverbindungen des Typs MeIMeIIF3, Z. Anorg. Allg. Chem., 1972, 393(3), 193–206, DOI:10.1002/zaac.19723930302.
- W. Shi, X. Zhang, H. S. Chen, K. Matras-Postolek and P. Yang, Transition metal halide derived phase transition from Cs4PbCl6 to CsPbxM1–xX3 for bright white light-emitting diodes, J. Mater. Chem. C, 2021, 9(17), 5732–5739, 10.1039/D1TC01150B.
- R. B. Rogge, Y. S. Yang, Z. Tun, B. D. Gaulin, J. A. Fernandez-Baca, R. M. Nicklow and A. Harrison, A neutron scattering study of the quasi-one-dimensional, dilute Ising-like antiferromagnet CsCo0.83Mg0.17Br3, J. Appl. Phys., 1993, 73(10), 6451–6453, DOI:10.1063/1.352630.
- J. van Duijn, B. D. Gaulin, M. A. Lumsden, J. P. Castellan and W. J. L. Buyers, Random Fields and the Partially Paramagnetic State of CsCo0.83Mg0.17Br3: Critical Scattering Study, Phys. Rev. Lett., 2004, 92(7), 077202, DOI:10.1103/PhysRevLett.92.077202.
- Y. S. Yang, F. Marsiglio, M. Madsen, B. D. Gaulin, R. B. Rogge and J. A. Fernandez-Baca, Spin-wave response in the dilute quasi-one-dimensional Ising-like antiferromagnet CsCo0.83Mg0.17Br3, Phys. Rev. B: Condens. Matter Mater. Phys., 2002, 65(21), 212408, DOI:10.1103/PhysRevB.65.212408.
- J.-S. Yao, J. Ge, B.-N. Han, K.-H. Wang, H.-B. Yao, H.-L. Yu, J.-H. Li, B.-S. Zhu, J.-Z. Song, C. Chen, Q. Zhang, H.-B. Zeng, Y. Luo and S.-H. Yu, Ce3+-Doping to Modulate Photoluminescence Kinetics for Efficient CsPbBr3 Nanocrystals Based Light-Emitting Diodes, J. Am. Chem. Soc., 2018, 140(10), 3626–3634, DOI:10.1021/jacs.7b11955.
- R. Chen, Y. Xu, S. Wang, C. Xia, Y. Liu, B. Yu, T. Xuan and H. Li, Zinc ions doped cesium lead bromide perovskite nanocrystals with enhanced efficiency and stability for white light-emitting diodes, J. Alloys Compd., 2021, 866, 158969, DOI:10.1016/j.jallcom.2021.158969.
- K. Cui, Y. Wen, X. Han, Z. Hao, J. Zhang and J. Xie, Intense blue emission from one-pot synthesized quaternary CsZnxPb1−xBr3 perovskite quantum dots, Opt. Mater., 2023, 136, 113441, DOI:10.1016/j.optmat.2023.113441.
- P.-N. Tran, H.-H. Phan, T.-N. Luu, Q.-H. Tran and T.-T. Duong, Optimizing the single-source flash thermal evaporation process of Zn-doped CsPbBr3 films for enhanced performance in perovskite LEDs, Appl. Phys. A: Mater. Sci. Process., 2023, 130(1), 20, DOI:10.1007/s00339-023-07179-8.
- X. Shen, Y. Zhang, S. V. Kershaw, T. Li, C. Wang, X. Zhang, W. Wang, D. Li, Y. Wang, M. Lu, L. Zhang, C. Sun, D. Zhao, G. Qin, X. Bai, W. W. Yu and A. L. Rogach, Zn-Alloyed CsPbI3 Nanocrystals for Highly Efficient Perovskite Light-Emitting Devices, Nano Lett., 2019, 19(3), 1552–1559, DOI:10.1021/acs.nanolett.8b04339.
- Y. Wu, Q. Li, B. C. Chakoumakos, M. Zhuravleva, A. C. Lindsey, J. A. Johnson II, L. Stand, M. Koschan and C. L. Melcher, Quaternary Iodide K(Ca,Sr)I3:Eu2+ Single-Crystal Scintillators for Radiation Detection: Crystal Structure, Electronic Structure, and Optical and Scintillation Properties, Adv. Opt. Mater., 2016, 4(10), 1518–1532, DOI:10.1002/adom.201600239.
- Y. Wu, M. Zhuravleva, A. C. Lindsey, M. Koschan and C. L. Melcher, Eu2+ concentration effects in KCa0.8Sr0.2I3:Eu2+: A novel high-performance scintillator, Nucl. Instrum. Methods Phys. Res., Sect. A, 2016, 820, 132–140, DOI:10.1016/j.nima.2016.03.027.
- M. Saliba, T. Matsui, K. Domanski, J.-Y. Seo, A. Ummadisingu, S. M. Zakeeruddin, J.-P. Correa-Baena, W. R. Tress, A. Abate, A. Hagfeldt and M. Grätzel, Incorporation of rubidium cations into perovskite solar cells improves photovoltaic performance, Science, 2016, 354(6309), 206–209, DOI:10.1126/science.aah5557.
- M. Saliba, T. Matsui, J.-Y. Seo, K. Domanski, J.-P. Correa-Baena, M. K. Nazeeruddin, S. M. Zakeeruddin, W. Tress, A. Abate, A. Hagfeldt and M. Grätzel, Cesium-containing triple cation perovskite solar cells: improved stability, reproducibility and high efficiency, Energy Environ. Sci., 2016, 9(6), 1989–1997, 10.1039/C5EE03874J.
- S. P. Senanayak, M. Abdi-Jalebi, V. S. Kamboj, R. Carey, R. Shivanna, T. Tian, G. Schweicher, J. Wang, N. Giesbrecht, D. Di Nuzzo, H. E. Beere, P. Docampo, D. A. Ritchie, D. Fairen-Jimenez, R. H. Friend and H. Sirringhaus, A general approach for hysteresis-free, operationally stable metal halide perovskite field-effect transistors, Sci. Adv., 2020, 6(15), eaaz4948, DOI:10.1126/sciadv.aaz4948.
- S.-Y. Kim, H.-C. Lee, Y. Nam, Y. Yun, S.-H. Lee, D. H. Kim, J. H. Noh, J.-H. Lee, D.-H. Kim, S. Lee and Y.-W. Heo, Ternary diagrams of the phase, optical bandgap energy and photoluminescence of mixed-halide perovskites, Acta Mater., 2019, 181, 460–469, DOI:10.1016/j.actamat.2019.10.008.
- F. Meng, X. Liu, X. Cai, Z. Gong, B. Li, W. Xie, M. Li, D. Chen, H.-L. Yip and S.-J. Su, Incorporation of rubidium cations into blue perovskite quantum dot light-emitting diodes via FABr-modified multi-cation hot-injection method, Nanoscale, 2019, 11(3), 1295–1303, 10.1039/C8NR07907B.
- R. K. Gunasekaran, J. Jung, S. W. Yang, J. Yun, Y. Yun, D. Vidyasagar, W. C. Choi, C.-L. Lee, J. H. Noh, D. H. Kim and S. Lee, High-throughput compositional mapping of triple-cation tin–lead perovskites for high-efficiency solar cells, InfoMat, 2023, 5(4), e12393, DOI:10.1002/inf2.12393.
- C. Otero-Martínez, M. Imran, N. J. Schrenker, J. Ye, K. Ji, A. Rao, S. D. Stranks, R. L. Z. Hoye, S. Bals, L. Manna, J. Pérez-Juste and L. Polavarapu, Fast A-Site Cation Cross-Exchange at Room Temperature: Single-to Double- and Triple-Cation Halide Perovskite Nanocrystals, Angew. Chem., Int. Ed., 2022, 61(34), e202205617, DOI:10.1002/anie.202205617.
- A. F. Palmstrom, G. E. Eperon, T. Leijtens, R. Prasanna, S. N. Habisreutinger, W. Nemeth, E. A. Gaulding, S. P. Dunfield, M. Reese, S. Nanayakkara, T. Moot, J. Werner, J. Liu, B. To, S. T. Christensen, M. D. McGehee, M. F. A. M. van Hest, J. M. Luther, J. J. Berry and D. T. Moore, Enabling Flexible All-Perovskite Tandem Solar Cells, Joule, 2019, 3(9), 2193–2204, DOI:10.1016/j.joule.2019.05.009.
- L. Gao, Y. Zhang, L. Gou, Q. Wang, M. Wang, W. Zheng, Y. Wang, H.-L. Yip and J. Zhang, High efficiency pure blue perovskite quantum dot light-emitting diodes based on formamidinium manipulating carrier dynamics and electron state filling, Light: Sci. Appl., 2022, 11(1), 346, DOI:10.1038/s41377-022-00992-5.
- T. Nakamura, K. Otsuka, S. Hu, R. Hashimoto, T. Morishita, T. Handa, T. Yamada, M. A. Truong, R. Murdey, Y. Kanemitsu and A. Wakamiya, Composition–Property Mapping in Bromide-Containing Tin Perovskite Using High-Purity Starting Materials, ACS Appl. Energy Mater., 2022, 5(12), 14789–14798, DOI:10.1021/acsaem.2c02144.
- T. Jesper Jacobsson, J.-P. Correa-Baena, M. Pazoki, M. Saliba, K. Schenk, M. Grätzel and A. Hagfeldt, Exploration of the compositional space for mixed lead halogen perovskites for high efficiency solar cells, Energy Environ. Sci., 2016, 9(5), 1706–1724, 10.1039/C6EE00030D.
- I. Susic, A. Kama, L. Gil-Escrig, C. Dreessen, F. Palazon, D. Cahen, M. Sessolo and H. J. Bolink, Combinatorial Vacuum-Deposition of Wide Bandgap Perovskite Films and Solar Cells, Adv. Mater. Interfaces, 2023, 10(4), 2202271, DOI:10.1002/admi.202202271.
- H. Xiao, H. Xiong, P. Li, L. Jiang, A. Yang, L. Lin, Z. Kang, Q. Yan and Y. Qiu, Tunable deep-blue luminescence from ball-milled chlorine-rich Csx(NH4)1−xPbCl2Br nanocrystals by ammonium modulation, Chem. Commun., 2022, 58(23), 3827–3830, 10.1039/D1CC07125D.
- C. Wang, Y. Liu, X. Feng, C. Zhou, Y. Liu, X. Yu and G. Zhao, Phase Regulation Strategy of Perovskite Nanocrystals from 1D Orthomorphic NH4PbI3 to 3D Cubic (NH4)0.5Cs0.5Pb(I0.5Br0.5)3 Phase Enhances Photoluminescence, Angew. Chem., Int. Ed., 2019, 58(34), 11642–11646, DOI:10.1002/anie.201903121.
- S. Terada, T. Oku, A. Suzuki, M. Okita, S. Fukunishi, T. Tachikawa and T. Hasegawa, Ethylammonium Bromide- and Potassium-Added CH3NH3PbI3 Perovskite Solar Cells, Photonics, 2022, 9(11), 791, DOI:10.3390/photonics9110791.
-
S. L. Sanchez, E. Foadian, M. Ziatdinov, J. Yang, S. V. Kalinin, Y. Liu and M. Ahmadi, Physics-driven discovery and bandgap engineering of hybrid perovskites, arXiv, 2023, preprint, arXiv:2310.06583 DOI:10.48550/arXiv.2310.06583.
- A. Gabriel Tomulescu, L. Nicoleta Leonat, F. Neatu, V. Stancu, V. Toma, S. Derbali, S. Neatu, A. Mihai Rostas, C. Besleaga, R. Pătru, I. Pintilie and M. Florea, Enhancing stability of hybrid perovskite solar cells by imidazolium incorporation, Sol. Energy Mater. Sol. Cells, 2021, 227, 111096, DOI:10.1016/j.solmat.2021.111096.
- R. K. Singh, P. Sharma, C.-H. Lu, R. Kumar, N. Jain and J. Singh, Structural, morphological and thermodynamic parameters investigation of tunable MAPb1−xCdxBr3−2xI2x hybrid perovskite, J. Alloys Compd., 2021, 866, 158936, DOI:10.1016/j.jallcom.2021.158936.
- R. K. Singh, P. Sharma, R. Kumar, S. Som, S. Dutta, N. Jain, R. Chaurasiya, M. L. Meena, J.-S. Ho, S.-W. Dai, J. Singh, C.-H. Lu and H.-W. Lin, CH3NH3Pb1–xCoxBr3–2xCl2x Perovskite Quantum Dots for Wide-Color Backlighting, ACS Appl. Nano Mater., 2021, 4(1), 717–728, DOI:10.1021/acsanm.0c03019.
- J. Xun, J. Deng, W. Shen, M. Li and R. He, Highly efficient green-emitting nanocrystals of MAPb1−xMnxBr3 perovskite with excellent thermal stability, Opt. Mater., 2021, 122, 111799, DOI:10.1016/j.optmat.2021.111799.
- C. Lu, J. Zhou, C. Tang, Q. Dai, Y. Peng, W. Lv, L. Sun, S. Xu and W. Hu, Ultranarrow-band filterless photodetectors based on CH3NH3PbClxBr3–x mixed-halide perovskite single crystals, Nanotechnology, 2023, 34(34), 345705, DOI:10.1088/1361-6528/acd944.
- M. Mazurin, A. Shelestova, D. Tsvetkov, V. Sereda, I. Ivanov, D. Malyshkin and A. Zuev, Thermochemical Study of CH3NH3Pb(Cl1−xBrx)3 Solid Solutions, Materials, 2022, 15(21), 7675, DOI:10.3390/ma15217675.
- Y. Pan, X. Wang, Y. Xu, S. Chai, J. Wu, Z. Zhao, Q. Li, J. Wu, J. Chen, Z. Zhu, B. S. Bae, O. E. Fayemi, J. Zhou, Y. Zhu and W. Lei, Epitaxy growth of MAPbBrxCl3−x single-crystalline perovskite films toward spectral selective detection in both broadband and narrowband ranges, J. Mater. Chem. C, 2023, 11(40), 13763–13773, 10.1039/D3TC02300A.
