DOI:
10.1039/D3NA00487B
(Paper)
Nanoscale Adv., 2024,
6, 826-831
Multigenerational exposure of Ag materials (nano and salt) in soil – environmental hazards in Enchytraeus crypticus (Oligochaeta)†
Received
4th July 2023
, Accepted 16th November 2023
First published on 22nd November 2023
Abstract
Because of its properties, silver is among the most used metals both as salt and as nanomaterials (NMs), hence reaching the environment. Multigenerational (MG) exposure testing is scarce, and especially so for NMs and soil invertebrates. In this study the MG effects of Ag NMs (Ag NM300K) and Ag salt (AgNO3) were assessed, using Enchytraeus crypticus in LUFA 2.2 soil. Survival, reproduction and internal Ag concentration in the animals were measured throughout 7 generations (5 generations (F0–F4) in spiked soil plus 2 (F5–F6) in clean soil) exposed to sublethal concentrations corresponding to the reproduction EC10 and EC50 obtained in standard toxicity tests (45 and 60 mg Ag per kg soil DW for AgNO3; 20 and 60 mg Ag per kg soil DW for Ag NM300K). MG exposure caused a dose-related decrease in reproduction for both Ag forms. Ag uptake peaked in the F1 (64 days) for AgNO3 and F2 (96 days) for Ag NM300K, after which it decreased. In agreement with toxicokinetic studies, a maximum body Ag concentration was reached (20 mg Ag per kg body DW (AgNO3) and 70 mg Ag per kg body DW (Ag NM300K)) and after which detoxification mechanisms seem to be activated with elimination of Ag accompanied by a decrease in reproduction. Transfer to clean soil allowed Ag to be (fully) eliminated from the animals. This MG study confirmed the effects determined in standard reproduction toxicity tests but further allowed to monitor the dynamics between exposure and effects of the Ag materials, and how the animals seem to cope with Ag for 7 generations by compensating between detoxification and reproductive output.
Introduction
Silver (Ag) is one of the most used metals in the nanomaterial (NM) form, with an estimated total production in the European Union (EU) of about 50 tons in 2014.1,2 Due to its antimicrobial activity properties, Ag is applied in a wide range of products, both in the salt and nano form, e.g. hospital supplies, clothing, daily care products, food packaging, water disinfectants, refrigerators.3–7 Silver is retained in wastewater treatment plants and the application of sewage sludge to soil is one of the largest sources of entry in the terrestrial environment, which acts as a sink for this element.1,8 The increasing use of Ag in various applications leads to its increasing entry into the soil environment.3,8,9 Soil organisms can be exposed to increasing levels of Ag, hence concerns on its potential adverse effects increase.10–13 Silver NM toxicity has been shown in soil invertebrates, even at low concentrations, e.g., in Enchytraeus crypticus,14Lumbricus rubellus,15Eisenia fetida,16Eisenia andrei, Folsomia candida,17 and Porcellionides pruinosus.18 The exposure period in standard toxicity tests commonly ranges from 21 to 28 days (enchytraeids, collembolans) to 56 days (earthworms) to assess chronic effects like on the reproduction of these soil invertebrates. Experience with NMs has often shown that effects occur at longer exposure times.19–21 For instance, results from a full life cycle test with E. crypticus,14 with exposure to silver (AgNO3 and Ag NM300K) over a longer period of time, allowed to identify effects of the two Ag forms which could not be shown via the standard test alone. It has been long recognized that longer-term testing is needed, e.g., exposure to a low concentration of a contaminant for a longer time can be even more hazardous than a short-term exposure to higher concentrations.22 Multigenerational (MG)23 exposure occurs when chemicals are present at lower concentrations in the environment, often when highly persistent. Moreover, it has been shown that for NMs special attention should be given to lower concentrations, where highest hazards can be observed, as shown e.g. for AgNMs in E. crypticus14 which showed a non-monotonic dose–response relationship. Cumulative damage over generations (like due to oxidative stress that can cause DNA damage) may only become evident after several generations.23 An MG approach with longer exposure durations and more sampling points carries more potential to both detect and interpret effects.24
MG testing is scarce, especially for soil organisms, although some studies have been performed in recent years.20,25,26 For both E. crypticus and F. candida a comparable test design has been developed, with exposure to spiked soil for 4 consecutive generations followed by incubation in clean soil for 2 generations, which allows to further analyze potential transgenerational impacts of (chemical) stressors. A multigenerational exposure is particularly relevant when studying persistent materials as is also the case for many NMs. Hence, it was aimed to investigate the effects of prolonged multigenerational exposure to Ag NM in soil-dwelling organisms, using E. crypticus as a model species. Enchytraeids have a key role in soil ecosystems, they promote organic matter decomposition and aeration of the soil. In this study the effects of an MG exposure (5 generations exposed + 2 generations in clean soil) to Ag NM300K and AgNO3 were investigated, using the soil model organism E. crypticus. Survival and reproduction were assessed throughout, and Ag concentrations were measured, both in the animals and the soil, following exposure to sublethal Ag concentrations corresponding with the EC10 and EC50 for effects on reproduction in standard toxicity tests. The reference JRC Ag NMs27 were selected given the vast characterization and prior knowledge of their hazards to E. crypticus, covering various endpoints, namely the ones in the standard reproduction and the full life cycle toxicity tests (hatching, growth, maturity, survival, reproduction),14 in avoidance,28 gene expression,29 toxicokinetics30 and toxicodynamics studies.31
Materials and methods
Test organisms
Enchytraeus crypticus (Oligochaeta: Enchytraeidae) were maintained in cultures in agar media, consisting of Bacti–Agar medium (Oxoid, Agar No. 1) and a sterilized mixture of 4 different salt solutions at final concentrations of 0.08 mM KCl, 2 mM CaCl2·2H2O, 1 mM MgSO4, and 0.75 mM NaHCO3. Age-synchronized cultures were prepared as described in ref. 32. In short, adult organisms with well-developed clitellum were transferred to agar plates for cocoon laying, after which the cocoons (1–2 days old) were transferred to new agar and allowed to hatch. Juveniles of 19–21 days old after cocoon laying were used for the parental exposures in this study.
Test soil
The natural LUFA 2.2 standard soil (Speyer, Germany) was used. The main characteristics were (as provided by the supplier): pH (0.01 M CaCl2) = 5.6, organic carbon = 1.77%, cation exchange capacity = 8.5 cmolc per kg, maximum water holding capacity (WHCmax) = 43.3%, and grain size distribution of 10.6% clay (<0.002 mm), 15.0% silt (0.002–0.05 mm), and 74.4% sand (0.05–2.0 mm).
Test materials, characterisation and spiking procedures
Silver nitrate (AgNO3, purity >99%, Sigma-Aldrich) and the reference silver nanomaterial Ag NM300K, from the European Commission Joint Research Centre (JRC), were used. Ag NM300K is fully characterized, for full details see.27 Ag NM300K are spherical, consisting of a colloidal dispersion with a nominal silver content of 10.2% w/w, dispersed in 4% w/w of polyoxyethylene glycerol trioleate and polyoxyethylene sorbitan monolaurate (Tween 20). Approximately >99% of the particles have a nominal size of 15 nm. Transmission electron microscopy (TEM) shows a size of 17 ± 8 nm and smaller nanoparticles of ca. 5 nm were also present.
The tested concentrations were 0–45–60 mg Ag per kg soil DW for AgNO3 and 0–20–60 mg Ag per kg soil DW for Ag NM300K, selected within the sub-lethal range, based on the EC10 and EC50 for effects on reproduction in standard enchytraeid toxicity tests.14
Aqueous solutions of AgNO3 and Ag NM300K were prepared by diluting with water to the required concentrations. Spiking was performed by mixing the aqueous solutions with pre-moistened soil batches, which were then thoroughly mixed to obtain a homogeneous distribution and split into replicates. For the highest concentration of Ag NM300K (60 mg Ag per kg soil DW), spiking was done replicate per replicate. Soil moisture content was adjusted to 50% of the WHCmax. Soil was freshly spiked 1 day prior to the start of the exposure of each generation. Soil (3 g per treatment) was collected for Ag concentration quantification at the beginning of the exposure of each generation, dried at 40 °C for 48 hours and stored.