- A. Rajagopal, R. J. Stoddard, H. W. Hillhouse and A. K. Y. Jen, On understanding bandgap bowing and optoelectronic quality in Pb–Sn alloy hybrid perovskites, J. Mater. Chem. A, 2019, 7(27), 16285–16293, 10.1039/C9TA05308E.
- F.-X. Liang, L.-L. Zhou, Y. Hu, S.-F. Li, Z.-Y. Zhang, J.-Y. Li, C. Fu, C.-Y. Wu, L. Wang, J.-A. Huang and L.-B. Luo, Self-Driven Narrow-Band Photodetector based on FAPbBr2.5I0.5 Single Crystal for Yellow Light Intensity Meter Application, Adv. Funct. Mater., 2023, 33(36), 2302175, DOI:10.1002/adfm.202302175.
- J. H. Noh, S. H. Im, J. H. Heo, T. N. Mandal and S. I. Seok, Chemical Management for Colorful, Efficient, and Stable Inorganic–Organic Hybrid Nanostructured Solar Cells, Nano Lett., 2013, 13(4), 1764–1769, DOI:10.1021/nl400349b.
- S. Hu, K. Otsuka, R. Murdey, T. Nakamura, M. A. Truong, T. Yamada, T. Handa, K. Matsuda, K. Nakano, A. Sato, K. Marumoto, K. Tajima, Y. Kanemitsu and A. Wakamiya, Optimized carrier extraction at interfaces for 23.6% efficient tin–lead perovskite solar cells, Energy Environ. Sci., 2022, 15(5), 2096–2107, 10.1039/D2EE00288D.
- S.-H. Turren-Cruz, J. Pascual, S. Hu, J. Sanchez-Diaz, S. Galve-Lahoz, W. Liu, W. Hempel, V. S. Chirvony, J. P. Martinez-Pastor, P. P. Boix, A. Wakamiya and I. Mora-Seró, Multicomponent Approach for Stable Methylammonium-Free Tin–Lead Perovskite Solar Cells, ACS Energy Lett., 2024, 9(2), 432–441, DOI:10.1021/acsenergylett.3c02426.
- H. Zhang, Z. Bi, Z. Zhai, H. Gao, Y. Liu, M. Jin, M. Ye, X. Li, H. Liu, Y. Zhang, X. Li, H. Tan, Y. Xu and L. Yang, Revealing Unusual Bandgap Shifts with Temperature and Bandgap Renormalization Effect in Phase-Stabilized Metal Halide Perovskite Thin Films, Adv. Funct. Mater., 2024, 34(9), 2302214, DOI:10.1002/adfm.202302214.
- R. Prasanna, A. Gold-Parker, T. Leijtens, B. Conings, A. Babayigit, H.-G. Boyen, M. F. Toney and M. D. McGehee, Band Gap Tuning via Lattice Contraction and Octahedral Tilting in Perovskite Materials for Photovoltaics, J. Am. Chem. Soc., 2017, 139(32), 11117–11124, DOI:10.1021/jacs.7b04981.
- J. Wang, M. A. Uddin, B. Chen, X. Ying, Z. Ni, Y. Zhou, M. Li, M. Wang, Z. Yu and J. Huang, Enhancing Photostability of Sn-Pb Perovskite Solar Cells by an Alkylammonium Pseudo-Halogen Additive, Adv. Energy Mater., 2023, 13(15), 2204115, DOI:10.1002/aenm.202204115.
- J. Guo, Y. Fu, W. Zheng, M. Xie, Y. Huang, Z. Miao, C. Han, W. Yin, J. Zhang, X. Yang, J. Tian and X. Zhang, Entropy-Driven Strongly Confined Low-Toxicity Pure-Red Perovskite Quantum Dots for Spectrally Stable Light-Emitting Diodes, Nano Lett., 2024, 24(1), 417–423, DOI:10.1021/acs.nanolett.3c04214.
- K.-C. Hsiao, C.-M. Ho, T.-H. Lin, S.-H. Chen, Y.-H. Chang, Y.-H. Liao, J.-M. Chang, T.-F. Lin, Y.-C. Huang, K.-M. Lee and M.-C. Wu, Ceiling of Barium Substitution for B-Site Cation in Organometal Halide Perovskite Solar Cells, Int. J. Energy Res., 2024, 2024, 9990559, DOI:10.1155/2024/9990559.
- I. Susic, L. Gil-Escrig, K. P. S. Zanoni, C. Roldán-Carmona, M. Sessolo and H. J. Bolink, Pure Iodide Multication Wide Bandgap Perovskites by Vacuum Deposition, ACS Mater. Lett., 2023, 5(12), 3299–3305, DOI:10.1021/acsmaterialslett.3c01094.
- S. Derbali, K. Nouneh, M. Florea, L. N. Leonat, V. Stancu, A. G. Tomulescu, A. C. Galca, M. Secu, L. Pintilie and M. E. Touhami, Potassium-containing triple-cation mixed-halide perovskite materials: Toward efficient and stable solar cells, J. Alloys Compd., 2021, 858, 158335, DOI:10.1016/j.jallcom.2020.158335.
- I. Ono, T. Oku, A. Suzuki, S. Fukunishi, T. Tachikawa and T. Hasegawa, Effects of ethylammonium and rubidium addition to guanidinium-based CH3NH3PbI3 perovskite photovoltaic devices prepared at 190 °C in ambient air, Mater. Today Commun., 2024, 38, 107623, DOI:10.1016/j.mtcomm.2023.107623.
- F. B. Minussi, L. A. Silva and E. B. Araújo, Structure, optoelectronic properties and thermal stability of the triple organic cation GAxFAxMA1−2xPbI3 system prepared by mechanochemical synthesis, Phys. Chem. Chem. Phys., 2022, 24(8), 4715–4728, 10.1039/D1CP04977A.
- F. B. Minussi, R. M. Silva Jr. and E. B. Araújo, Composition-Property Relations for GAxFAyMA1−x−yPbI3 Perovskites, Small, 2024, 20(7), 2305054, DOI:10.1002/smll.202305054.
- S. Wang, S. Pang, D. Chen, W. Zhu, H. Xi and C. Zhang, Improving perovskite solar cell performance by compositional engineering via triple-mixed cations, Sol. Energy, 2021, 220, 412–417, DOI:10.1016/j.solener.2021.03.036.
- M. Badrooj, F. Jamali-Sheini and N. Torabi, Zn-doped Pb/Sn hybrid perovskite solar cells: Towards high photovoltaic performance, Sol. Energy, 2022, 236, 63–74, DOI:10.1016/j.solener.2022.02.034.
- T. Soto-Montero, S. Kralj, W. Soltanpoor, J. S. Solomon, J. S. Gómez, K. P. S. Zanoni, A. Paliwal, H. J. Bolink, C. Baeumer, A. P. M. Kentgens and M. Morales-Masis, Single-Source Vapor-Deposition of MA1−xFAxPbI3 Perovskite Absorbers for Solar Cells, Adv. Funct. Mater., 2023, 2300588, DOI:10.1002/adfm.202300588.
- O. J. Weber, B. Charles and M. T. Weller, Phase behaviour and composition in the formamidinium–methylammonium hybrid lead iodide perovskite solid solution, J. Mater. Chem. A, 2016, 4(40), 15375–15382, 10.1039/C6TA06607K.
- C. Zhao, C. Cazorla, X. Zhang, H. Huang, X. Zhao, D. Li, J. Shi, Q. Zhao, W. Ma and J. Yuan, Fast Organic Cation Exchange in Colloidal Perovskite Quantum Dots toward Functional Optoelectronic Applications, J. Am. Chem. Soc., 2024, 146(7), 4913–4921, DOI:10.1021/jacs.3c14000.
- D. Ju, Y. Dang, Z. Zhu, H. Liu, C.-C. Chueh, X. Li, L. Wang, X. Hu, A. K. Y. Jen and X. Tao, Tunable Band Gap and Long Carrier Recombination Lifetime of Stable Mixed CH3NH3PbxSn1−xBr3 Single Crystals, Chem. Mater., 2018, 30(5), 1556–1565, DOI:10.1021/acs.chemmater.7b04565.
- S. Kahmann, Z. Chen, O. Hordiichuk, O. Nazarenko, S. Shao, M. V. Kovalenko, G. R. Blake, S. Tao and M. A. Loi, Compositional Variation in FAPb1–xSnxI3 and Its Impact on the Electronic Structure: A Combined Density Functional Theory and Experimental Study, ACS Appl. Mater. Interfaces, 2022, 14(30), 34253–34261, DOI:10.1021/acsami.2c00889.
- X. Lü, C. Stoumpos, Q. Hu, X. Ma, D. Zhang, S. Guo, J. Hoffman, K. Bu, X. Guo, Y. Wang, C. Ji, H. Chen, H. Xu, Q. Jia, W. Yang, M. G. Kanatzidis and H.-K. Mao, Regulating off-centering distortion maximizes photoluminescence in halide perovskites, Natl. Sci. Rev., 2020, 8(9), nwaa288, DOI:10.1093/nsr/nwaa288.
- S. S. H. Dintakurti, D. Walker, T. A. Bird, Y. Fang, T. White and J. V. Hanna, A powder XRD, solid state NMR and calorimetric study of the phase evolution in mechanochemically synthesized dual cation (Csx(CH3NH3)1−x)PbX3 lead halide perovskite systems, Phys. Chem. Chem. Phys., 2022, 24(30), 18004–18021, 10.1039/D2CP02131E.
- M. Chen, Q. Dong, C. Xiao, X. Zheng, Z. Dai, Y. Shi, J. M. Luther and N. P. Padture, Lead-Free Flexible Perovskite Solar Cells with Interfacial Native Oxide Have >10% Efficiency and Simultaneously Enhanced Stability and Reliability, ACS Energy Lett., 2022, 7(7), 2256–2264, DOI:10.1021/acsenergylett.2c01130.
- F. Hao, C. C. Stoumpos, R. P. H. Chang and M. G. Kanatzidis, Anomalous Band Gap Behavior in Mixed Sn and Pb Perovskites Enables Broadening of Absorption Spectrum in Solar Cells, J. Am. Chem. Soc., 2014, 136(22), 8094–8099, DOI:10.1021/ja5033259.
- J. Im, C. C. Stoumpos, H. Jin, A. J. Freeman and M. G. Kanatzidis, Antagonism between Spin–Orbit Coupling and Steric Effects Causes Anomalous Band Gap Evolution in the Perovskite Photovoltaic Materials CH3NH3Sn1–xPbxI3, J. Phys. Chem. Lett., 2015, 6(17), 3503–3509, DOI:10.1021/acs.jpclett.5b01738.
- Y. Chu, C. Wang, L. Ma, X. Feng, B. Wang, Y. Wu, Y. Jia, M. Zhang, Y. Sun, H. Zhang and G. Zhao, Unveiling the photoluminescence regulation of colloidal perovskite quantum dots via defect passivation and lattice distortion by potassium cations doping: Not the more the better, J. Colloid Interface Sci., 2021, 596, 199–205, DOI:10.1016/j.jcis.2021.03.128.
- A. Ali, H. Park, R. Mall, B. Aïssa, S. Sanvito, H. Bensmail, A. Belaidi and F. El-Mellouhi, Machine Learning Accelerated Recovery of the Cubic Structure in Mixed-Cation Perovskite Thin Films, Chem. Mater., 2020, 32(7), 2998–3006, DOI:10.1021/acs.chemmater.9b05342.
- D. Jia, J. Chen, R. Zhuang, Y. Hua and X. Zhang, Antisolvent-Assisted In Situ Cation Exchange of Perovskite Quantum Dots for Efficient Solar Cells, Adv. Mater., 2023, 35(21), 2212160, DOI:10.1002/adma.202212160.
- F.-C. Liang, F.-C. Jhuang, Y.-H. Fang, J.-S. Benas, W.-C. Chen, Z.-L. Yan, W.-C. Lin, C.-J. Su, Y. Sato, T. Chiba, J. Kido and C.-C. Kuo, Synergistic Effect of Cation Composition Engineering of Hybrid Cs1−xFAxPbBr3 Nanocrystals for Self-Healing Electronics Application, Adv. Mater., 2023, 35(9), 2207617, DOI:10.1002/adma.202207617.
-
A. Pisanu, Chemical tuning of hybrid perovskites for solar-driven clean energy technologies, PhD Thesis, Universita’ di Pavia, 2020.
- S. Nagane, D. Ghosh, R. L. Z. Hoye, B. Zhao, S. Ahmad, A. B. Walker, M. S. Islam, S. Ogale and A. Sadhanala, Lead-Free Perovskite Semiconductors Based on Germanium–Tin Solid Solutions: Structural and Optoelectronic Properties, J. Phys. Chem. C, 2018, 122(11), 5940–5947, DOI:10.1021/acs.jpcc.8b00480.
- Y. Liu, Y.-P. Gong, S. Geng, M.-L. Feng, D. Manidaki, Z. Deng, C. C. Stoumpos, P. Canepa, Z. Xiao, W.-X. Zhang and L. Mao, Hybrid Germanium Bromide Perovskites with Tunable Second Harmonic Generation, Angew. Chem., Int. Ed., 2022, 61(43), e202208875, DOI:10.1002/anie.202208875.
- J. Liu, H. Fu, Z. Du, D. Ou, S. Li, Q. Chen, W. Yang, J. Zhao and J. Zheng, Enhanced photothermal stability of in situ grown FAPbBr3 nanocrystals in polyvinylidene fluoride by incorporation of Cd2+ ions, J. Mater. Chem. C, 2022, 10(46), 17512–17520, 10.1039/D2TC04100F.