Experimental procedure
The standard OECD test guideline 220 (ref. 33) was followed with adaptations as described in ref. 20. In short, 40 juveniles (19–21 days old) were used per replicate to start the test. Test vessels were prepared containing 40 g of moist soil and food supply (ground oats: 36 ± 1 mg). Test duration was 32 days per generation and tests ran at 20 °C, with a 16
:
8 h photoperiod. The test design included a total of 7 generations with a total test duration of 224 days: 5 generations in spiked soil (F0–F4) plus 2 generations in clean soil (F5 and F6) to evaluate recovery. Six replicates were used for the control and the EC10, and 10 replicates for the EC50 exposure (the extra replicates were used to compensate for mortality and ensure enough animals would be available to start a next generation). Water and food were replenished weekly. At the end of each generation, organisms were collected directly from the soil and placed in ISO water34 for a short period of time to clean from soil particles. Juveniles (n = 40 per replicate) of medium size were collected and transferred to freshly spiked soil for the next generation exposure. Adults (n = 8, per treatment) were collected for Ag quantification, kept in ISO water34 for 12 hours to purge the gut from soil particles, then blotted dry on filter paper, stored individually and frozen at −20 °C. The remaining soil containing organisms was fixated with 96% ethanol and stained with Bengal rose (1% in ethanol) to count the total number of animals. The replicates were sieved through 3 decreasing pore size meshes (1.6, 0.5 and 0.3 mm) to help separating most of the soil from the organisms and facilitate counting. The reproduction and survival were assessed by counting the juveniles and adults with the help of a stereo microscope.
Ag measurement
Concentration of Ag was measured in animals and soil using graphite furnace atomic absorption spectrometry (AAS; PinAAcle 900Z, PerkinElmer, Singapore) and flame AAS (AAnalyst 100; PerkinElmer; Germany), respectively. Sample preparation was as follows: organisms were freeze-dried for 24 hours, weighted individually and digested with 300 μL of a mixture of HNO3 (Fischer Scientific OPTIMA Grade, Loughborough, UK) and HClO4 (70%; J.T.Baker (Avantor) Ultrex Ultra-Pure, Radnor, PA, USA) in a block heater (Techne Dri-Block Heater, Staffordshire, UK) using a heating ramp ranging from 85 to 180 °C. After all acid was evaporated, the left residue was dissolved in 300 (or 500) μL of 1 M HCl and Ag concentrations in the digests were measured by AAS. Limit of detection (LOD) for Ag was 0.003 mg kg−1 dry body weight, which is well within the experimental design requirements.
To determine total Ag concentrations in the test soil, approx. 130 mg dry sample from each replicate of each sampling day were digested using 2 ml of a destruction mixture of HNO3 (65%, Sigma-Aldrich) and HCl (37%, Sigma-Aldrich) (1
:
4 v/v), in Teflon containers that were closed tightly and heated at 140 °C for 7 hours. After cooling, 8 ml of deionized water was added, and the Ag concentrations measured by flame AAS. Certified reference material LGC 6181 was included in the analysis; mean (±SD; n = 2) silver concentrations measured in the reference material were 85.7 ± 0.69% of the certified values; all measured soil concentrations were corrected for this recovery. LOD for Ag analysis in soil samples was 0.003 mg Ag per kg dry soil, which is well within the experimental design requirements.
Data analysis
One-way analysis of variance (ANOVA) followed by Dunnett's comparison post-hoc test (p ≤ 0.05) was used to assess the significance of differences between F0 and the other generations within each treatment.35
Results
The soil pH (0.01 M CaCl2) did not significantly change during the test duration and with Ag treatment (Table S1†), being (average ± standard error) 6.0 ± 0.07 and 6.0 ± 0.05 for AgNO3 and 6.1 ± 0.02 and 6.1 ± 0.04 for Ag NM300K at the beginning and end of the tests, respectively.
Measured Ag concentrations in the spiked soils ranged between 73.0 and 113% of nominal ones (Table S2†), with average of 81.1 and 95.1% for AgNO3 at the EC10 and EC50 level, respectively. For Ag NM300K, Ag recovery ranged between 79.3 and 114%, with one outlier of 251% at the EC10 level in F2 (Table S2†). This resulted in average recoveries of 127 and 91.8% at the EC10 and EC50, respectively, with the high average recovery at the EC10 explained from the outlier at the F2.
The validity criteria for control performance of the test organisms, as defined by the OECD test guideline 220,33 were fulfilled. Control adult mortality was well below 20% (2–11% for AgNO3 and 4–7% for Ag NM300K) (Table S3†); juvenile numbers in the controls were always far above the minimum of 25 per 10 animals, ranging between 2700 and 5250 for the AgNO3 test and between 2473 and 4460 for the test with Ag NM300K (Table S3†). The coefficient of variation of juvenile numbers was always <20% (Table S3†), ranging between 6 and 18% for AgNO3 and between 9 and 20% for the NM300K exposures.
Enchytraeid survival (Fig. 1A) was not significantly affected by MG exposure, except for the decrease in the Ag NM300K exposure in the F1 at the EC50 level.
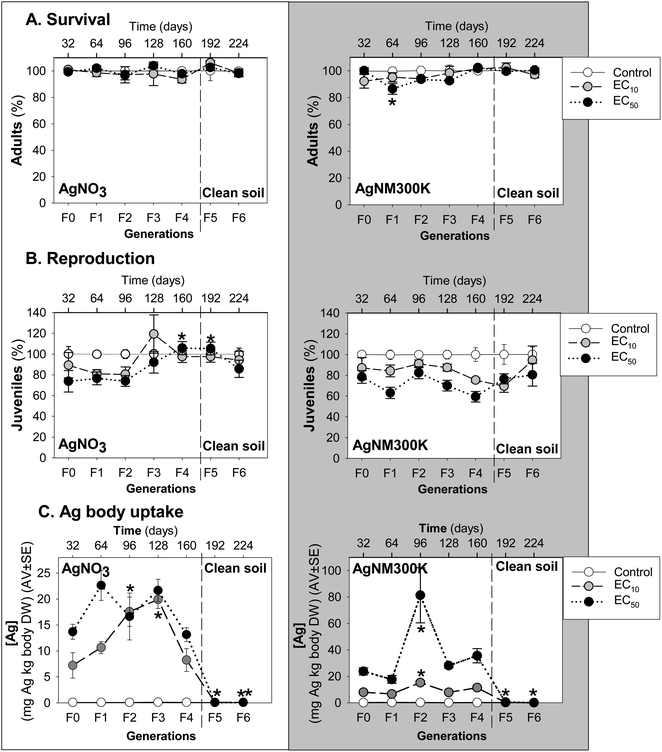 |
| Fig. 1 Results of the multigenerational test with Enchytraeus crypticus exposed for five generations (F0–F4) to AgNO3 (0–45–60 mg Ag per kg soil DW) and Ag NM300K (0–20–60 mg Ag per kg soil DW) in LUFA 2.2 natural soil followed by two generations in clean soil (F5-6). Measured endpoints are (A) survival, (B) reproduction, and (C) internal Ag concentrations in surviving animals. Results for survival and reproduction are expressed as % of the control. All values are presented as average ± standard error (AV ± SE), and exposure concentrations are indicated as effect levels (control, EC10 and EC50). *p < 0.05 (Dunnett's test for differences between parental generation (F0/F1) and the other generations). | |
MG exposure to AgNO3 caused a small dose-related decrease in enchytraeid reproduction up to F2, followed by an increase in F3, most pronounced at the EC10 level (higher than control) (Fig. 1B). This was followed by a decrease to juvenile numbers similar to the control in F4, a trend continuing in the last two generations in clean soils (F5-6).
For Ag NM300K MG exposure, a dose-related decrease occurred in reproduction up to F1, “interrupted” by an increase in F2, and followed by a decrease until F4 (Fig. 1B). After transfer to clean soil, increasing recovery was observed throughout F5 to F6.