- T. Oku, S. Uchiya, R. Okumura, A. Suzuki, I. Ono, S. Fukunishi, T. Tachikawa and T. Hasegawa, Effects of Co-Addition of Guanidinium and Cesium to CH3NH3PbI3 Perovskite Solar Cells, Inorganics, 2023, 11(7), 273, DOI:10.3390/inorganics11070273.
- J. Deng, J. Xun, Y. Qin, M. Li and R. He, Blue-emitting NH4+-doped MAPbBr3 perovskite quantum dots with near unity quantum yield and super stability, Chem. Commun., 2020, 56(79), 11863–11866, 10.1039/D0CC04912C.
- C.-H. Lu, R. K. Singh, T.-Y. Chen, S. Som, R. Kumar, S. A. Lu and M. L. Meena, Rapid synthesis and theoretical analysis of CH3NH3Pb1−xCdxBr3 perovskite quantum dots for backlight LEDs: A step towards enhanced stability, Org. Electron., 2022, 102, 106444, DOI:10.1016/j.orgel.2022.106444.
- M. T. Klug, A. Osherov, A. A. Haghighirad, S. D. Stranks, P. R. Brown, S. Bai, J. T. W. Wang, X. Dang, V. Bulović, H. J. Snaith and A. M. Belcher, Tailoring metal halide perovskites through metal substitution: influence on photovoltaic and material properties, Energy Environ. Sci., 2017, 10(1), 236–246, 10.1039/C6EE03201J.
- J. Yu, H. Xu, L. Wu, Q. Han and W. Wu, Highly sensitive photodetector of Zn/Bi doped MAPbBr3 single crystals formed homojunction, Mater. Sci. Semicond. Process., 2022, 149, 106824, DOI:10.1016/j.mssp.2022.106824.
- Z. Zhou, J. Xu, Y. Liu, C. Wei, H. Zhang and Q. Wang, Zn-alloyed MAPbBr3 crystals with improved thermoelectric and photocatalytic properties, Mater. Chem. Front., 2021, 5(24), 8319–8332, 10.1039/D1QM00993A.
- A. E. Abd El-Samad, N. Gad, M. El-Aasser, M. M. Rashad and A. Mourtada Elseman, Optoelectronic investigation and simulation study of zinc and cobalt doped lead halide perovskite nanocrystals, Sol. Energy, 2022, 247, 553–563, DOI:10.1016/j.solener.2022.10.061.
- S. L. Roscoe and H. M. Haendler, Synthesis of fluorometallates in methanol. The solid solution NH4MnF3–NH4ZnF3, Inorg. Chim. Acta, 1967, 1, 73–75, DOI:10.1016/S0020-1693(00)93142-6.
- B. Jiang, Y. Yu, H. Chen, J. Cui, X. Liu, L. Xie and J. He, Entropy engineering promotes thermoelectric performance in p-type chalcogenides, Nat. Commun., 2021, 12(1), 3234, DOI:10.1038/s41467-021-23569-z.
- A. Gold-Parker, P. M. Gehring, J. M. Skelton, I. C. Smith, D. Parshall, J. M. Frost, H. I. Karunadasa, A. Walsh and M. F. Toney, Acoustic phonon lifetimes limit thermal transport in methylammonium lead iodide, Proc. Natl. Acad. Sci. U. S. A., 2018, 115(47), 11905–11910, DOI:10.1073/pnas.1812227115.
- J. M. Frost and A. Walsh, What Is Moving in Hybrid Halide Perovskite Solar Cells?, Acc. Chem. Res., 2016, 49(3), 528–535, DOI:10.1021/acs.accounts.5b00431.
- C. Katan, A. D. Mohite and J. Even, Entropy in halide perovskites, Nat. Mater., 2018, 17(5), 377–379, DOI:10.1038/s41563-018-0070-0.
- S. A. Cuthriell, S. Panuganti, C. C. Laing, M. A. Quintero, B. Guzelturk, N. Yazdani, B. Traore, A. Brumberg, C. D. Malliakas, A. M. Lindenberg, V. Wood, C. Katan, J. Even, X. Zhang, M. G. Kanatzidis and R. D. Schaller, Nonequilibrium Lattice Dynamics in Photoexcited 2D Perovskites, Adv. Mater., 2022, 34(44), 2202709, DOI:10.1002/adma.202202709.
-
H. Seiler, D. Zahn, V. C. A. Taylor, M. I. Bodnarchnuk, Y. W. Windsor, M. V. Kovalenko and R. Ernstorfer, Direct observation of ultrafast lattice distortions during exciton-polaron formation in lead-halide perovskite nanocrystals, arXiv, 2022, preprint, arXiv:2209.05931 DOI:10.48550/arXiv.2209.05931.
- R. Nie, R. R. Sumukam, S. H. Reddy, M. Banavoth and S. I. Seok, Lead-free
perovskite solar cells enabled by hetero-valent substitutes, Energy Environ. Sci., 2020, 13(8), 2363–2385, 10.1039/D0EE01153C.
- J. Endres, D. A. Egger, M. Kulbak, R. A. Kerner, L. Zhao, S. H. Silver, G. Hodes, B. P. Rand, D. Cahen, L. Kronik and A. Kahn, Valence and Conduction Band Densities of States of Metal Halide Perovskites: A Combined Experimental–Theoretical Study, J. Phys. Chem. Lett., 2016, 7(14), 2722–2729, DOI:10.1021/acs.jpclett.6b00946.
- X. Liu, D. Luo, Z.-H. Lu, J. S. Yun, M. Saliba, S. I. Seok and W. Zhang, Stabilization of photoactive phases for perovskite photovoltaics, Nat. Rev. Chem., 2023, 7(7), 462–479, DOI:10.1038/s41570-023-00492-z.
- S. P. Dunfield, L. Bliss, F. Zhang, J. M. Luther, K. Zhu, M. F. A. M. van Hest, M. O. Reese and J. J. Berry, From Defects to Degradation: A Mechanistic Understanding of Degradation in Perovskite Solar Cell Devices and Modules, Adv. Energy Mater., 2020, 10(26), 1904054, DOI:10.1002/aenm.201904054.
- W. A. Saidi, W. Shadid and I. E. Castelli, Machine-learning structural and electronic properties of metal halide perovskites using a hierarchical convolutional neural network, npj Comput. Mater., 2020, 6(1), 36, DOI:10.1038/s41524-020-0307-8.
- C. Yi, J. Luo, S. Meloni, A. Boziki, N. Ashari-Astani, C. Grätzel, S. M. Zakeeruddin, U. Röthlisberger and M. Grätzel, Entropic stabilization of mixed A-cation ABX3 metal halide perovskites for high performance perovskite solar cells, Energy Environ. Sci., 2016, 9(2), 656–662, 10.1039/C5EE03255E.
- Y.-H. Kim, S. Kim, A. Kakekhani, J. Park, J. Park, Y.-H. Lee, H. Xu, S. Nagane, R. B. Wexler, D.-H. Kim, S. H. Jo, L. Martínez-Sarti, P. Tan, A. Sadhanala, G.-S. Park, Y.-W. Kim, B. Hu, H. J. Bolink, S. Yoo, R. H. Friend, A. M. Rappe and T.-W. Lee, Comprehensive defect suppression in perovskite nanocrystals for high-efficiency light-emitting diodes, Nat. Photonics, 2021, 15(2), 148–155, DOI:10.1038/s41566-020-00732-4.
- I. M. Pavlovetc, M. C. Brennan, S. Draguta, A. Ruth, T. Moot, J. A. Christians, K. Aleshire, S. P. Harvey, S. Toso, S. U. Nanayakkara, J. Messinger, J. M. Luther and M. Kuno, Suppressing Cation Migration in Triple-Cation Lead Halide Perovskites, ACS Energy Lett., 2020, 5(9), 2802–2810, DOI:10.1021/acsenergylett.0c01207.
- S. Kim, T. Eom, Y.-S. Ha, K.-H. Hong and H. Kim, Thermodynamics of Multicomponent Perovskites: A Guide to Highly Efficient and Stable Solar Cell Materials, Chem. Mater., 2020, 32(10), 4265–4272, DOI:10.1021/acs.chemmater.0c00893.
- M. C. Folgueras, Y. Jiang, J. Jin and P. Yang, High-entropy halide perovskite single crystals stabilized by mild chemistry, Nature, 2023, 621, 282–288, DOI:10.1038/s41586-023-06396-8.
- K. Jayanthi, I. Spanopoulos, N. Zibouche, A. A. Voskanyan, E. S. Vasileiadou, M. S. Islam, A. Navrotsky and M. G. Kanatzidis, Entropy Stabilization Effects and Ion Migration in 3D “Hollow” Halide Perovskites, J. Am. Chem. Soc., 2022, 144(18), 8223–8230, DOI:10.1021/jacs.2c01383.
- A. Bonadio, C. A. Escanhoela, F. P. Sabino, G. Sombrio, V. G. de Paula, F. F. Ferreira, A. Janotti, G. M. Dalpian and J. A. Souza, Entropy-driven stabilization of the cubic phase of MaPbI3 at room temperature, J. Mater. Chem. A, 2021, 9(2), 1089–1099, 10.1039/D0TA10492B.
- S. F. Solari, L.-N. Poon, M. Wörle, F. Krumeich, Y.-T. Li, Y.-C. Chiu and C.-J. Shih, Stabilization of Lead-Reduced Metal Halide Perovskite Nanocrystals by High-Entropy Alloying, J. Am. Chem. Soc., 2022, 144(13), 5864–5870, DOI:10.1021/jacs.1c12294.
- J.-H. Pöhls, M. Heyberger and A. Mar, Comparison of computational and experimental inorganic crystal structures, J. Solid State Chem., 2020, 290, 121557, DOI:10.1016/j.jssc.2020.121557.
- N. Achiwa, Linear Antiferromagnetic Chains in Hexagonal ABCl3-Type Compounds (A; Cs, or Rb, B; Cu, Ni, Co, or Fe), J. Phys. Soc. Jpn., 1969, 27(3), 561–574, DOI:10.1143/JPSJ.27.561.
- M. S. Alam, M. Saiduzzaman, A. Biswas, T. Ahmed, A. Sultana and K. M. Hossain, Tuning band gap and enhancing optical functions of AGeF3 (A = K, Rb) under pressure for improved optoelectronic applications, Sci. Rep., 2022, 12(1), 8663, DOI:10.1038/s41598-022-12713-4.
- P. S. Aleonard, Fluorometallates obtenus par dissolution d’oxydes metalliques dans bain fondu a base de fluoroborate de potassium, C. R. Seances Acad. Sci., Ser. D, 1965, 260, 1977–1980 Search PubMed.
- K. S. Alexandrov, B. V. Besnosikov and L. A. Posdnjakova, Successive phase transitions in perovskites. II. Structures of distorted phases, Ferroelectrics, 1976, 12(1), 197–198, DOI:10.1080/00150197608241424.
- E. Alter and R. Hoppe, Uber Fluoropalladate(II): KPdF3, RbPdF3, TIPdF3 und K2PdF4, Z. Anorg. Allg. Chem., 1974, 408(2), 115–120, DOI:10.1002/zaac.19744080205.
-
M. Arakawa, H. Ebisu and H. Takeuchi, EPR study of Cr3+ centres in Tl2MgF4 and Tl2ZnF4 crystals, in EPR in the 21st Century, ed. A. Kawamori, J. Yamauchi and H. Ohta, Elsevier Science B.V., Amsterdam, 2002, pp. 219–224 Search PubMed.
- H. Arif, M. B. Tahir, M. Sagir, S. Znaidia, H. Alrobei and M. Alzaid, First-principles calculations to investigate “H” and “K” doped RbSrF3 for photovoltaic applications, Optik, 2022, 271, 169864, DOI:10.1016/j.ijleo.2022.169864.
- E. C. Ashby, R. S. Smith and A. B. Goel, Comparative studies on the addition reactions of the Normant reagent (“CH3MgBr” + CuBr) and the new tetrahydrofuran-soluble magnesium methylcuprates MgmCun(CH3)2m+n with phenylacetylene, J. Org. Chem., 1981, 46(25), 5133–5139, DOI:10.1021/jo00338a013.
- R. W. Asmussen, T. K. Larsen and H. Soling, The crystal structure of RbNiCl3 and RbNiBr3. The Weiss constant in relation to the crystal structure of some double halides of the type ANiX3, Acta Chem. Scand., 1969, 23, 2055–2060, DOI:10.3891/acta.chem.scand.23-2055.
-
D. Babel, in Structural chemistry of octahedral fluorocomplexes of the transition elements, Structure and Bonding, Berlin, Heidelberg, ed. C. K. Jørgensen, J. B. Neilands, R. S. Nyholm, D. Reinen and R. J. P. Williams, Springer Berlin Heidelberg, Berlin, Heidelberg, 1967, pp. 1–87 Search PubMed.
- B. Bachmann and B. G. Müller, Einkristalluntersuchungen an Fluoroperowskiten MPdF3 (M = Rb, K) und PdF2, Z. Anorg. Allg. Chem., 1993, 619(2), 387–391, DOI:10.1002/zaac.19936190225.
- C. Baopeng, W. Shihua and Z. Xinhua, Synthesis and structure of AEuI3 (A = Rb, Cs) and AEu2I5 (A = K, Rb, Cs), J. Alloys Compd., 1992, 181(1), 511–514, DOI:10.1016/0925-8388(92)90348-D.
- H. P. Beck, H. Tratzky, V. Kallmayer and K. Stöwe, The InSnCl3-Type Arrangement: I. A New ABX3 Structure Type with Close Cation–Cation Contacts, J. Solid State Chem., 1999, 146(2), 344–350, DOI:10.1006/jssc.1999.8360.
- M. Belabbas, N. Marbouh, O. Arbouche and A. Hussain, Optoelectronic properties of the novel perovskite materials LiPb(Cl:Br:I)3 for enhanced hydrogen production by visible photo-catalytic activity: Theoretical prediction based
on empirical formulae and DFT, Int. J. Hydrogen Energy, 2020, 45(58), 33466–33477, DOI:10.1016/j.ijhydene.2020.09.066.