The internal Ag concentrations measured in the surviving animals (Fig. 1C) of the exposure to AgNO3 showed that Ag uptake increased from F0 to F3, from 7 mg Ag per kg body DW (at the EC10) and 13 mg Ag per kg body DW (at the EC50) up to a maximum of 22 mg Ag per kg body DW (at both EC10 and EC50), after which it decreased in F4 to concentrations equivalent to F0. After transferring to clean soil (F5 and F6) the Ag concentration in the animals was negligible.
For Ag NM300K, a dose-related increase of Ag body concentrations was seen in F0 and F1 (8 and 24 mg Ag per kg body DW for EC10 and EC50, respectively) followed by a peak in F2, reaching a maximum of 80 mg Ag per kg body DW in the exposure to 60 mg Ag NM300K per kg soil DW (EC50). At the EC10 level, maximum body concentration in the F2 was about 20 mg Ag per kg body DW. This peak in the F2 was followed by a decrease of Ag body concentrations in F3 and F4 to values similar to F0–F1. After transfer to clean soil, Ag concentration in the animals was close to zero.
Discussion
Exposure to sublethal reproduction EC10 and EC50 of AgNO3 and Ag NM300K did not affect long-term survival of E. crypticus in LUFA 2.2 soil. Similar MG studies with CoCl2 and WCCo NM26 and CuCl2 and CuO NM20 showed no major variations in the survival of E. crypticus also exposed to concentrations corresponding with the EC10 and EC50 for effects on reproduction in standard toxicity tests. This is not surprising since the exposure concentrations were sublethal but there could be an unknown accumulation of effects via MG exposure, as observed e.g. in F. candida exposed for multiple generations to phenanthrene.36 The reproduction response at F0/F1 was as expected based on the ECx determined previously in standard toxicity tests.14
A large variation was observed for the internal Ag concentrations in the enchytraeids, in particular for Ag NM300K in the F3. Such large variation has been reported previously, e.g., by.26,37–40 Variability is naturally expected due to individual genetic variation, sexual reproduction, age, patchy soil exposure, to name some of the factors. Body Ag concentrations in the enchytraeids did not clearly explain toxicity, as no clear relationships were found between juvenile numbers produced in the different generations and body Ag concentrations in the adults. Nevertheless, the reproduction in the AgNO3 MG test at the EC10 level showed an increase in F3, which coincided with a higher Ag uptake while the reduction in juvenile numbers in the following generations also went along with a decrease in Ag body concentrations. For AgNM300K a similar pattern was seen but one generation earlier, in the F2, and with a less wide increase in reproduction with Ag body concentrations, especially for the EC50, after a peak in internal Ag concentration measured in F2 (80 mg Ag per kg body DW). Also in this case, the decrease in Ag body concentrations in the F3 and F4 coincided with a decrease in reproduction. This may perhaps indicate a trade-off in energy allocation between detoxification and reproduction, i.e., more energy was spent on detoxification/elimination of Ag and hence there was less energy to reproduce. The MG effect of Ag NM300K was different from that of CuO NM20 which increased throughout the generations, while for Ag NM300K the initial increase was followed by a decrease in effect. For both Ag forms, transfer to clean soil allowed animals to recover reproduction to the control level.
Moreover, results of this MG study were in agreement with those of toxicokinetic studies, where a maximum uptake of 20 mg Ag per kg body DW and 70 mg Ag per kg body DW was observed after 14 days exposure to 45 mg AgNO3 per kg soil DW and 60 mg AgNM300K per kg soil DW, respectively.38 This might suggest that after reaching an internal Ag threshold, detoxification mechanisms were activated and Ag was more efficiently eliminated, co-occurring with a reduction in reproduction. A similar pattern was observed for exposure to CoCl2 where at F4 reproduction peaked for E. crypticus exposed to the EC10 and EC50, also corresponding with an increase in the uptake of Co, i.e., internal Co concentration at F3.26 This trend is confirmed by a study where the exposure to CoCl2 was extended up to 56 days (equivalent to an additional generation or F2), which showed an increase in the impact at the population level,41 also after a peak in the internal Co concentration in the F1 (28 days exposure). For E. crypticus, MG exposure to CuCl2 at the EC10 and EC50 (ref. 41) also showed an increased reproduction from F2 to F5, although in this case we do not know if there was a relation with internal Cu concentration.
A similar MG exposure design with F. candida showed lower impacts, with no effects for CuO NM up to 6400 mg Cu per kg soil DW or for WCCo NM where effects on reproduction and survival only occurred from the F3 onwards, with EC50 values between 2400 and 5600 mg WCCo NM per kg soil DW.25 Nevertheless, expression of target genes was affected in all exposures and generations, and not always returned to control levels after transfer to clean soil. This indicates the different species sensitivity but also that the mechanisms were triggered even though without apparent phenotypic toxicity.
Conclusions
The MG exposure of E. crypticus to AgNO3 and Ag NM300K showed that Ag uptake was dose-related and increased throughout generations, peaking in the F1 (64 days) for AgNO3 and F2 (96 days) for Ag NM300K, after which it decreased. In line with earlier toxicokinetic studies, a maximum body Ag concentration was reached (20 mg Ag (AgNO3) per kg body DW and 70 mg Ag (AgNM300K) per kg body DW), after which detoxification mechanisms seemed to be activated leading to elimination of Ag and co-occurring with a decreased reproduction. Transfer to clean soil allowed Ag concentrations in the animals to return to background levels. This MG study confirms the effects at the EC10 and EC50 levels of exposure to AgNO3 and Ag NM300K determined in standard reproduction toxicity tests. MG results allowed to monitor the dynamics between exposure and effects of Ag materials, and how the animals seem to cope with exposure throughout 7 generations by compensating between detoxification and reproductive output.
Author contributions
FCFS: formal analysis, investigation, methodology, writing – original draft, RV: investigation, AMVM: conceptualization, CvG, JSF, MJBA: conceptualization, data curation, formal analysis, funding acquisition, resources, supervision, writing – original draft. All authors: write – review & editing.
Conflicts of interest
There are no conflicts of interest to declare.
Acknowledgements
This study was supported by the European Commission Projects BIORIMA (H2020-NMBP-2017, GA No. 760928), NanoInformaTIX (H2020-NMBP-14-2018, No. 814426) and NANORIGO (H2020-NMBP-13-2018, GA No. 814530). Further support by FCT/MCTES through national funds (PIDDAC), and the co-funding by the FEDER, within the PT2020 Partnership Agreement and Compete 2020 via CESAM (UIDB/50017/2020+UIDP/50017/2020+LA/P/0094/2020) and BEAUTY: Big gEnome wide Applications for an ecotoxicology soil model – a knowledge base to Unravel mechanisms (nanopesTY_cides)” (PTDC/CTA-AMB/3970/2020). Thanks are due to FCT via a PhD grant to FCFS (SFRH/BD/118294/2016) and an exceptional PhD grant to FCFS (COVID/BD/151720/2021). Sekerani B. Chidiamassamba is acknowledged by the help in methodological procedures and sampling in some generations.
References
- T. Y. Sun, N. A. Bornhöft, K. Hungerbühler and B. Nowack, Environ. Sci. Technol., 2016, 50, 4701–4711 CrossRef CAS PubMed.
- M. Hauser and B. Nowack, Environ. Int., 2021, 146, 106184 CrossRef CAS PubMed.
- R. Behra, L. Sigg, M. J. D. Clift, F. Herzog, M. Minghetti, B. Johnston, A. Petri-Fink and B. Rothen-Rutishauser, J. R. Soc., Interface, 2013, 10, 20130396 CrossRef.
- J. Fabrega, S. N. Luoma, C. R. Tyler, T. S. Galloway and J. R. Lead, Environ. Int., 2011, 37, 517–531 CrossRef CAS PubMed.
- N. Hadrup and H. R. Lam, Regul. Toxicol. Pharmacol., 2014, 68, 1–7 CrossRef CAS PubMed.