- F. L. M. Bernal, J. Sottmann, D. S. Wragg, H. Fjellvåg, Ø. S. Fjellvåg, C. Drathen, W. A. Sławiński and O. M. Løvvik, Structural and magnetic characterization of the elusive Jahn-Teller active NaCrF3, Phys. Rev. Mater., 2020, 4(5), 054412, DOI:10.1103/PhysRevMaterials.4.054412.
- J. Bill, K. Lerch and W. Laqua, Cs2AgIAgIIICl6. Eine gemischtvalente Verbindung mit dreiwertigem Silber, Z. Anorg. Allg. Chem., 1990, 589(1), 7–11, DOI:10.1002/zaac.19905890102.
- S. R. A. Bird, J. D. Donaldson and J. Silver, The Mössbauer effect in tin(II) compounds. Part XII. The spectra of the chloro- and bromo-stannates(II), J. Chem. Soc., Dalton Trans., 1972,(18), 1950–1953, 10.1039/DT9720001950.
- A. V. Bogdanova, N. P. Zaslavskaya, E. V. Sinichka, M. F. Fedyna, I. R. Mokra and S. M. Gasinets, Synthesis and Crystal Structure of Compounds TlCdCl3 and TlCdBr3, Inorg. Mater., 1993, 29, 664–666, DOI:10.1002/chin.199346021.
- A. I. Boltalin and Y. M. Korenev, Reaction between KF and PbF2 in Solid and Gas Phases, J. Inorg. Chem., 1996, 41(6), 924–927 Search PubMed.
- E. D. Bourret-Courchesne, G. A. Bizarri, R. Borade, G. Gundiah, E. C. Samulon, Z. Yan and S. E. Derenzo, Crystal growth and characterization of alkali-earth halide scintillators, J. Cryst. Growth, 2012, 352(1), 78–83, DOI:10.1016/j.jcrysgro.2012.01.014.
- J. Brynestad, H. L. Yakel and G. P. Smith, Temperature Dependence of the Absorption Spectrum of Nickel(II)-Doped KMgCl3 and the Crystal Structure of KMgCl3, J. Chem. Phys., 2004, 45(12), 4652–4664, DOI:10.1063/1.1727550.
- G. A. Bukhalova, V. T. Berezhnaya and N. E. Okol’chishena, Some properties of compounds of the type M1+M2+F3, Russ. J. Inorg. Chem., 1969, 14, 917–921 Search PubMed.
- A. Bulou, J. Nouet, A. W. Hewat and F. J. Schäfer, Structural phase transitions in KCaF3 – DSC, birefringence and neutron powder diffraction results, Ferroelectrics, 1980, 25(1), 375–378, DOI:10.1080/00150198008207024.
- F. L. Carter, On the existence of two forms of NaNiF3, Solid State Commun., 1969, 7(14), 993–995, DOI:10.1016/0038-1098(69)90070-2.
- L. Ch’ih-fa and L. S. Morozov, Thermal and tensimetric investigation of systems formed by tin(II) chloride with alkali metal and ammonium chloride, J. Therm. Anal. Calorim., 1963, 8(3), 708–711 Search PubMed.
- J. C. Cousseins and A. De Kozak, Sur les fluorures doubles de chrome bivalent de potassium ou de rubidium, C. R. Seances Acad. Sci., Ser. C, 1966, 263(25), 1533–1535 CAS.
- D. E. Cox and F. C. Merkert, The preparation, crystal growth and perfection of double halides of CsNiCl3 type, J. Cryst. Growth, 1972, 13–14, 282–284, DOI:10.1016/0022-0248(72)90170-4.
-
W. J. Crama, On the cooperative Jahn-Teller effect in ternary chromium(II) and copper(II) halides, PhD Thesis, Rijksuniversiteit Leiden, Netherlands, 1980.
- C. Cros, L. Hanebali, L. Latié, G. R. Villeneuve and W. Gang, Structure, ionic motion and conductivity in some solid-solutions of the LiClMCl2 systems (M = Mg, V, Mn), Solid State Ionics, 1983, 9–10, 139–147, DOI:10.1016/0167-2738(83)90223-0.
- J.-M. Dance, N. Kerkouri, J.-L. Soubeyroux, J. Darriet and A. Tressaud, Cationic substitutions in fluorides of hexagonal perovskite type. III. The CsNi1−xCdxF3 system: Crystal chemistry and trimeric magnetic interactions in CsNi34Cd14F3, Mater. Lett., 1982, 1(2), 49–52, DOI:10.1016/0167-577X(82)90004-0.
- P. Daniel, J. Toulouse, J. Y. Gesland and M. Rousseau, Raman-scattering
investigation of the hexagonal perovskite RbZnF3, Phys. Rev. B: Condens. Matter Mater. Phys., 1995, 52(13), 9129–9132, DOI:10.1103/PhysRevB.52.9129.
- P. Demchenko, O. Y. Khyzhun, P. M. Fochuk, S. I. Levkovets, G. L. Myronchuk and O. V. Parasyuk, Single crystal growth, structure and properties of TlHgBr3, Opt. Mater., 2015, 49, 94–99, DOI:10.1016/j.optmat.2015.08.026.
-
C. Deschene, In Situ Monitoring of Cation and Anion Exchange in Perovskite Nanoparticles for Applications in Catalysis, Sensing, and Batteries, BS Honors thesis, Syracuse University, 2019 Search PubMed.
- K. O. Devaney, M. R. Freedman, G. L. McPherson and J. L. Atwood, Electron paramagnetic resonance studies of manganese(II) and nickel(II) in three structural phases of rubidium magnesium chloride and the crystal structure of 6H-rubidium magnesium chloride, Inorg. Chem., 1981, 20(1), 140–145, DOI:10.1021/ic50215a030.
- R. C. DeVries and R. Roy, Fluoride Models for Oxide Systems of Dielectric Interest. The Systems KF—MgF2 and AgF-ZnF2, J. Am. Chem. Soc., 1953, 75(10), 2479–2484, DOI:10.1021/ja01106a059.
- R. Dronskowski, InFeBr3 and InMnBr3: Synthesis, Crystal Structure, Magnetic Properties, and Electronic Structure, Inorg. Chem., 1994, 33(25), 5927–5933, DOI:10.1021/ic00103a047.
- R. Dronskowski, Synthesis, Crystal Structure, and Electronic Structure of InCdBr3, J. Solid State Chem., 1995, 116(1), 45–52, DOI:10.1006/jssc.1995.1180.
- P. G. Dubovoj, Formation of compounds in the RbCl-BeCl2 system, Ukr. Khem. Zh., 1979, 45(12), 1234–1235 Search PubMed.
- D. E. Eastman and M. W. Shafer, Antiferromagnetic Resonance in Cubic TlMnF3, J. Appl. Phys., 2004, 38(3), 1274–1276, DOI:10.1063/1.1709576.
- H. Ebisu, M. Arakawa and H. Takeuchi, An EPR study of trigonally symmetric Cr3+ centres in TlZnF3 single crystals, J. Phys.: Condens. Matter, 2005, 17(29), 4653, DOI:10.1088/0953-8984/17/29/008.
- H. Ehrenberg, H. Fuess, S. Hesse, J. Zimmermann, H. von Seggern and M. Knapp, Structures of CsEuBr3 and its degradation product Cs2EuBr5·10H2O, Acta Crystallogr., Sect. B: Struct. Sci., 2007, 63(2), 201–204, DOI:10.1107/S0108768106049032.
- Å. Engberg and H. Soling, On the crystal structures of RbCoCl3 and Rb3CoCl5, Acta Chem. Scand., 1967, 21, 168–174, DOI:10.3891/acta.chem.scand.21-0168.
- G. E. Eperon, S. D. Stranks, C. Menelaou, M. B. Johnston, L. M. Herz and H. J. Snaith, Formamidinium lead trihalide: a broadly tunable perovskite for efficient planar heterojunction solar cells, Energy Environ. Sci., 2014, 7(3), 982–988, 10.1039/C3EE43822H.
-
B. Ewald, C. Kudla, P. Heines, H.-L. Keller and C. Lathe, Investigation of the group subgroup transition in AgPbBr3, 2002, vol. 2.
- N. Fedoseeva, I. Spevakova, G. Petrakovskii, V. Chuev and S. Petrov, Magnetic structure and magnetic field behaviour of NaMnCl3, J. Magn. Magn. Mater., 1980, 15–18, 539–541, DOI:10.1016/0304-8853(80)91166-X.
- N. V. Fedoseeva, T. A. Velikanova and A. G. Zvegintsev, High-pressure cubic phase of RbMnCl3 - magnetic properties, Phys. Status Solidi A, 1979, 51(1), K93–K96, DOI:10.1002/pssa.2210510157.
- H. Fink and H.-J. Seifert, Über die Systeme des Europium(II)- und Strontiumchlorids mit Alkalimetallchloriden und Thalliumchlorid [1], Z. Anorg. Allg. Chem., 1980, 466(1), 87–96, DOI:10.1002/zaac.19804660111.
- I. Földvári, R. Voszka and Z. Morlin, The Properties of Ni Ions in NaCl Single Crystals. I. Vacuum Ultraviolet, Ionic Conductivity, and X-Ray Diffraction Studies, Phys. Status Solidi B, 1978, 89(1), 235–240, DOI:10.1002/pssb.2220890130.
- J. Foulon, J. Durand, A. Larbot, L. Cot and A. Soufiane, Crystal structures of MSnF3 for M = K, Rb, Tl; ionic mobility, Eur. J. Solid State Inorg. Chem., 1993, 30, 87–99, DOI:10.1002/chin.199321003.
- N. Gallo, V. D. Bianco and S. Doronzo, Mercury thiocyanate and cadmium iodide complexes with KCNS and KI in methylmethacrylate, J. Inorg. Nucl. Chem., 1972, 34(7), 2374–2375, DOI:10.1016/0022-1902(72)80178-7.
- J. I. Gómez-Peralta and X. Bokhimi, Ternary halide perovskites for possible optoelectronic applications revealed by Artificial Intelligence and DFT calculations, Mater. Chem. Phys., 2021, 267, 124710, DOI:10.1016/j.matchemphys.2021.124710.
- E. C. Gonzalo, M. L. Sanjuán, M. Hoelzel, M. T. Azcondo, U. Amador, I. Sobrados, J. Sanz, F. García-Alvarado and A. Kuhn, Synthesis and Characterization of NaNiF3·3H2O: An Unusual Ordered Variant of the ReO3 Type, Inorg. Chem., 2015, 54(7), 3172–3182, DOI:10.1021/ic5026262.
- J. Goodyear, E. M. Ali and H. H. Sutherland, Rubidium tribromomanganate, Acta Crystallogr., Sect. B: Struct. Crystallogr. Cryst. Chem., 1980, 36(3), 671–672, DOI:10.1107/S0567740880004074.
- J. Goodyear and D. J. Kennedy, The crystal structure of CsMnCl3, Acta Crystallogr., Sect. B: Struct. Crystallogr. Cryst. Chem., 1973, 29(4), 744–748, DOI:10.1107/S0567740873003286.
- E. Gordo, G. Z. Chen and D. J. Fray, Toward optimisation of electrolytic reduction of solid chromium oxide to chromium powder in molten chloride salts, Electrochim. Acta, 2004, 49(13), 2195–2208, DOI:10.1016/j.electacta.2003.12.045.
-
R. G. Grebenshchikov, A study of the RbF–BeF2 equilibrium diagram and its relation to BaO–SiO2 system, Proceedings of the Academy of Sciences, Institute of Silicate Chemistry of the USSR Academy of Sciences, 1957, vol. 114(2), pp. 316–319.
- E. Gurewitz, A. Horowitz and H. Shaked, Magnetic spiral structure of KMnCl3—a neutron-diffraction study, Phys. Rev. B: Condens. Matter Mater. Phys., 1979, 20(11), 4544–4549, DOI:10.1103/PhysRevB.20.4544.
- E. Gurewitz, J. Makovsky and H. Shaked, Neutron-diffraction study of the magnetic structure of KFeCl3, Phys. Rev. B: Solid State, 1974, 9(3), 1071–1076, DOI:10.1103/PhysRevB.9.1071.
- E. Gurewitz and H. Shaked, Neutron diffraction study of the crystallographic and magnetic structures of potassium tribromoferrate(II), Acta Crystallogr., Sect. B: Struct. Crystallogr. Cryst. Chem., 1982, 38(11), 2771–2775, DOI:10.1107/S0567740882009923.
- I. Hamideddine, N. Tahiri, O. E. Bounagui and H. Ez-Zahraouy,
Ab initio study of structural and optical properties of the halide perovskite KBX3 compound, J. Korean Ceram. Soc., 2022, 59(3), 350–358, DOI:10.1007/s43207-021-00178-6.
- M. Harada, Jahn-Teller Phase Transitions in RbCuCl3, J. Phys. Soc. Jpn., 1983, 52(5), 1646–1657, DOI:10.1143/JPSJ.52.1646.
- T. Haseda, N. Wada, M. Hata and K. Amaya, Spin ordering in a triangular X–Y antiferromagnet: CsFeCl3 and RbFeCl3, Physica B+C, 1981, 108(1), 841–842, DOI:10.1016/0378-4363(81)90725-7.
- H. J. Haupt, F. Huber and H. Preut, Darstellung und Kristallstruktur von Rubidiumtrijodoplumbat(II), Z. Anorg. Allg. Chem., 1974, 408(2), 209–213, DOI:10.1002/zaac.19744080215.
- A. Hauser, U. Falk, P. Fischer, A. Furrer and H. U. Güdel, Neutron scattering investigation of 1D and 3D magnetic ordering and excitations in AVX3 (A = Rb, Cs; X = Cl, Br, I), J. Magn. Magn. Mater., 1983, 31–34, 1139–1140, DOI:10.1016/0304-8853(83)90832-6.