- N. R. Panyala, E. M. Peña-Méndez and J. Havel, J. Appl. Biomed., 2008, 6, 117–129 CrossRef CAS.
- F. Ribeiro, C. A. M. Van Gestel, M. D. Pavlaki, S. Azevedo, A. M. V. M. Soares and S. Loureiro, Sci. Total Environ., 2017, 574, 1633–1639 CrossRef CAS.
- A. E. P. Del Real, H. Castillo-Michel, R. Kaegi, B. Sinnet, V. Magnin, N. Findling, J. Villanova, M. Carriére, C. Santaella, A. Fernández-Martínez, C. Levard and G. Sarret, Environ. Sci. Technol., 2016, 50, 1759–1768 CrossRef PubMed.
- M. E. Vance, T. Kuiken, E. P. Vejerano, S. P. McGinnis, M. F. Hochella, D. Rejeski and M. S. Hull, Beilstein J. Nanotechnol., 2015, 6, 1769–1780 CrossRef CAS PubMed.
- Y. Chen, Y. Si, D. Zhou and F. Dang, Environ. Pollut., 2017, 222, 50–57 CrossRef CAS PubMed.
- R. A. González-Fuenzalida, L. Sanjuan-Navarro, Y. Moliner-Martínez and P. Campíns-Falcó, Sci. Total Environ., 2018, 630, 1226–1236 CrossRef PubMed.
- S. Makama, J. Piella, A. Undas, W. J. Dimmers, R. Peters, V. F. Puntes and N. W. van den Brink, Environ. Pollut., 2016, 218, 870–878 CrossRef CAS.
- A. R. Whitley, C. Levard, E. Oostveen, P. M. Bertsch, C. J. Matocha, F. von der Kammer and J. M. Unrine, Environ. Pollut., 2013, 182, 141–149 CrossRef CAS.
- R. C. Bicho, T. Ribeiro, N. P. Rodrigues, J. J. Scott-Fordsmand and M. J. B. B. Amorim, J. Hazard. Mater., 2016, 318, 608–614 CrossRef CAS.
- M. J. C. van der Ploeg, R. D. Handy, P. L. Waalewijn-Kool, J. H. J. van den Berg, Z. E. Herrera Rivera, J. Bovenschen, B. Molleman, J. M. Baveco, P. Tromp, R. J. B. Peters, G. F. Koopmans, I. M. C. M. Rietjens and N. W. Van den Brink, Environ. Toxicol. Chem., 2014, 33, 743–752 CrossRef CAS.
- S. I. L. L. Gomes, D. Hansen, J. J. Scott-Fordsmand and M. J. B. B. Amorim, Environ. Pollut., 2015, 199, 49–55 CrossRef CAS PubMed.
- A. H. Jesmer, J. R. Velicogna, D. M. Schwertfeger, R. P. Scroggins and J. I. Princz, Environ. Toxicol. Chem., 2017, 36, 2756–2765 CrossRef CAS PubMed.
- P. S. Tourinho, C. A. M. van Gestel, A. J. Morgan, P. Kille, C. Svendsen, K. Jurkschat, J. F. W. Mosselmans, A. M. V. M. Soares and S. Loureiro, Ecotoxicology, 2016, 25, 267–278 CrossRef CAS PubMed.
- M. Amorim, Int. J. Environ. Res. Public Health, 2016, 13, 245 CrossRef PubMed.
- R. Bicho, F. Santos, J. Scott-Fordsmand and M. Amorim, Sci. Rep., 2017, 7, 1–7 CrossRef CAS.
- C. L. C. L. Schultz, A. Wamucho, O. V. O. V. Tsyusko, J. M. J. M. Unrine, A. Crossley, C. Svendsen and D. J. D. J. Spurgeon, Proc. R. Soc. B, 2016, 283, 20152911 CrossRef PubMed.
- M. Broerse and C. A. M. van Gestel, Chemosphere, 2010, 79, 953–957 CrossRef CAS.
- B. Guimarães, J. Römbke and M. J. B. Amorim, Sci. Total Environ., 2023, 854, 158680 CrossRef.