- A. Hauser, U. Falk, P. Fischer and H. U. Güdel, Magnetic order in AVX3 (A = Rb, Cs, (CD3)4 N; X = Cl, Br, I): A neutron diffraction study, J. Solid State Chem., 1985, 56(3), 343–354, DOI:10.1016/0022-4596(85)90184-7.
- Hayatullah, G. Murtaza, R. Khenata, S. Mohammad, S. Naeem, M. N. Khalid and A. Manzar, Structural, elastic, electronic and optical properties of CsMCl3 (M = Zn, Cd), Phys. B, 2013, 420, 15–23, DOI:10.1016/j.physb.2013.03.011.
- C. Hebecker, Neue ternäre Fluoride mit einwertigem Thallium und Silber als Kationen, Naturwissenschaften, 1973, 60(3), 154, DOI:10.1007/BF00594787.
- M. Hidaka and S. Hosogi, The crystal structure of KCdF3, J. Phys. France, 1982, 43(8), 1227–1232, DOI:10.1051/jphys:019820043080122700.
- K. Hirakawa, H. Yoshizawa and K. Ubukoshi, Magnetic and Neutron Scattering Study of One-Dimensional Heisenberg Antiferromagnet CsVCl3, J. Phys. Soc. Jpn., 1982, 51(4), 1119–1122, DOI:10.1143/JPSJ.51.1119.
- J. B. Hoffman, A. L. Schleper and P. V. Kamat, Transformation of Sintered CsPbBr3 Nanocrystals to Cubic CsPbI3 and Gradient CsPbBrxI3–x through Halide Exchange, J. Am. Chem. Soc., 2016, 138(27), 8603–8611, DOI:10.1021/jacs.6b04661.
- C. Hohnstedt and G. Meyer, The first ternary iodides with divalent dysprosium, Naturwissenschaften, 1991, 78(10), 462–463, DOI:10.1007/BF01134384.
- C. Hohnstedt and G. Meyer, Metallothermische Reduktion des Tribromids und -iodids von Dysprosium mit Alkalimetallen, Z. Anorg. Allg. Chem., 1993, 619(8), 1374–1378, DOI:10.1002/zaac.19936190809.
- W. Hönle, G. Miller and A. Simon, Preparation, crystal structures, and electronic properties of LiGaCl3 and LiGaI3, J. Solid State Chem., 1988, 75(1), 147–155, DOI:10.1016/0022-4596(88)90312-X.
- W. Hönle and A. Simon, Darstellung und Kristallstrukturen von LiGaBr4 und LiGaBr3, Z. Naturforsch. B, 1986, 41(11), 1391–1398, DOI:10.1515/znb-1986-1113.
- R. Hoppe, W. Dähne and W. Klemm, Mangantetrafluorid mit einem Anhang über LiMnF5 und LiMnF4, Justus Liebigs Ann. Chem., 1962, 658(1), 1–5, DOI:10.1002/jlac.19626580102.
- R. Hoppe and R. Über Homann, CsHgF3, RbHgF3 und KHgF3, Z. Anorg. Allg. Chem., 1969, 369(3–6), 212–216, DOI:10.1002/zaac.19693690312.
- A. Horowitz, M. Amit, J. Makovsky, L. B. Dor and Z. H. Kalman, Structure types and phase transformations in KMnCl3 and TlMnCl3, J. Solid State Chem., 1982, 43(2), 107–125, DOI:10.1016/0022-4596(82)90220-1.
- R. A. Howie, W. Moser, R. G. Starks, F. W. D. Woodhams and W. Parker, Potassium tin(II) sulphate and related tin apatites: Mössbauer and X-ray studies, J. Chem. Soc., Dalton Trans., 1973, 14, 1478–1484, 10.1039/DT9730001478.
- J. Huang, T. Lei, M. Siron, Y. Zhang, S. Yu, F. Seeler, A. Dehestani, L. N. Quan, K. Schierle-Arndt and P. Yang, Lead-free Cesium Europium Halide Perovskite Nanocrystals, Nano Lett., 2020, 20(5), 3734–3739, DOI:10.1021/acs.nanolett.0c00692.
- S. Huang, H. Shan, W. Xuan, W. Xu, D. Hu, L. Zhu, C. Huang, W. Sui, C. Xiao, Y. Zhao, Y. Qiang, X. Gu, J. Song and C. Zhou, High-Performance Humidity Sensor Based on CsPdBr3 Nanocrystals for Noncontact Sensing of Hydromechanical Characteristics of Unsaturated Soil, Phys. Status Solidi RRL, 2022, 16(6), 2200017, DOI:10.1002/pssr.202200017.
- J. Huart, Étude de trois halogénomercurates de thallium, Bull. Mineral., 1965, 88(1), 65–68, DOI:10.3406/bulmi.1965.5806.
- S. Idrissi, O. Mounkachi, L. Bahmad and A. Benyoussef, Study of the electronic and opto-electronic properties of the perovskite KPbBr3 by DFT and TDDFT methods, Comput. Condens. Matter, 2022, 33, e00617, DOI:10.1016/j.cocom.2021.e00617.
- H. Jex, J. Maetz and M. Müllner, Cubic-to-tetragonal phase transition in RbCaF3 investigated by diffraction experiments with neutrons, x rays, and γ rays from a Mössbauer source, Phys. Rev. B: Condens. Matter Mater. Phys., 1980, 21(3), 1209–1218, DOI:10.1103/PhysRevB.21.1209.
- L. Jongen, T. Gloger, J. Beekhuizen and G. Meyer, Divalent Titanium: The Halides ATiX3 (A = K, Rb, Cs; X = Cl, Br, I), Z. Anorg. Allg. Chem., 2005, 631(2–3), 582–586, DOI:10.1002/zaac.200400464.
- N. Jouini, L. Guen and M. Tournoux, Structure de TlFeBr3: Distorsion du type perovskite hexagonale 2L, Mater. Res. Bull., 1982, 17(11), 1421–1427, DOI:10.1016/0025-5408(82)90228-8.
- N. Jouini, L. Guen and M. Tournoux, Le systeme TlI-GeI2 – Structure cristalline de TlGeI3, Ann. Chim., 1982, 7(1), 45–51 CAS.
- M.-H. Jung, S. H. Rhim and D. Moon, TiO2/RbPbI3 halide perovskite solar cells, Sol. Energy Mater. Sol. Cells, 2017, 172, 44–54, DOI:10.1016/j.solmat.2017.07.011.
- V. Kaiser, M. Otto, F. Binder and D. Babel, Jahn-Teller-Effekt und Kristallstruktur-Verzerrung bei den Kupfer-Fluorperowskiten NaCuF3 und RbCuF3, Z. Anorg. Allg. Chem., 1990, 585(1), 93–104, DOI:10.1002/zaac.19905850112.
-
C. Kaladevi, Studies on some ternary alkali lead bromide crystals, PhD Thesis, Manonmaniam Sundaranar University, 2011 Search PubMed.
- A. Katrusiak and A. Ratuszna, Phase transitions and the structure of NaMnF3 perovskite crystals as a function of temperature and pressure, Solid State Commun., 1992, 84(4), 435–441, DOI:10.1016/0038-1098(92)90492-R.
- K. Khan, J. Sahariya and A. Soni, Structural, electronic and optical modeling of perovskite solar materials ASnX3 (A = Rb, K; X = Cl, Br): First principle investigations, Mater. Chem. Phys., 2021, 262, 124284, DOI:10.1016/j.matchemphys.2021.124284.
- O. Y. Khyzhun, P. M. Fochuk, I. V. Kityk, M. Piasecki, S. I. Levkovets, A. O. Fedorchuk and O. V. Parasyuk, Single crystal growth and electronic structure of TlPbI3, Mater. Chem. Phys., 2016, 172, 165–172, DOI:10.1016/j.matchemphys.2016.01.058.
- H. Kitagawa, H. Sato, N. Kojima, T. Kikegawa and O. Shimomura, Metallization and phase transitions of the three-dimensional halogen-bridge mixed-valence complex Cs2Au2I6 under high pressure, Solid State Commun., 1991, 78(11), 989–995, DOI:10.1016/0038-1098(91)90220-P.
- H. Klasens, P. Zalm and F. Huysman, The manganese emission in ABF3-compounds, Philips Res. Rep., 1953, 8, 441–451 CAS.
- K. Knox, Perovskite-like fluorides. I. Structures of KMnF3, KFeF3, KNiF3 and KZnF3. Crystal field effects in the series and in KCrF3 and KCuF3, Acta Crystallogr., 1961, 14(6), 583–585, DOI:10.1107/S0365110X61001868.
- K. Komarek and P. Herasymenko, Equilibria between Titanium Metal and Solutions of Titanium Dichloride in Fused Sodium Chloride, J. Electrochem. Soc., 1958, 105(4), 216, DOI:10.1149/1.2428803.
- E. N. Kovalenko, O. N. Yunakova and N. N. Yunakov, The exciton absorption spectrum of thin films of ternary compounds in the AgBr–PbBr2 system, Low Temp. Phys., 2018, 44(8), 856–859, DOI:10.1063/1.5049171.
- T. A. Kuku, Structure and ionic conductivity of CuCdCl3, Solid State Ionics, 1987, 25(2), 105–108, DOI:10.1016/0167-2738(87)90109-3.
- M. H. Kuok, L. S. Tan, Z. X. Shen, C. H. Huan and K. F. Mok, A Raman study of RbSnBr3, Solid State Commun., 1996, 97(6), 497–501, DOI:10.1016/0038-1098(95)00625-7.
- M. H. Kuok and S. H. Tang, A Raman Study of KCdBr3 Single Crystals, Phys. Status Solidi B, 1988, 147(2), K195–K199, DOI:10.1002/pssb.2221470261.
- I. Y. Kuznetsova, I. S. Kovaleva and V. A. Fedorov, Cs2CdBr4-CsPbBr3 and CsCdBr3-CsPbBr3 joins of the CdBr2-PbBr2-CsBr ternary system, Zh. Neorg. Khim., 2002, 47(6), 1010–1012 CAS.
- A. Lachgar, D. S. Dudis, P. K. Dorhout and J. D. Corbett, Synthesis and properties of two novel line phases that contain linear scandium chains, lithium scandium iodide (LiScI3) and sodium scandium iodide (Na0.5ScI3), Inorg. Chem., 1991, 30(17), 3321–3326, DOI:10.1021/ic00017a019.
- M. E. Levina and E. V. Yunakovskaya, Phase transitions of the metafluoroberyllates RbBeF3 and CsBeF3, Moscow Univ. Chem. Bull., 1971, 26(6), 40–42 Search PubMed.
- Q.-J. Li, D. Sprouster, G. Zheng, J. C. Neuefeind, A. D. Braatz, J. McFarlane, D. Olds, S. Lam, J. Li and B. Khaykovich, Complex Structure of Molten NaCl–CrCl3 Salt: Cr–Cl Octahedral Network and Intermediate-Range Order, ACS Appl. Energy Mater., 2021, 4(4), 3044–3056, DOI:10.1021/acsaem.0c02678.
- T.-I. Li and G. D. Stucky, Exchange interactions in polynuclear transition metal complexes. Structural properties of cesium tribromocuprate(II), CsCuBr3, a strongly coupled copper(II) system, Inorg. Chem., 1973, 12(2), 441–445, DOI:10.1021/ic50120a040.
- T.-I. Li and G. D. Stucky, The effect of exchange coupling on the spectra of transition metal ions. The crystal structure and optical spectrum of CsCrBr3, Acta Crystallogr., Sect. B: Struct. Crystallogr. Cryst. Chem., 1973, 29(7), 1529–1532, DOI:10.1107/S0567740873004863.
- T.-I. Li, G. D. Stucky and G. L. McPherson, The crystal structure of CsMnCl3 and a summary of the structures of RMX3 compounds, Acta Crystallogr., Sect. B: Struct. Crystallogr. Cryst. Chem., 1973, 29(6), 1330–1335, DOI:10.1107/S0567740873004450.
- Y. Li, Y. Ding, Y. Li, H. Liu, X. Meng, Y. Cong, J. Zhang, X. Li, X. Chen and J. Qin, Synthesis, Crystal Structure and Nonlinear Optical Property of RbHgI3, Crystals, 2017, 7(5), 148, DOI:10.3390/cryst7050148.
- L. Liang, L. Wencong and C. Nianyi, On the criteria of formation and lattice distortion of perovskite-type complex halides, J. Phys. Chem. Solids, 2004, 65(5), 855–860, DOI:10.1016/j.jpcs.2003.08.021.
- A. R. Lim and S.-Y. Jeong,
133Cs nuclear magnetic resonance study in CsZnCl3 single crystals of perovskite ABX3 type, Phys. B, 2008, 403(18), 3217–3220, DOI:10.1016/j.physb.2008.04.007.
- W. Lin, J. He, K. M. McCall, C. C. Stoumpos, Z. Liu, I. Hadar, S. Das, H.-H. Wang, B.-X. Wang, D. Y. Chung, B. W. Wessels and M. G. Kanatzidis, Inorganic Halide Perovskitoid TlPbI3 for Ionizing Radiation Detection, Adv. Funct. Mater., 2021, 31(13), 2006635, DOI:10.1002/adfm.202006635.
-
M. R. Linaburg, Studies of Halide Perovskites CsPbX3, RbPbX3 (X = Cl−, Br−, I−), and Their Solid Solutions, MS thesis, The Ohio State University, 2015 Search PubMed.
- E. v Loef, L. S. Pandian, N. Kaneshige, G. Ciampi, L. Stand, D. Rutstrom, Y. Tratsiak, M. Zhuravleva, C. Melcher and K. S. Shah, Crystal Growth, Density Functional Theory, and Scintillation Properties of TlCaX3 (X = Cl, Br, I), IEEE Trans. Nucl. Sci., 2023, 70(7), 1378–1383, DOI:10.1109/TNS.2023.3258065.