- M. J. B. Amorim, M. L. Fernández-Cruz, K. Hund-Rinke and J. J. Scott-Fordsmand, Environ. Sci. Eur., 2020, 32, 1–13 CrossRef.
- J. W. Noordhoek, R. A. Verweij, C. A. M. van Gestel, N. M. van Straalen and D. Roelofs, Environ. Sci.: Nano, 2018, 5, 564–571 RSC.
- M. J. Ribeiro, J. J. Scott-Fordsmand and M. J. B. Amorim, Nanotoxicology, 2019, 13, 751–760 CrossRef CAS PubMed.
-
C. L. Klein, S. Comero, B. Stahlmecke, J. Romazanov, T. A. J. Kuhlbusch, E. Van Doren, P.-J. D. T. J. Mast, P. Wick, H. Krug, G. Locoro, K. Hund-Rinke, W. Kördel, S. Friedrichs, G. Maier, J. Werner, T. Linsinger and B. M. Gawlik, NM-Series of Representative Manufactured Nanomaterials, NM-300 Silver Characterisation, Stability, Homogeneity, Publications Office of the European Union, Luxembourg, 2011 Search PubMed.
- N. P. Rodrigues, J. J. Scott-Fordsmand and M. J. B. Amorim, Environ. Pollut., 2020, 262, 114277 CrossRef CAS PubMed.
- S. I. L. Gomes, C. P. P. Roca, J. J. Scott-Fordsmand and M. J. B. Amorim, Environ. Sci.: Nano, 2017, 4, 929–937 RSC.
- F. C. F. Santos, P. S. Tourinho, J. J. Scott-Fordsmand, C. A. M. van Gestel and M. J. B. Amorim, Environ. Sci.: Nano, 2021, 8, 2629–2640 RSC.
- F. C. F. Santos, R. A. Verweij, C. A. M. van Gestel and M. J. B. Amorim, Ecotoxicol. Environ. Saf., 2023, 252, 114599 CrossRef CAS PubMed.
- R. Bicho, F. Santos, M. Goncalves, A. Soares and M. Amorim, Ecotoxicology, 2015, 24, 1053–1063 CrossRef CAS PubMed.
-
OECD, Guidelines for the Testing of Chemicals No. 220. Enchytraeid Reproduction Test, Organization for Economic Cooperation and Development, Paris, France, 2016 Search PubMed.
-
OECD, Test No. 202: OECD Guidelines for Testing of Chemicals — Daphnia sp., Acute Immobilisation Test, OECD (Organization for Economic Cooperation and Development). OECD Publishing, Paris, Paris, France, 2004 Search PubMed.
-
SPSS, Sigma Plot for Windows Version 12.5, Copyright 2011 Systat Software, Inc., 2011 Search PubMed.
- M. L. L. Paumen, E. Steenbergen, M. H. S. Kraak, N. M. van Straalen and C. A. M. van Gestel, Environ. Sci. Technol., 2008, 42, 6985–6990 CrossRef PubMed.
- M. J. Ribeiro, M. J. B. Amorim and J. J. Scott-Fordsmand, Nanomaterials, 2019, 9, 1087 CrossRef CAS PubMed.
- F. C. F. Santos, P. S. Tourinho, J. J. Scott-Fordsmand, C. A. M. van Gestel and M. J. B. Amorim, Environ. Sci.: Nano, 2021, 8, 2629–2640 RSC.
- F. C. F. Santos, R. A. Verweij, C. A. M. van Gestel and M. J. B. Amorim, Ecotoxicol. Environ. Saf., 2023, 252, 114599 CrossRef CAS.
- F. C. F. Santos, C. A. M. Van Gestel and M. J. B. Amorim, Chemosphere, 2021, 270, 129433 CrossRef CAS.
- M. J. Ribeiro, V. L. Maria, A. M. V. M. Soares, J. J. Scott-Fordsmand and M. J. B. Amorim, Environ. Sci. Technol., 2018, 52, 11394–11401 CrossRef CAS.
|
This journal is © The Royal Society of Chemistry 2024 |
Click here to see how this site uses Cookies. View our privacy policy here.