- J. M. Longo, J. A. Kafalas, J. R. O’Connor and J. B. Goodenough, Magnetic and Optical Properties of the High- and Low-Pressure Forms of CsCoF3, J. Appl. Phys., 1970, 41(3), 935–936, DOI:10.1063/1.1659031.
- C. J. J. Loon and D. J. W. Ijdo, The crystal structure of Na6MnCl8 and Na2Mn3Cl8 and some isostructural compounds, Acta Crystallogr., Sect. B: Struct. Crystallogr. Cryst. Chem., 1975, 31(3), 770–773, DOI:10.1107/S0567740875003779.
- M. M. Lukina and G. P. Klientova, Hydrothermal synthesis of KZnF3 single crystals with a perovskite structure, Sov. Phys. – Crystallogr., 1969, 14(2), 314–315 Search PubMed.
- S. Lv, Q. Wu, X. Meng, L. Kang, C. Zhong, Z. Lin, Z. Hu, X. Chen and J. Qin, A promising new nonlinear optical crystal with high laser damage threshold for application in the IR region: synthesis, crystal structure and properties of noncentrosymmetric CsHgBr3, J. Mater. Chem. C, 2014, 2(33), 6796–6801, 10.1039/C4TC00565A.
- M. A. Macdonald, E. N. Mel’chakov, I. H. Munro, P. A. Rodnyi and A. S. Voloshinovsky, Radiative core–valence transitions in CsMgCl3 and CsSrCl3, J. Lumin., 1995, 65(1), 19–23, DOI:10.1016/0022-2313(95)00051-Q.
- K. H. Mahendran, S. Nagaraj, R. Sridharan and T. Gnanasekaran, Differential scanning calorimetric studies on the phase diagram of the binary LiCl–CaCl2 system, J. Alloys Compd., 2001, 325(1), 78–83, DOI:10.1016/S0925-8388(01)01387-1.
- G. Maity and S. K. Pradhan, Composition related structural transition between mechanosynthesized CsPbBr3 and CsPb2Br5 perovskites and their optical properties, J. Alloys Compd., 2020, 816, 152612, DOI:10.1016/j.jallcom.2019.152612.
- K. P. Marshall, S. Tao, M. Walker, D. S. Cook, J. Lloyd-Hughes, S. Varagnolo, A. Wijesekara, D. Walker, R. I. Walton and R. A. Hatton, Cs1−xRbxSnI3 light harvesting semiconductors for perovskite photovoltaics, Mater. Chem. Front., 2018, 2(8), 1515–1522, 10.1039/C8QM00159F.
- C. D. Martin, S. Chaudhuri, C. P. Grey and J. B. Parise, Effect of A-site cation radius on ordering of BX6 octahedra in (K,Na)MgF3 perovskite, Am. Mineral., 2005, 90(10), 1522–1533, DOI:10.2138/am.2005.1693.
- N. Matsushita, H. Ahsbahs, S. S. Hafner and N. Kojima, Crystal Structure of Mixed-Valence Gold Compound, Cs2AuIAuIIICl6 up to 18 GPa, Rev. High Pressure Sci. Technol., 1998, 7, 329–331, DOI:10.4131/jshpreview.7.329.
- N. Matsushita, F. Fukuhara and N. Kojima, A three-dimensional bromo-bridged mixed-valence gold(I,III) compound, Cs2AuIAuIIIBr6, Acta Crystallogr., Sect. E: Struct. Rep. Online, 2005, 61(6), i123–i125, DOI:10.1107/S1600536805016594.
- K. M. McCall, D. Friedrich, D. G. Chica, W. Cai, C. C. Stoumpos, G. C. B. Alexander, S. Deemyad, B. W. Wessels and M. G. Kanatzidis, Perovskites with a Twist: Strong In1+ Off-Centering in the Mixed-Valent CsInX3 (X = Cl, Br), Chem. Mater., 2019, 31(22), 9554–9566, DOI:10.1021/acs.chemmater.9b04095.
- G. L. McPherson, A. M. McPherson and J. L. Atwood, Structures of CsMgBr3, CsCdBr3 and CsMgI3— diamagnetic linear chain lattices, J. Phys. Chem. Solids, 1980, 41(5), 495–499, DOI:10.1016/0022-3697(80)90180-8.
- D. Messer, Die Kristallstruktur von RbGeCl3, Z. Naturforsch., B: J. Chem. Sci., 1978, 33(4), 366–369, DOI:10.1515/znb-1978-0403.
- G. Meyer, Neue Chlor-Perowskite mit zweiwertigen Lanthaniden: CsLnIICl3 (LnII = Sm, Eu, Tm, Yb), Naturwissenschaften, 1978, 65(5), 258, DOI:10.1007/BF00368570.
- G. Meyer, Reduced ternary rare earth halides: State of the art, J. Less-Common Met., 1983, 93(2), 371–380, DOI:10.1016/0022-5088(83)90190-X.
- G. Meyer and J. D. Corbett, Reduced ternary halides of scandium: RbScX3 (X = chlorine, bromine) and CsScX3 (X = chlorine, bromine, iodine), Inorg. Chem., 1981, 20(8), 2627–2631, DOI:10.1021/ic50222a047.
- G. Meyer, D. J. Hinz and U. Flörke, Crystal structure of caesium titanium tribromide, CsTiBr3, Z. Kristallogr. – Cryst. Mater., 1993, 208(1–2), 370–371, DOI:10.1524/zkri.1993.208.12.370.
- G. Meyer and U. Packruhn, Chlorotitanate(II): RbTiCl3 und CsTiCl3, Z. Anorg. Allg. Chem., 1985, 524(5), 90–94, DOI:10.1002/zaac.19855240512.
- V. J. Minkiewicz, D. E. Cox and G. Shirane, The magnetic structures of RbNiCl3 and CsNiCl3, Solid State Commun., 1970, 8(12), 1001–1005, DOI:10.1016/0038-1098(70)90505-3.
-
C. K. Møller, The structure of cæsium plumbo iodide CsPbI3, Munksgaard, 1959, vol. 32, p. 18 Search PubMed.
- H. Monzel, M. Schramm, K. Stöwe and H. P. Beck, Zur Neuuntersuchung des Phasendiagramms RbCl/PbCl2, Z. Anorg. Allg. Chem., 2000, 626(2), 408–411, DOI:10.1002/(SICI)1521-3749(200002)626:2<408::AID-ZAAC408>3.0.CO;2-A.
- E. L. Muetterties, Chemistry of the Difluorides of Germanium and Tin, Inorg. Chem., 1962, 1(2), 342–345, DOI:10.1021/ic50002a029.
- I. C. Muñoz, E. Cruz-Zaragoza, A. Favalli and C. Furetta, Thermoluminescence property of LiMgF3 erbium activated phosphor, Appl. Radiat. Isot., 2012, 70(5), 893–896, DOI:10.1016/j.apradiso.2012.02.006.
- S. Nagaraj, C. V. Vishnuvardhan, S. Ghosh and R. Sridharan, Phase diagram study of CaBr2–LiBr system using DTA, J. Therm. Anal. Calorim., 2014, 115(2), 1835–1839, DOI:10.1007/s10973-013-3507-3.
- N. Narsimlu, D. Srinivasu and G. S. Sastry, Study of optical and transport properties of K2CuCl4·2H2O single crystal, Cryst. Res. Technol., 1994, 29(4), 577–582, DOI:10.1002/crat.2170290423.
- A. Naskar, R. Khanal and S. Choudhury, Role of Chemistry and Crystal Structure on the Electronic Defect States in Cs-Based Halide Perovskites, Materials, 2021, 14(4), 1032, DOI:10.3390/ma14041032.
- M. Natarajan and B. Prakash, Phase transitions in ABX3 type halides, Phys. Status Solidi A, 1971, 4(3), K167–K172, DOI:10.1002/pssa.2210040331.
- M. Natarajan and E. A. Secco, Electrical conductivity and phase transformation studies on the mixed metal halides RbCdX3 (X = Cl, Br, I) and Cs2CuBr4, Phys. Status Solidi A, 1976, 33(1), 427–433, DOI:10.1002/pssa.2210330146.
- M. Niel, C. Cros, G. Le Flem and M. Pouchard, Sur les chlorures doubles de vanadium+II: les phases TlVCl3 et NH4VCl3, C. R. Acad. Sci., 1975, 280(17), 1093–1095 CAS.
-
Y. Nishiwaki and K. Iio, 9aSH-2 Dielectricity and magnetism of hexagonal RbCoBr3 related substance ACoX3 (A = K,Tl B = Cl,Br) (dielectric, region 10), The Physical Society of Japan Lecture Summary Collection, 2002, p. 830 Search PubMed.
- R. H. Odenthal and R. Hoppe, Fluorargentate(II) der Alkalimetalle, Monatsh. Chem., 1971, 102(5), 1340–1350, DOI:10.1007/BF00917190.
- A. Okazaki, Y. Suemune and T. Fuchikami, The Crystal Structures of KMnF3, KFeF3, KCoF3, KNiF3 and KCuF3, J. Phys. Soc. Jpn., 1959, 14(12), 1823–1824, DOI:10.1143/JPSJ.14.1823.
- D. R. Onken, D. Perrodin, S. C. Vogel, E. D. Bourret and F. Moretti, The crystal structure of TlMgCl3 from 290 K to 725 K, Acta Crystallogr., Sect. E: Crystallogr. Commun., 2020, 76(11), 1716–1719, DOI:10.1107/S2056989020013201.
- V. I. Pakhomov and A. V. Goryunov, On the nature of complex formation in some inorganic halides, Russ. J. Inorg. Chem., 1993, 38(9), 1402–1408 Search PubMed.
- M. Paul and H. J. Seifert, EMF-measurements with galvanic bromine cells in the systems RBr/MBr2 (M = Sr, Ba), J. Therm. Anal., 1989, 35(2), 585–593, DOI:10.1007/BF01904460.
- E. Y. Peresh, V. B. Lazarev, V. V. Tsigika, A. V. Orinchaj, I. S. Balog, V. I. Tkachenko and I. I. Pogojda, Homogeneity regions, preparation and analysis of single crystals of certain compounds of Cs(Tl)X-Ge(Sn,Pb,Cd)X2 systems, where X = Cl, Br, I, Izv. Akad. Nauk SSSR, Neorg. Mater., 1985, 21(5), 774–778 CAS.
- M. P. Petrov and G. M. Nedlin, Spin-Density Space Oscillations and Hyperfine Interaction in RbCoF3, J. Appl. Phys., 1968, 39(2), 1012–1014, DOI:10.1063/1.1656148.
-
N. S. Pidzyrajlo, T. V. Triska and Z. A. Khapko, In Recombination luminescence of CsCdI3monocrystals, Theses of the 23 all-union conference on luminescence, USSR, USSR, 1976, p. 89.
- A. I. Popov and Y. M. Kiselev, ChemInform Abstract: Synthesis and Characterization of the Higher Fluorides of Silver and Alkali Metals, Chem. Inf., 1988, 19(27) DOI:10.1002/chin.198827039.
- J. Portier, A. Tressaud and J. Dupin, Les Pérovskites Fluorées AgMeF3 (Me = Mg, Mn; Co; Ni; Cu; Zn), Cr. Acad. Sci. C, 1970, 270, 216–218 CAS.
- S. H. Pulcinelli, J. Senegas, B. Tanguy, F. Menil and J. Portier, Préparation et étude structurale par ratons X et RMN d’un fluorure de composition LiZnF3, Rev. Chim. Miner., 1986, 23(2), 238–249 CAS.
- S. Racine, J. Cipriani and C. Pontikis, Diffusion de la lumiere et excitations magnetiques dans l’antiferromagnetique KCoF3, C. R. Seances Acad. Sci., Ser. B, 1972, 274, 16–18 CAS.
- A. H. Reshak, I. V. Kityk, Z. A. Alahmed, S. Levkovets, A. O. Fedorchuk, G. Myronchuk, K. J. Plucinski, H. Kamarudin and S. Auluck, Experimental and theoretical investigation of the electronic structure and optical properties of TlHgCl3 single crystal, Opt. Mater., 2015, 47, 445–452, DOI:10.1016/j.optmat.2015.06.018.
- M. Retuerto, T. Emge, J. Hadermann, P. W. Stephens, M. R. Li, Z. P. Yin, M. Croft, A. Ignatov, S. J. Zhang, Z. Yuan, C. Jin, J. W. Simonson, M. C. Aronson, A. Pan, D. N. Basov, G. Kotliar and M. Greenblatt, Synthesis and Properties of Charge-Ordered Thallium Halide Perovskites, CsTl+0.5Tl3+0.5X3 (X = F or Cl): Theoretical Precursors for Superconductivity?, Chem. Mater., 2013, 25(20), 4071–4079, DOI:10.1021/cm402423x.
- R. Riccardi, C. Sinistri, G. Y. Campari and A. Magistris, Binary Systems Formed by Alkali Bromides with Barium or Strontium Bromide, Z. Naturforsch., A: Astrophys., Phys. Phys. Chem., 1970, 25(5), 781–785, DOI:10.1515/zna-1970-0536.
- M. Rousseau, J. Y. Gesland, J. Julliard, J. Nouet, J. Zarembowitch and A. Zarembowitch, Changement de phase structural dans RbCdF3 et TlCdF3, J. Physique Lett., 1975, 36(5), 121–124, DOI:10.1051/jphyslet:01975003605012100.
- W. Rüdorff, G. Lincke and D. Babel, Untersuchungen an ternären Fluoriden. (II). Kobalt(II)- und Kupfer(II)-fluoride, Z. Anorg. Allg. Chem., 1963, 320(1–4), 150–170, DOI:10.1002/zaac.19633200119.
- C. Rüegg, N. Cavadini, A. Furrer, H. U. Güdel, K. Krämer, H. Mutka, A. Wildes, K. Habicht and P. Vorderwisch, Bose–Einstein condensation of the triplet states in the magnetic insulator TlCuCl3, Nature, 2003, 423(6935), 62–65, DOI:10.1038/nature01617.
- Sandeep, D. P. Rai, A. Shankar, M. P. Ghimire, R. Khenata, S. Bin Omran, S. V. Syrotyuk and R. K. Thapa, Investigation of the structural, electronic and optical properties of the cubic RbMF3 perovskites (M = Be, Mg, Ca, Sr and Ba) using modified Becke-Johnson exchange potential, Mater. Chem. Phys., 2017, 192, 282–290, DOI:10.1016/j.matchemphys.2017.02.005.
- K. Sawada and M. Tanaka, Formation of bromo complexes of cobalt(II) in acetic acid, J. Inorg. Nucl. Chem., 1977, 39(2), 339–344, DOI:10.1016/0022-1902(77)80026-2.
- V. Scatturin, L. Corliss, N. Elliott and J. Hastings, Magnetic structures of 3d transition metal double fluorides, KMeF3, Acta Crystallogr., 1961, 14(1), 19–26, DOI:10.1107/S0365110X61000036.
- G. Schilling, C. Kunert, T. Schleid and G. Meyer, Metallothermische Reduktion der Tribromide und -iodide von Thulium und Ytterbium mit Alkalimetallen, Z. Anorg. Allg. Chem., 1992, 618(12), 7–12, DOI:10.1002/zaac.19926180102.
- G. Schilling and G. Meyer, Ternäre Bromide und Iodide zweiwertiger Lanthanide und ihre Erdalkali-Analoga vom Typ AMX3 und AM2X5, Z. Anorg. Allg. Chem., 1996, 622(5), 759–765, DOI:10.1002/zaac.19966220502.
- O. Schmitz-Dumont, G. Bergerhoff and E. Hartert, Über den Einfluß des Kationenradius auf die Bildungsenergie von Anlagerungsverbindungen. VII. Die Systeme Alkalifluorid/Bleifluorid, Z. Anorg. Allg. Chem., 1956, 283(1–6), 314–329, DOI:10.1002/zaac.19562830131.
- M. Schölten and R. Dronskowski, Crystal structure of indium magnesium tribromide, InMgBr3, Z. Kristallogr. – New Cryst. Struct., 1997, 212(1), 5, DOI:10.1524/ncrs.1997.212.1.5.
- M. Scholten, R. Dronskowski and H. Jacobs, InCrBr3:
A Ternary Indium Bromide Containing Jahn−Teller Unstable Cr2+ and the Magnetic Structures of InCrBr3 and InFeBr3, Inorg. Chem., 1999, 38(11), 2614–2620, DOI:10.1021/ic981383t.
- M. Scholten, R. Dronskowski, T. Staffel and G. Meyer, Synthesis and Crystal Structure of Potassium Indium Tribromide, KInBr3, Z. Anorg. Allg. Chem., 1998, 624(11), 1741–1745, DOI:10.1002/(SICI)1521-3749(1998110)624:11<1741::AID-ZAAC1741>3.0.CO;2-W.
-
B. Schüpp, Präparation und Charakterisierung neuer Halogenopalladate mit besonderem Schwergewicht be
glich mehrkerniger Halogenopalladatgruppen, PhD Thesis, Universität Dortmund, 1999.
- B. Schüpp and H.-L. Keller, CsPdCl3 – eine Verbindung mit [Pd2Cl6]-Baugruppen und anorganischem Kation, Z. Anorg. Allg. Chem., 1999, 625(11), 1944–1950, DOI:10.1002/(SICI)1521-3749(199911)625:11<1944::AID-ZAAC1944>3.0.CO;2-V.
- M. Sebastian, J. A. Peters, C. C. Stoumpos, J. Im, S. S. Kostina, Z. Liu, M. G. Kanatzidis, A. J. Freeman and B. W. Wessels, Excitonic emissions and above-band-gap luminescence in the single-crystal perovskite semiconductors CsPbBr3 and CsPbCl3, Phys. Rev. B: Condens. Matter Mater. Phys., 2015, 92(23), 235210, DOI:10.1103/PhysRevB.92.235210.
- H.-J. Seifert and E. Dau, Über die Systeme Alkalimetallbromid/Mangan(II)-bromid, Z. Anorg. Allg. Chem., 1972, 391(3), 302–312, DOI:10.1002/zaac.19723910311.
- H.-J. Seifert and P. Ehrlich, Über die Systeme NaCl/VCl2, KCl/VCl2 und CsCl/VCl2, Z. Anorg. Allg. Chem., 1960, 302(5–6), 284–288, DOI:10.1002/zaac.19603020506.
- H.-J. Seifert, H. Fink, G. Thiel and J. Uebach, Thermodynamische und strukturelle Untersuchungen an den Verbindungen der Systeme KCl/MCl2 (M = Ca, Cd, Co, Ni), Z. Anorg. Allg. Chem., 1985, 520(1), 151–159, DOI:10.1002/zaac.19855200118.
- H.-J. Seifert and D. Haberhauer, Über die Systeme Alkalimetallbromid/Calciumbromid, Z. Anorg. Allg. Chem., 1982, 491(1), 301–307, DOI:10.1002/zaac.19824910139.
- H.-J. Seifert and K. Klatyk, Über die Systeme Alkalimetallchlorid/Chrom(II)-chlorid, Z. Anorg. Allg. Chem., 1964, 334(3–4), 113–124, DOI:10.1002/zaac.19643340302.
- H.-J. Seifert and K. Klatyk, Über die Systeme RbCl/FeCl2 und CsCl/FeCl2, Z. Anorg. Allg. Chem., 1966, 342(1–2), 1–9, DOI:10.1002/zaac.19663420102.
- H. J. Seifert and K. H. Kischka, Investigations on systems AX/MnX2 (A = Li–Cs, TI; X = Cl, Br, I) by DTA and X-ray analysis, Thermochim. Acta, 1978, 27(1), 85–93, DOI:10.1016/0040-6031(78)85023-0.
- H. J. Seifert and K. Klatyk, Das System CsCl/CrCl2, Naturwissenschaften, 1962, 49(23), 539, DOI:10.1007/BF00626806.
- H. J. Seifert, T. Krimmel and W. Heinemann, Über die Systeme TlX/MnX2 und AgX/MnX2 (X = Cl, Br, I), J. Therm. Anal., 1974, 6(1), 175–182, DOI:10.1007/BF01911498.
- H. J. Seifert and U. Langenbach, Thermoanalytische und röntgenographische Untersuchungen an Systemen Alkalichlorid/Calciumchlorid, Z. Anorg. Allg. Chem., 1969, 368(1–2), 36–43, DOI:10.1002/zaac.19693680107.
- M. W. Shafer, The synthesis and characterization of vanadium difluoride, NaVF3, KVF3, and RbVF3, Mater. Res. Bull., 1969, 4(12), 905–912, DOI:10.1016/0025-5408(69)90047-6.
- K. Sintani, Y. Tomono, A. Tsuchida and K. Siratori, Influence of Magnetic Ordering on the Lattice Vibration of KNiF3, J. Phys. Soc. Jpn., 1968, 25(1), 99–108, DOI:10.1143/JPSJ.25.99.
- J. L. Sommerdijk and A. Bril, Divalent europium luminescence in perovskite-like alkaline-earth alkaline fluorides, J. Lumin., 1976, 11(5), 363–367, DOI:10.1016/0022-2313(76)90021-1.
- J. L. Soubeyroux, C. Cros, W. Gang, R. Kanno and M. Pouchard, Neutron diffraction investigation of the cationic distribution in the structure of the spinel-type solid solutions Li2−2xM1+xCl4 (M = Mg, V): Correlation with the ionic conductivity and NMR data, Solid State Ionics, 1985, 15(4), 293–300, DOI:10.1016/0167-2738(85)90132-8.
- J. Spector, G. Villeneuve, L. Hanebali and C. Cros, NMR Investigations of the Li+ ion mobility in the double chlorides Li2MgCl4 and LiMgCl3, Mater. Lett., 1982, 1(2), 43–48, DOI:10.1016/0167-577X(82)90003-9.
- V. I. Spitsyn, S. V. Kryuchkov, M. S. Grigoriev and A. F. Kuzina, Polynuclear Clusters of Technetium. Part 1. Synthesis, Crystal and Molecular Structure of Bromide Octanuclear Prismatic and Hexanuclear Octahedral Clusters of Technetium, Dokl. Akad. Nauk SSSR, 1988, 288, 389–393, DOI:10.1002/chin.198849036.
- M. Steiner, W. Krüger and D. Babel, Proof of ferromagnetic chains in CsNiF3 by neutron diffraction, Solid State Commun., 1971, 9(3), 227–229, DOI:10.1016/0038-1098(71)90123-2.
- H. Steinfink and G. D. Brunton, The crystal structure of CsBeF3, Acta Crystallogr., Sect. B: Struct. Crystallogr. Cryst. Chem., 1968, 24(6), 807–810, DOI:10.1107/S0567740868003225.
- J. Strähle, J. Gelinek and M. Kölmel, Über den thermischen Abbau einiger Alkalimetall- und Ammoniumhalogenoaurate(III) und die Kristallstruktur der Zersetzungsprodukte Rb2Au2Br6, Rb3Au3Cl8 und Au(NH3)Cl3, Z. Anorg. Allg. Chem., 1979, 456(1), 241–260, DOI:10.1002/zaac.19794560125.
- I. Sumio and I. Yoshihiro, The congruent melting compounds in the system KCl–SnCl2, Chem. Lett., 1983,(12), 1803–1806, DOI:10.1246/cl.1983.1803.
- Y. Syono, S.-i Akimoto and K. Kohn, Structure Relations of Hexagonal Perovskite-Like Compounds ABX3 at High Pressure, J. Phys. Soc. Jpn., 1969, 26(4), 993–999, DOI:10.1143/JPSJ.26.993.
- R. G. Szlag, L. Suescun, B. D. Dhanapala and F. A. Rabuffetti, Rubidium–Alkaline-Earth Trifluoroacetate Hybrids as Self-Fluorinating Single-Source Precursors to Mixed-Metal Fluorides, Inorg. Chem., 2019, 58(5), 3041–3049, DOI:10.1021/acs.inorgchem.8b02988.
- Y. Takeda, M. Shimada, F. Kanamaru and M. Koizumi, Structure and Properties of CsFeBr3, J. Phys. Soc. Jpn., 1974, 37(1), 276, DOI:10.1143/JPSJ.37.276.
- L. C. Tang, J. Y. Huang, C. S. Chang, M. H. Lee and L. Q. Liu, New infrared nonlinear optical crystal CsGeBr3: synthesis, structure and powder second-harmonic generation properties, J. Phys.: Condens. Matter, 2005, 17(46), 7275, DOI:10.1088/0953-8984/17/46/011.
- D. T. Teaney, M. J. Freiser and R. W. H. Stevenson, Discovery of a Simple Cubic Antiferromagnet: Antiferromagnetic Resonance in RbMnF3, Phys. Rev. Lett., 1962, 9(5), 212–214, DOI:10.1103/PhysRevLett.9.212.
- T. Thao Tran and P. Shiv Halasyamani, Synthesis and characterization of ASnF3 (A = Na+, K+, Rb+, Cs+), J. Solid State Chem., 2014, 210(1), 213–218, DOI:10.1016/j.jssc.2013.11.025.
- G. Thiele, H. W. Rotter and K. D. Schmidt, Die Kristallstrukturen und Phasentransformationen von RbGeBr3, Z. Anorg. Allg. Chem., 1988, 559(1), 7–16, DOI:10.1002/zaac.19885590101.
- G. Thiele, H. W. Rotter and K. D. Schmidt, Die Kristallstrukturen und Phasentransformationen des tetramorphen RbGel3, Z. Anorg. Allg. Chem., 1989, 571(1), 60–68, DOI:10.1002/zaac.19895710106.
- G. Thiele and B. R. Serr, Crystal structure of rubidium triiodostannate(II), RbSnI3, Z. Kristallogr. – Cryst. Mater., 1995, 210(1), 64, DOI:10.1524/zkri.1995.210.1.64.
- J. Tong, C. Lee, M. H. Whangbo, R. K. Kremer, A. Simon and J. Köhler, Cooperative Jahn–Teller distortion leading to the spin-1/2 uniform antiferromagnetic chains in triclinic perovskites AgCuF3 and NaCuF3, Solid State Sci., 2010, 12(5), 680–684, DOI:10.1016/j.solidstatesciences.2009.02.028.
- A. Tressaud, R. De Pape, J. Portier and P. Hagenmuller, Les systemes MF-FeF2 (M = Li, Na, Rb, Tl), C. R. Seances Acad. Sci., Ser. C, 1968, 266, 984–986 CAS.
- P. V. Tumram, P. R. Kautkar, S. A. Acharya and S. V. Moharil, NIR Emission and Eu2+Nd3+ Energy Transfer in KSrCl3:Eu2+, Nd3+ phosphor, Mater. Today: Proc., 2017, 4(14), 12582–12585, DOI:10.1016/j.matpr.2017.10.065.
- Y. Vaills, J. Y. Buzaré, A. Gibaud and C. Launay, X-ray investigations of the cubic to tetragonal phase transition in CsCaCl3 at Tc = 95 K, Solid State Commun., 1986, 60(2), 139–141, DOI:10.1016/0038-1098(86)90546-6.
- A. van Roekeghem, J. Carrete, C. Oses, S. Curtarolo and N. Mingo, High-Throughput Computation of Thermal Conductivity of High-Temperature Solid Phases: The Case of Oxide and Fluoride Perovskites, Phys. Rev. X, 2016, 6(4), 041061, DOI:10.1103/PhysRevX.6.041061.
-
P. C. E. Villars, SpringerMaterials Landolt-Börnstein database, Springer Nature, 2023 Search PubMed.
- D. Visser and A. Prodan, Disorder in KNiCl3 as observed by electron diffraction, Phys. Status Solidi A, 1980, 58(2), 481–488, DOI:10.1002/pssa.2210580218.
- A. V. Voloshinovskii, P. A. Rodnyi, A. G. Dmitriev, E. N. Melchakov and S. N. Pidzyrailo, Core-valence luminescence of CsMgCl3 and CsMgF3 crystals, J. Appl. Spectrosc., 1993, 59(1), 560–562, DOI:10.1007/BF00663370.
- F. F. Y. Wang and M. Kestigian, Magnetic Properties of RbFeF3, J. Appl. Phys., 1966, 37(3), 975–976, DOI:10.1063/1.1708546.
- H. Wang Shi and M. Zhoa, Phase diagram of YbI2-RbI binary system and structural investigation of RbYbI3, J. Less-Common Met., 1987, 127, 219–224, DOI:10.1016/0022-5088(87)90381-X.
- J. E. Weidenborner and A. L. Bednowitz, Structures of ferrimagnetic fluorides of ABF3 type. I. RbNiF3, Acta Crystallogr., Sect. B: Struct. Crystallogr. Cryst. Chem., 1970, 26(10), 1464–1468, DOI:10.1107/S0567740870004338.
- A. Weiss and K. Damm, Notizen: Zur Kenntnis des Natrium-trichloromercurats (II) Na(HgCI3). Über Quecksilberhalogenide IV, Z. Naturforsch. B, 1954, 9(1), 82, DOI:10.1515/znb-1954-0116.
- A. F. Wells, 332. The crystal structure of CsCuCl3 and the crystal chemistry of complex halides ABX3, J. Chem. Soc. B, 1947, 1662–1670, 10.1039/JR9470001662.
- R. D. Willett, C. Dwiggins Jr., R. F. Kruh and R. E. Rundle, Crystal Structures of KCuCl3 and NH4CuCl3, J. Chem. Phys., 2004, 38(10), 2429–2436, DOI:10.1063/1.1733520.
- R. F. Williamson and W. O. J. Boo, Lower valence fluorides of vanadium. 1. Synthesis and characterization of sodium trifluorovanadate, potassium trifluorovanadate, and rubidium trifluorovanadate, Inorg. Chem., 1977, 16(3), 646–648, DOI:10.1021/ic50169a030.
-
G. Wittenburg, Untersuchungen von Struktur-Eigenschafts-Beziehungen bei Trihalogenometallaten AMX3 von Germanium(II), Zinn(II) und Blei(II), PhD Thesis, Universität Freiburg, Breisgau, 2000 Search PubMed.
- H. T. Witteveen and J. A. R. Van Veen, Magnetic susceptibilities of polycrystalline samples of the compounds ANiCl3 (A = Rb, NH4, Tl, Cs) and ANiBr3 (A = Rb, Cs), J. Phys. Chem. Solids, 1974, 35(3), 337–346, DOI:10.1016/S0022-3697(74)80027-2.
- H. T. Witteveen and J. A. R. van Veen, Magnetic susceptibilities of the compounds AFeCl3 (A = Tl,Rb,NH4,Cs) with antiferromagnetic linear chains, J. Chem. Phys., 1973, 58(1), 186–191, DOI:10.1063/1.1678903.
- G. Wu and R. Hoppe, Zur Kenntnis der Fluoride zweiwertiger Lanthanoide. II. Über die Synthese von MLnF3 aus MLnF4, Z. Anorg. Allg. Chem., 1984, 514(7), 92–98, DOI:10.1002/zaac.19845140712.
- G.-Q. Wu and R. Hoppe, Neue Fluoro-Perowskite zweiwertiger Lanthaniden. Zur Kenntnis von CsEuF3, CsYbF3 und RbYbF3, Z. Anorg. Allg. Chem., 1983, 504(9), 55–59, DOI:10.1002/zaac.19835040907.
- Y. Xie, S. Wang and X. Zhao, Phase diagram and structure of CsSm(1−x)YbxI3 systems, J. Alloys Compd., 1996, 241(1), 40–43, DOI:10.1016/0925-8388(96)02196-2.
- S. Yakovlev, M. Avdeev and M. Mezouar, High-pressure structural behavior and equation of state of NaZnF3, J. Solid State Chem., 2009, 182(6), 1545–1549, DOI:10.1016/j.jssc.2009.03.031.
- Y. Yamane, K. Yamada and K. Inoue, Mechanochemical synthesis and order–disorder phase transition in fluoride ion conductor RbPbF3, Solid State Ionics, 2008, 179(17), 605–610, DOI:10.1016/j.ssi.2008.04.022.
- T. Yanagida, Y. Fujimoto, M. Arai, M. Koshimizu, T. Kato, D. Nakauchi and N. Kawaguchi, Comparative studies of scintillation properties of Tl-based crystals, Sens. Mater., 2020, 32(4), 1351–1356 CAS.
- H. W. Zandbergen, Neutron powder diffraction and magnetic measurements on TlMnI3 and TlFeI3, J. Solid State Chem., 1981, 37(2), 189–203, DOI:10.1016/0022-4596(81)90085-2.
- H. W. Zandbergen, Neutron powder diffraction and magnetic measurements on RbTil3, RbVI3, and CsVI3, J. Solid State Chem., 1981, 37(3), 308–317, DOI:10.1016/0022-4596(81)90492-8.
- H. W. Zandbergen, G. C. Verschoor and D. J. W. IJdo, The structures of thallium cadmium triiodide and dirubidium iron tetraiodide, Acta Crystallogr., Sect. B: Struct. Crystallogr. Cryst. Chem., 1979, 35(6), 1425–1427, DOI:10.1107/S0567740879006580.
- J. Zhang and J. D. Corbett, Synthesis and structure of The Novel Layered Phase CsTi2Cl7, Z. Anorg. Allg. Chem., 1990, 580(1), 36–44, DOI:10.1002/zaac.19905800105.
- J. Zhang and G. Hong, Luminescence properties of Ce3+ in KMF3 (M = Mg,Ca,Sr,Ba) hosts with perovskite structure, J. Rare Earths, 1997, 2, 75–78 Search PubMed.
- J. Zhang, R. Y. Qi and J. D. Corbett, Two novel titanium halide phases: KTi4Cl11 and CsTi4.3I11, Inorg. Chem., 1991, 30(25), 4794–4798, DOI:10.1021/ic00025a022.
- A. Zodkevitz, J. Makovsky and Z. H. Kalman, The Preparation and Crystal Structure of TlMnCl3, TlFeCl3, TlCoCl3 and TlNiCl3, Isr. J. Chem., 1970, 8(5), 755–762, DOI:10.1002/ijch.197000095.
- G. Bergerhoff and L. Goost, Ammoniumtrifluorostannat(II), Acta Crystallogr., Sect. B: Struct. Crystallogr. Cryst. Chem., 1973, 29(3), 632–633, DOI:10.1107/S0567740873003031.
- P. Charpin, N. Roux and J. Ehnetsmann, Compt. Rend., 1968, 267 C, 484–486 Search PubMed.
- T. Das, G. Di Liberto and G. Pacchioni, Density Functional Theory Estimate of Halide Perovskite Band Gap: When Spin Orbit Coupling Helps, J. Phys. Chem. C, 2022, 126(4), 2184–2198, DOI:10.1021/acs.jpcc.1c09594.
- R. Hoppe, W. Liebe and W. Dähne, Über Fluoromanganate der
Alkalimetalle, Z. Anorg. Allg. Chem., 1961, 307(5–6), 276–289, DOI:10.1002/zaac.19613070507.
- T. D. Huan, V. N. Tuoc and N. V. Minh, Layered structures of organic/inorganic hybrid halide perovskites, Phys. Rev. B, 2016, 93(9), 094105, DOI:10.1103/PhysRevB.93.094105.
- A. Kozak, Rev. Chim. Miner., 1971, 8, 301–335 Search PubMed.
- V. G. Krishnan, S.-q Dou and A. Weiss, Structure and Bonding of Tribromocadmates, ACdBr3, A = NH4, Rb, Cs, CH3NH3, (CH3)2NH2, (CH3)4N], [H2NNH3, and (H2N)3C. An X-ray Diffraction and 79-81Br NQR Study, Z. Naturforsch., A: Phys. Sci., 1991, 46(12), 1063–1082, DOI:10.1515/zna-1991-1212.
- A. Le Bail, J. L. Fourquet, J. Rubín, E. Palacios and J. Bartolomé, NH4CdF3: Structure of the low temperature phase, Phys. B, 1990, 162(3), 231–236, DOI:10.1016/0921-4526(90)90017-O.
- G. Meyer and N. Böhmer, Korrosion von Messing und Bronze durch Ammoniumhalogenide, Z. Anorg. Allg. Chem., 2000, 626(6), 1332–1334, DOI:10.1002/(SICI)1521-3749(200006)626:6<1332::AID-ZAAC1332>3.0.CO;2-X.
- M. J. Portier, A. Tressaud, J. L. Dupin and R. de Pape, Structures et proprietes magnetiques de quelques composes de formule M Fe F3 (M = Na, K, Rb, Cs, NH4, T1), Mater. Res. Bull., 1969, 4(1), 45–50, DOI:10.1016/0025-5408(69)90015-4.
- M. M. Rolies and C. J. De Ranter, A new investigation of ammonium cadmium chloride, Acta Crystallogr., Sect. B: Struct. Crystallogr. Cryst. Chem., 1978, 34(10), 3057–3059, DOI:10.1107/S0567740878010018.
- W. Rüdorbf, J. Kandler and D. Babel, Untersuchungen an ternären Fluoriden. I. Struktur, Magnetismus und Reflexionsspektren von Alkali-, Ammonium- und Thallium-Nickel(II)-fluoriden, Z. Anorg. Allg. Chem., 1962, 317(5–6), 261–287, DOI:10.1002/zaac.19623170502.
- G. Shachar, J. Makovsky and H. Shaked, Neutron diffraction and magnetic measurements of polycrystalline NH4MnCl3, Solid State Commun., 1971, 9(9), 493–495, DOI:10.1016/0038-1098(71)90131-1.
- D. Smith, Hindered Rotation of the Ammonium Ion in the Solid State, Chem. Rev., 1994, 94(6), 1567–1584, DOI:10.1021/cr00030a005.
- C. C. Stoumpos, L. Frazer, D. J. Clark, Y. S. Kim, S. H. Rhim, A. J. Freeman, J. B. Ketterson, J. I. Jang and M. G. Kanatzidis, Hybrid Germanium Iodide Perovskite Semiconductors: Active Lone Pairs, Structural Distortions, Direct and Indirect Energy Gaps, and Strong Nonlinear Optical Properties, J. Am. Chem. Soc., 2015, 137(21), 6804–6819, DOI:10.1021/jacs.5b01025.
- C. C. Stoumpos, L. Mao, C. D. Malliakas and M. G. Kanatzidis, Structure–Band Gap Relationships in Hexagonal Polytypes and Low-Dimensional Structures of Hybrid Tin Iodide Perovskites, Inorg. Chem., 2017, 56(1), 56–73, DOI:10.1021/acs.inorgchem.6b02764.
- J. Tian, D. B. Cordes, C. Quarti, D. Beljonne, A. M. Z. Slawin, E. Zysman-Colman and F. D. Morrison, Stable 6H Organic–Inorganic Hybrid Lead Perovskite and Competitive Formation of 6H and 3C Perovskite Structure with Mixed A Cations, ACS Appl. Energy Mater., 2019, 2(8), 5427–5437, DOI:10.1021/acsaem.9b00419.
- S. I. Troyanov, I. V. Morozov and Y. M. Korenev, The synthesis and crystal structure of ammonium fluorocuprates NH4CuF3 and (NH4)2CuF4, Russ. J. Inorg. Chem., 1993, 38(6), 909–913, DOI:10.1002/chin.199352035.
- K. Yamada, T. Matsui, T. Tsuritani, T. Okuda and S. Ichiba,
127I-NQR, 119Sn Mössbauer Effect, and Electrical Conductivity of MSnI3 (M = K, NH4, Rb, Cs, and CH3NH3), Z. Naturforsch., A: Phys. Sci., 1990, 45(3–4), 307–312, DOI:10.1515/zna-1990-3-416.
- A. Jain, S. P. Ong, G. Hautier, W. Chen, W. D. Richards, S. Dacek, S. Cholia, D. Gunter, D. Skinner, G. Ceder and K. A. Persson, Commentary: The Materials Project: A materials genome approach to accelerating materials innovation, APL Mater., 2013, 1(1), 011002, DOI:10.1063/1.4812323.
- V. I. Hegde, C. K. H. Borg, Z. del Rosario, Y. Kim, M. Hutchinson, E. Antono, J. Ling, P. Saxe, J. E. Saal and B. Meredig, Quantifying uncertainty in high-throughput density functional theory: A comparison of AFLOW, Materials Project, and OQMD, Phys. Rev. Mater., 2023, 7(5), 053805, DOI:10.1103/PhysRevMaterials.7.053805.
- K. V. Sopiha, C. Comparotto, J. A. Márquez and J. J. S. Scragg, Chalcogenide Perovskites: Tantalizing Prospects, Challenging Materials, Adv. Opt. Mater., 2022, 10(3), 2101704, DOI:10.1002/adom.202101704.
- G. Kresse and J. Furthmüller, Efficiency of ab initio total energy calculations for metals and semiconductors using a plane-wave basis set, Comput. Mater. Sci., 1996, 6(1), 15–50, DOI:10.1016/0927-0256(96)00008-0.
- G. Kresse and J. Furthmüller, Efficient iterative schemes for ab initio total-energy calculations using a plane-wave basis set, Phys. Rev. B: Condens. Matter Mater. Phys., 1996, 54(16), 11169–11186, DOI:10.1103/PhysRevB.54.11169.
- J. P. Perdew, K. Burke and M. Ernzerhof, Generalized Gradient Approximation Made Simple, Phys. Rev. Lett., 1996, 77(18), 3865–3868, DOI:10.1103/PhysRevLett.77.3865.
|
This journal is © The Royal Society of Chemistry 2024 |
Click here to see how this site uses Cookies. View our privacy policy here.